Ever wondered how the cells in your body get the nutrients they need to function? It’s not like they have a mini grocery store to pop into whenever they run low on sugars or amino acids, right? Welcome to the fascinating world of Active Transport.
“Active Transport – the unsung hero of cellular operations, ensuring that our cells never run out of stock.”
Just like a diligent stock replenisher in a supermarket, active transport is constantly at work, moving molecules from a region of lower concentration to a region of higher concentration. But how does it happen? Buckle up, as we journey into the heart of our cells to explore this vital process.
Transport System in Membrane
The transport system in cellular membranes plays a crucial role in maintaining the proper functioning of biological organisms. The semi-permeable nature of the cellular membrane allows for selective movement of ions, molecules, and water across the membrane. This permeability is determined by the structural arrangement of the lipid bilayer, which interacts with various macromolecules such as proteins, carbohydrates, and lipids to facilitate molecular transport. Controlling the transportation of molecules across the membrane is vital for the survival and well-being of organisms.
There are two main modes of transport employed by cellular membranes:
- Passive Transport: This mechanism enables the movement of substances across the membrane without requiring any expenditure of energy. It occurs down the concentration gradient, meaning molecules move from areas of high concentration to areas of low concentration. Some examples of passive transport include:
- The diffusion of neurotransmitters like acetylcholine across synaptical junctions in neurons.
- The diffusion of gases in the lungs across alveolar membranes.
- The movement of water molecules through osmosis.
- Active Transport: In contrast to passive transport, active transport involves the movement of molecules against the concentration gradient, from areas of low concentration to areas of high concentration. This process requires energy derived from adenosine triphosphate (ATP). Examples of active transport include:
- The movement of Ca2+ ions out of cardiac muscle cells.
- The transportation of glucose molecules across membranes.
- The movement of amino acids across the intestinal lining in the human gut.
Any disruptions or mutations in the transport system or the membrane transporters involved can lead to significant health conditions. Disorders such as depression, cystic fibrosis, drug abuse, migraine, and epilepsy can be attributed to abnormalities in the transportation system within cellular membranes. Understanding the intricacies of membrane transport is crucial for identifying potential therapeutic targets and developing treatments for these conditions.
In summary, the transport system in cellular membranes is responsible for the selective movement of molecules across the membrane. Passive transport occurs down the concentration gradient without energy expenditure, while active transport involves the movement of molecules against the concentration gradient with the help of ATP. Maintaining the integrity and functionality of this transport system is vital for the overall health and well-being of biological organisms.
Lipid Bilayers and Membrane Proteins
- Lipid bilayers and membrane proteins are integral components of biological membranes, playing essential roles in maintaining the integrity and functionality of cells. The phospholipid bilayer, a fundamental structural element of most biological membranes, consists of two layers of phospholipids with hydrophilic heads facing outward and hydrophobic tails facing inward. Embedded within this lipid bilayer are transmembrane proteins that span the entire thickness of the membrane.
- The integrated proteins within the membrane serve as transport channels, pumps, and carriers, facilitating the translocation of ions and macromolecules across the membrane. Channel proteins, for instance, create pores or channels in the membrane that enable the unrestricted passage of inorganic ions such as Na+, Cl-, K+, and H+, as well as molecules of appropriate size. These channel proteins are tightly regulated by extracellular signals, allowing for precise control of ion and solute movement across the membrane.
- Carrier proteins, on the other hand, function like enzymes, selectively binding to specific small molecules such as glucose and transporting them across the membrane. They undergo conformational changes to facilitate the translocation of these molecules from one side of the membrane to the other. This selective binding and transport mechanism ensures the efficient movement of necessary molecules while maintaining proper cellular homeostasis.
- The composition of the lipid-to-protein ratio within the membrane can vary depending on the specific cell type. For example, the mitochondrial inner membrane possesses a high protein content, with approximately 75% of its composition being proteins. This suggests the presence of protein complexes that are vital for oxidative phosphorylation and the electron transport system, crucial processes in cellular respiration.
- Understanding the interplay between lipid bilayers and membrane proteins is crucial for comprehending the functional dynamics of biological membranes. The lipid bilayer provides a structural framework and acts as a barrier, while membrane proteins enable selective transport and communication between the cell and its environment. This intricate relationship between lipids and proteins contributes to the overall integrity and functionality of cellular membranes, allowing cells to perform their diverse biological functions.
What is Active Transport?
Active transport refers to the energy-driven movement of ions, small molecules, and solutes across the biological membrane, against an electrochemical or concentration gradient. Unlike passive transport, which occurs spontaneously without the need for energy, active transport requires the expenditure of cellular energy to maintain the homeostasis of molecules and ions within the cell. It plays a crucial role in regulating the internal environment of cells.
There are two types of active transport: primary and secondary active transport.
- Primary active transport: In primary active transport, energy derived from ATP (adenosine triphosphate) is directly used to transport molecules against their concentration gradient. ATP provides the necessary energy to fuel carrier proteins or pumps embedded in the cell membrane. These pumps utilize ATP to actively move molecules or ions from an area of lower concentration to an area of higher concentration. An example of primary active transport is the sodium-potassium pump, which maintains the concentration gradients of sodium and potassium ions across the cell membrane.
- Secondary active transport: Secondary active transport refers to the transport of molecules across the membrane using the energy stored in the electrochemical gradient established by primary active transport. This transport mechanism relies on the coupling of two molecules or ions. The movement of one molecule or ion down its concentration gradient provides the energy necessary to transport another molecule or ion against its concentration gradient. An example of secondary active transport is the uptake of glucose in the intestine. Sodium ions are actively pumped out of the cell, creating a sodium concentration gradient. The energy stored in this gradient is then used to transport glucose into the cell against its concentration gradient.
Active transport is crucial for the proper functioning of cells, as it enables the uptake of essential molecules and ions against their natural tendency to diffuse from areas of higher concentration to lower concentration. It allows cells to maintain specific internal concentrations required for various cellular processes. Examples of active transport include the uptake of glucose in the intestine and the uptake of minerals or ions into plant root hair cells.
In summary, active transport is a cellular process that utilizes energy to move molecules and ions against their concentration gradient across the cell membrane. It plays a vital role in maintaining cellular homeostasis and facilitating the transport of essential substances into and out of cells.
Definition of Active Transport
Active transport is the energy-driven movement of molecules or ions across a membrane against their concentration gradient, requiring the expenditure of cellular energy, usually in the form of ATP (adenosine triphosphate).
Process of Active Transport
- The process of active transport involves the movement of substances across the cell membrane from an area of low concentration to an area of high concentration, contrary to the process of osmosis. Active transport relies on the expenditure of energy and is typically facilitated by specific transport proteins that undergo shape changes when interacting with adenosine triphosphate (ATP), the cell’s “fuel” molecule.
- In primary active transport, a transport protein within the cell membrane binds to the substance it intends to transport, such as a sodium ion. The protein holds onto the substance until ATP molecules bind to it. The energy stored in ATP allows the transport protein to change its shape, releasing the substance on the opposite side of the cell membrane. This direct use of ATP for active transport is known as primary active transport.
- Secondary active transport is another form of active transport that does not directly use ATP. Instead, the cell must utilize ATP to maintain the functioning of the transport protein. In secondary active transport, the transport protein uses the energy stored in the electrochemical gradient established by primary active transport to transport other substances. This process will be further explained in the section on Symport Pumps.
- Apart from primary and secondary active transport, active transport can also occur through processes called endocytosis and exocytosis. In exocytosis, the cell expels large quantities of substances outside of itself by enclosing them in a membrane-bound vesicle and releasing the vesicle from the cell. On the other hand, in endocytosis, the cell internalizes substances by enveloping them with its membrane, forming a vesicle.
- Each type of active transport has its own specific mechanisms and functions. Primary active transport directly uses ATP to transport substances, while secondary active transport utilizes the energy stored in electrochemical gradients. Additionally, endocytosis and exocytosis allow for the movement of larger quantities of substances into and out of cells, respectively.
- In summary, active transport involves the movement of substances against their concentration gradient, requiring energy expenditure. It can occur through primary active transport, secondary active transport, as well as through endocytosis and exocytosis processes. These mechanisms play vital roles in maintaining cellular homeostasis and facilitating the transportation of essential molecules and substances.
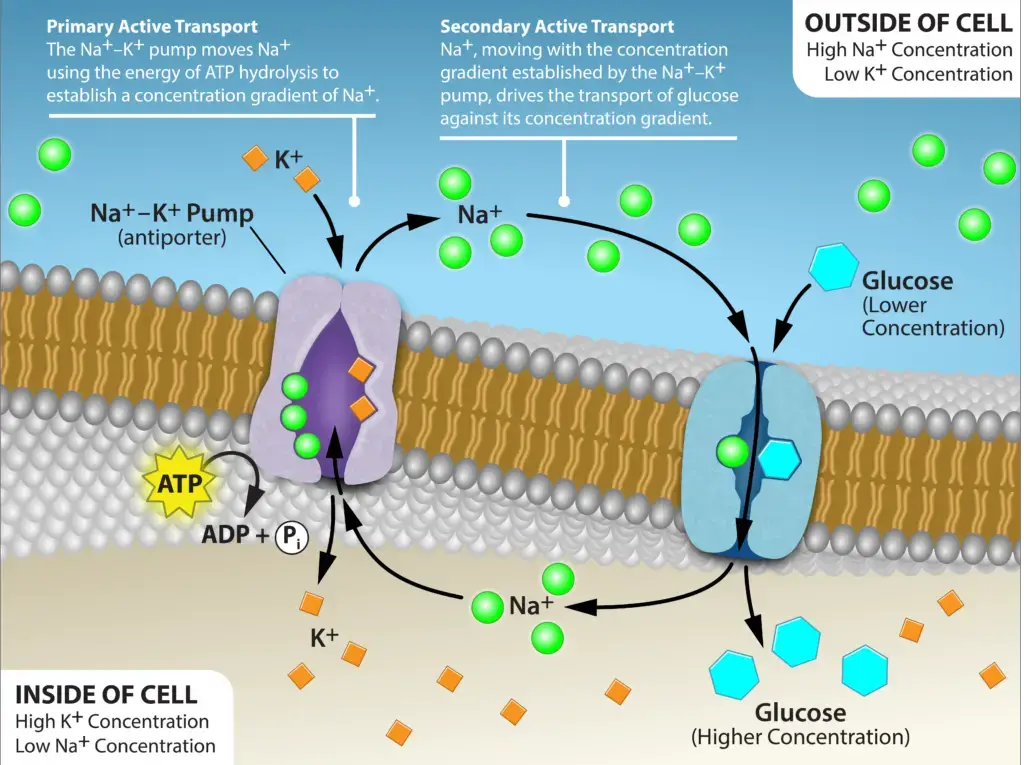
Electrochemical Gradient
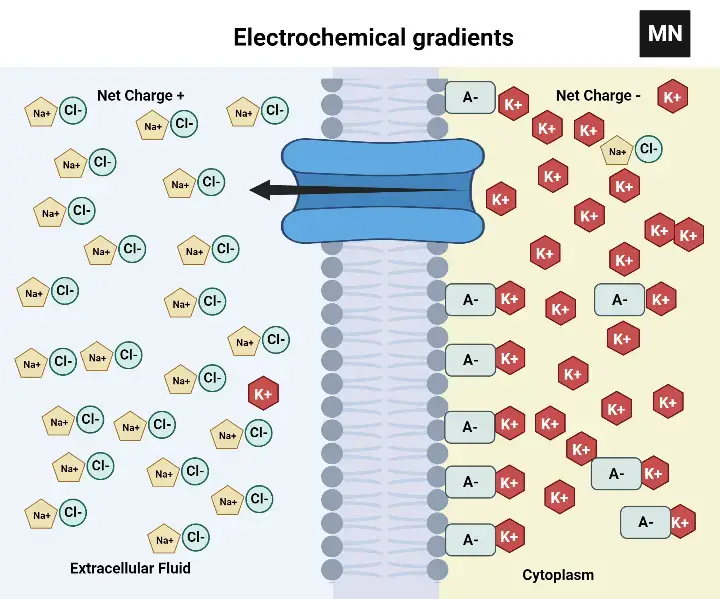
- In addition to simple concentration gradients, living systems exhibit a more intricate phenomenon known as an electrochemical gradient. This gradient arises due to the movement of ions into and out of cells, as well as the presence of proteins that do not cross the cell membrane and carry a negative charge. Consequently, there exists a difference in charge across the plasma membrane, resulting in an electrical gradient.
- Inside living cells, the interior is electrically negative in comparison to the positively charged extracellular fluid. Furthermore, cells have higher concentrations of potassium ions (K+) and lower concentrations of sodium ions (Na+) than the extracellular fluid. As a result, the concentration gradient of Na+ tends to drive it into the cell, while its positive charge also promotes inward movement towards the negatively charged interior.
- However, the situation is more intricate for other ions such as potassium. The positive charge of potassium also drives it into the cell, but the concentration gradient of potassium drives it out of the cell. Thus, there is a simultaneous influence of both the concentration gradient and the electrical charge on ions such as potassium. The combined effect of these factors on an ion is referred to as its electrochemical gradient.
- In summary, the electrochemical gradient encompasses both the concentration gradient and the electrical charge that affect the movement of ions. This phenomenon plays a crucial role in the regulation of ion transport across cell membranes and is a fundamental aspect of cellular function.
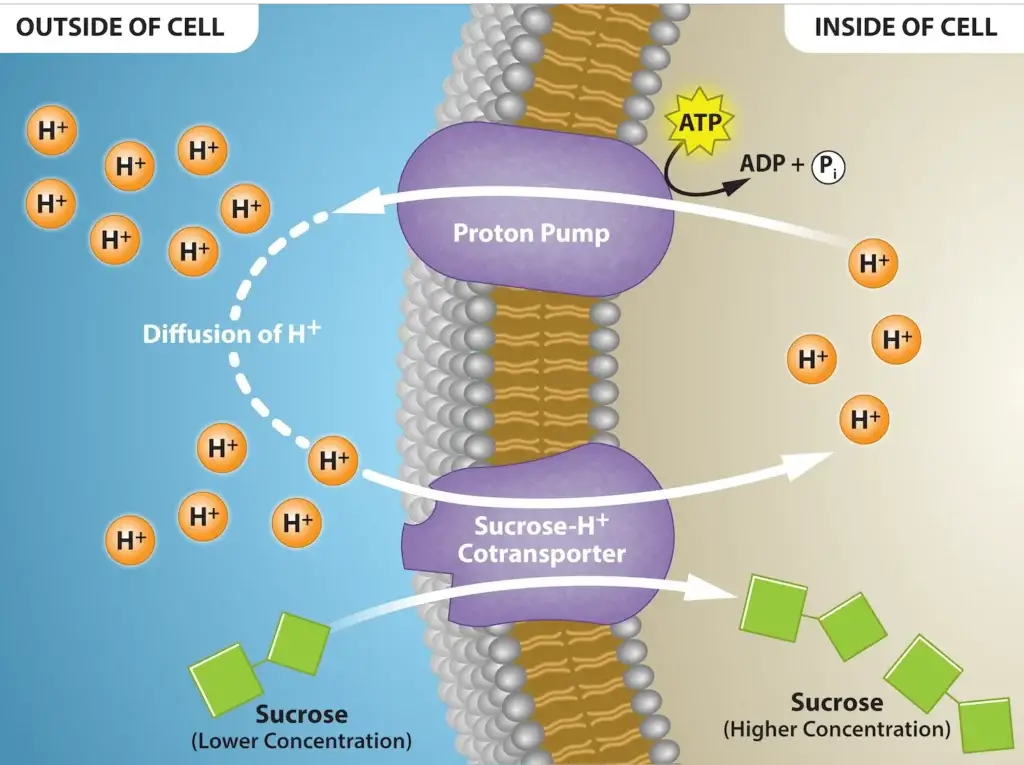
Moving Against a Gradient
In order to transport substances against a concentration or electrochemical gradient, cells need to expend energy. This energy is derived from adenosine triphosphate (ATP) generated through the cell’s metabolic processes. Active transport mechanisms, often referred to as pumps, work against electrochemical gradients to move substances.
Plasma membranes allow small substances to constantly pass through. However, active transport is necessary to maintain the concentrations of ions and other substances that living cells require, counteracting the passive movements. Cells dedicate a significant portion of their metabolic energy supply to sustain these active transport processes. For instance, a red blood cell utilizes a substantial amount of its metabolic energy to maintain the imbalance between the levels of sodium and potassium in its exterior and interior environments.
Active transport mechanisms rely on the cell’s metabolism to produce the required energy, making them vulnerable to metabolic poisons that disrupt the supply of ATP.
There are two primary mechanisms for transporting small-molecular weight material and small molecules against a gradient: primary active transport and secondary active transport.
- Primary Active Transport: Primary active transport involves the movement of ions across a membrane, creating a charge difference across that membrane. This process directly depends on the availability of ATP to drive the transport of substances against their concentration or electrochemical gradient.
- Secondary Active Transport: Secondary active transport does not directly require ATP. Instead, it relies on the establishment of an electrochemical gradient by primary active transport. The movement of material occurs due to the energy stored in this established gradient.
In summary, moving substances against a concentration or electrochemical gradient necessitates the utilization of energy by the cell. Active transport mechanisms, driven by ATP, allow cells to maintain the required concentrations of ions and other substances despite the passive movements. Primary active transport directly uses ATP, while secondary active transport utilizes the energy stored in an electrochemical gradient established by primary active transport.
Carrier Proteins for Active Transport
One of the critical adaptations of cell membranes for active transport is the presence of specific carrier proteins or pumps that facilitate the movement of substances. There are three types of proteins or transporters involved in active transport: uniporters, symporters, and antiporters.
- Uniporters: Uniporters are carrier proteins that transport a single specific ion or molecule across the membrane. They facilitate the movement of a particular substance in one direction.
- Symporters: Symporters are carrier proteins that transport two different ions or molecules simultaneously, and they transport these substances in the same direction across the membrane. These proteins work by coupling the movement of one molecule or ion with the movement of another molecule or ion.
- Antiporters: Antiporters are carrier proteins that transport two different ions or molecules across the membrane, but in opposite directions. They simultaneously move one substance into the cell while moving another substance out of the cell.
All three types of carrier proteins can also transport small, uncharged organic molecules like glucose. It is important to note that these carrier proteins are also involved in facilitated diffusion, but in that process, they do not require ATP to function.
Some examples of pumps used in active transport include the Na+-K+ ATPase and H+-K+ ATPase. The Na+-K+ ATPase is an antiporter that transports sodium ions out of the cell while moving potassium ions into the cell. The H+-K+ ATPase is another antiporter that transports hydrogen ions out of the cell while moving potassium ions into the cell.
Additionally, there are other carrier proteins involved in active transport, such as the Ca2+ ATPase, which specifically transports calcium ions, and the H+ ATPase, which specifically transports hydrogen ions. Both of these proteins act as pumps, transporting their respective ions across the membrane.
In summary, carrier proteins play a crucial role in active transport by facilitating the movement of specific ions or molecules across the cell membrane. Uniporters, symporters, and antiporters are involved in this process, and they can also transport small, uncharged organic molecules. Examples of pumps used in active transport include the Na+-K+ ATPase, H+-K+ ATPase, Ca2+ ATPase, and H+ ATPase. These carrier proteins contribute to the maintenance of concentration gradients and the regulation of cellular processes.
Types of Active Transport
Active conveyance is divided into two categories based on how energy is coupled to the mechanisms that provide propulsion. Primary and secondary active transport are the courses.
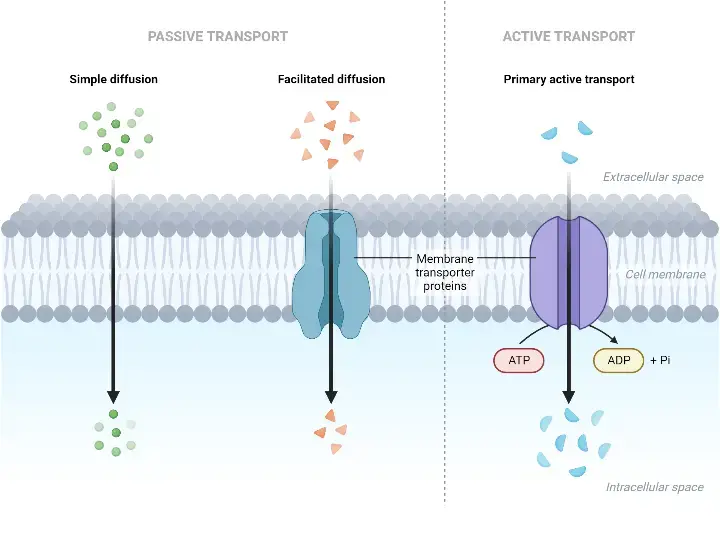
A. Primary Active Transport
- Primary active transport, also known as direct active or uniport transport, is a form of active transport that directly utilizes metabolic energy to transport substances across the cell membrane. This mode of transport is often associated with the breakdown of adenosine triphosphate (ATP) to adenosine diphosphate (ADP) and inorganic phosphate.
- Primary active transport is primarily involved in the movement of metal ions such as K+, Na+, Cl-, H+, Mg2+, and Ca2+. The transportation of these ions requires the activity of specific enzymes called ATPases. These ATPases function as ion pumps or channels, facilitating the movement of charged ions across the membrane.
- Through primary active transport, ions are transported against their concentration gradient, meaning they are moved from an area of lower concentration to an area of higher concentration. This process requires the direct expenditure of energy derived from ATP hydrolysis.
- The movement of ions via primary active transport plays a crucial role in various physiological processes. For example, the sodium-potassium pump is a well-known example of primary active transport, which actively transports sodium ions out of the cell and potassium ions into the cell. This process is vital for maintaining the proper electrochemical balance and membrane potential of cells.
- In summary, primary active transport is a direct mode of active transport that utilizes energy from ATP hydrolysis to transport molecules, particularly metal ions, across the cell membrane. The enzymes involved, known as ATPases, facilitate the movement of charged ions through ion channels or pumps. This transport mechanism is essential for maintaining ion gradients and ensuring proper cellular function.
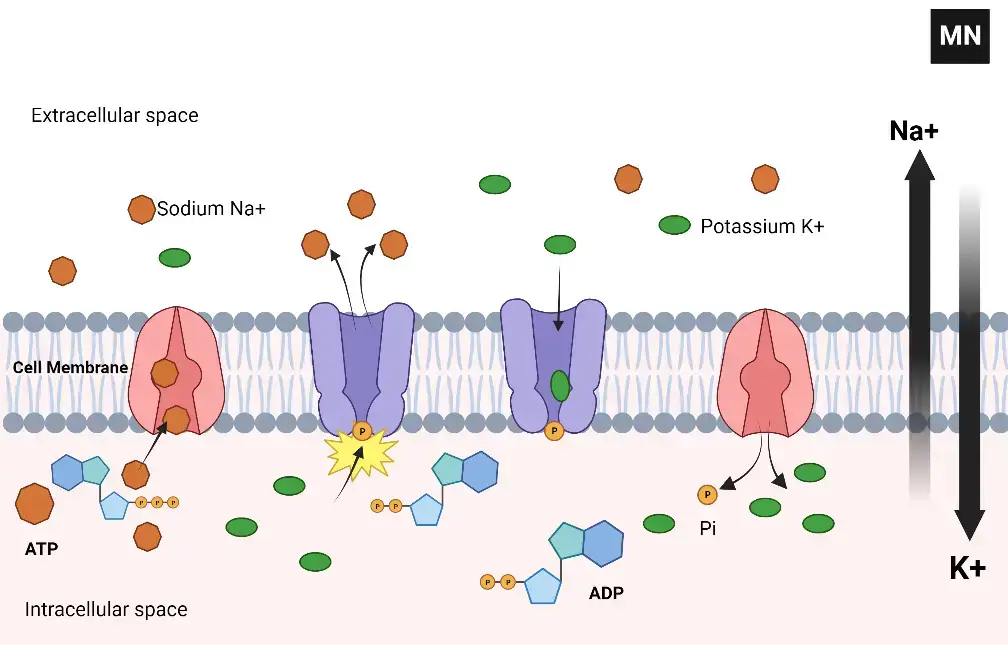
Types of Primary Transport
1. P-type ATPase
- P-type ATPase, also known as E1-E2 ATPase, is a class of ion and lipid pumps found in bacteria, archaea, and eukaryotes. The term “P-type” refers to their ability to autophosphorylate specific components within the pump, such as aspartate and ATP.
- These ATPases can interchange between two conformations, labeled as E1 and E2. Structurally, they consist of four functional domains: the Phosphorylation (P) domain, Nucleotide-binding (N) domain, Actuator (A) domain, and Regulatory (R) domain.
- Various P-type ATPases have been identified, including the sodium-potassium pump (Na+/K+-ATPase), calcium pump (Ca2+-ATPase), proton-potassium pump (H+/K+-ATPase), and proton pump (H+-ATPase) found in plants and fungi.
- In humans, P-type ATPases play diverse roles in processes such as nerve impulses, absorption of nutrients and solutes in the intestine and kidney, and muscle relaxation.
- These ATPases are involved in active transport processes and are particularly associated with ion channels. They utilize ATP to transport ions across the cell membrane, maintaining the ion gradients necessary for various cellular functions. The autophosphorylation of components, including ATP and aspartate, within the P-type ATPase structure is a key characteristic of these pumps.
- The functional domains within P-type ATPase enable their transport functions. The P-domain, or Phosphorylation domain, contains amino acids like aspartate involved in autophosphorylation. The N-domain, or Nucleotide-binding domain, is responsible for binding to ATP.
- The A-domain, or Actuator domain, includes a phosphatase domain that participates in the dephosphorylation of the phosphorylated component. The R-domain, or Regulatory domain, regulates the activity of the ATPase.
- Overall, P-type ATPases are important players in active ion transport processes in eukaryotes and bacteria. Their involvement in various cellular functions highlights their significance in maintaining ion homeostasis and proper physiological processes.
Sodium-Potassium (Na+/K+ ATPase) Pump Mechanism
The sodium-potassium (Na+/K+) ATPase pump is an essential membrane protein involved in maintaining the electrochemical balance and proper functioning of cells. Here is an overview of the mechanism of the Na+/K+ ATPase pump:
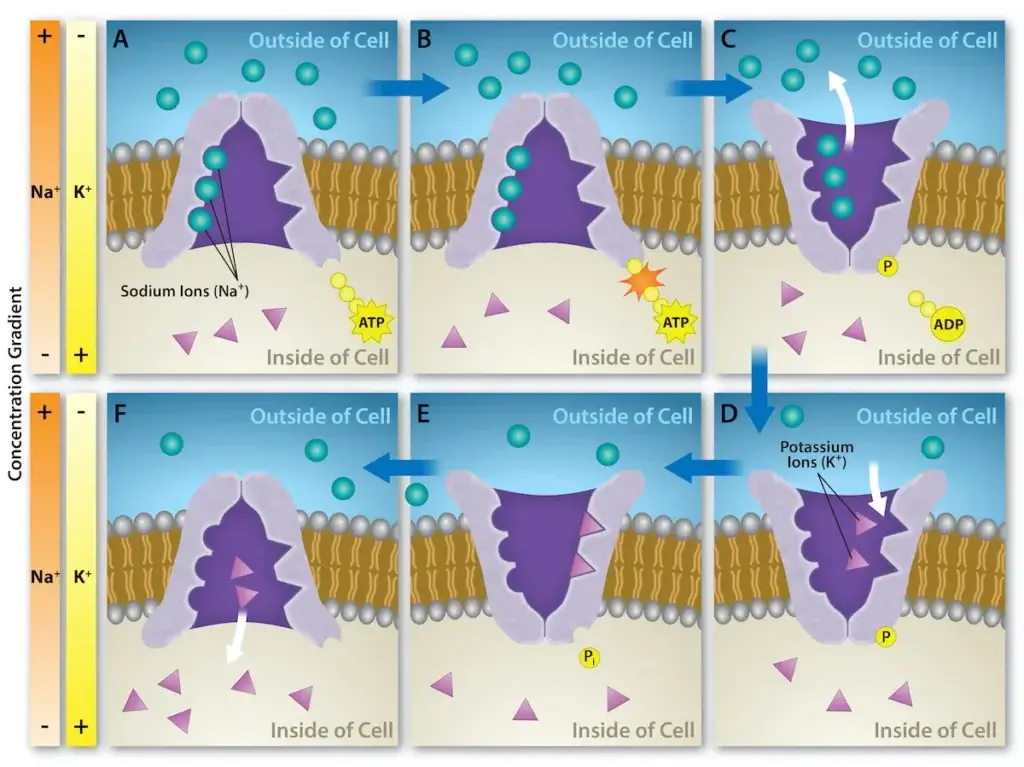
- The carrier protein of the Na+/K+ ATPase pump opens to the cell interior, allowing sodium ions (Na+) to bind to the high-affinity site on the pump.
- Binding of sodium ions to the carrier protein triggers ATP hydrolysis, resulting in the phosphorylation of the pump. This phosphorylation is achieved by transferring a phosphate group from ATP to the pump.
- The phosphorylation causes a conformational change in the pump, leading to a decrease in its affinity for sodium ions. As a result, the sodium ions are released from the pump to the extracellular space.
- While the pump has a low affinity for sodium ions, the conformational change creates a high-affinity binding site for potassium ions (K+). Potassium ions then bind to the pump, leading to the release of the phosphate group that was attached during the phosphorylation step.
- The release of the phosphate group triggers the pump to revert to its original conformation, allowing it to open inside the cell again.
- As the pump returns to its initial conformation, its affinity for sodium ions increases once more, restarting the cycle.
Ouabain, a cardiac glycoside, acts as an inhibitor of the Na+/K+ ATPase pump. It binds to the pump’s surface and prevents its dephosphorylation, thus blocking the transport of potassium ions into the cell.
The Na+/K+ ATPase pump is involved in various important functions in humans, including the generation of nerve impulses, muscle relaxation, secretion and absorption in the kidney, absorption of nutrients in the intestine, and other physiological processes. Its role in maintaining the proper balance of sodium and potassium ions is crucial for the overall function and integrity of cells.
2. F-ATPase
F-ATPase, also known as ATP synthase or ATP phosphohydrolase (H+-transporting), is an enzyme found in the mitochondrial inner membranes and chloroplast thylakoid membranes. It plays a crucial role in energy conversion by facilitating the synthesis of ATP using the energy derived from the movement of ions.
The F-ATPase enzyme functions as a rotary machine, utilizing ATP hydrolysis to pump hydrogen ions (H+) or sodium ions (Na+) across a membrane. It consists of two main domains: the F1 domain and the F0 domain.
The F1 domain is a hydrophilic catalytic globular domain consisting of an asymmetric hexameric ring and a central stalk at its center. This domain contains catalytic sites responsible for ATP hydrolysis and synthesis.
On the other hand, the F0 domain is responsible for the translocation of ions across the membrane. It is hydrophobic in nature and is embedded within the membrane.
During ATP synthesis, the F-ATPase uses the energy derived from the movement of protons across the membrane to drive the synthesis of ATP. The newly formed ATP is released from the active site of the F-ATPase.
In conditions of a lower driving force, the F-ATPase functions as an ATPase, consuming ATP to generate a transmembrane electrochemical gradient.
Structurally, the F-ATPase consists of the following components:
- Fo Domain: This domain is integrated into the membrane and comprises three integral proteins classified as a, b, and c. It is responsible for the translocation of ions across the membrane.
- F1 Domain: Composed of five polypeptide units – α (in three copies), β (in three copies), γ, δ, and ε. The F1 domain carries the catalytic sites for ATP synthesis and hydrolysis.
Examples of F-ATPase include mitochondrial ATP synthase and chloroplast ATP synthase, which are involved in ATP synthesis in oxidative phosphorylation and photosynthesis, respectively.
In summary, F-ATPase is an enzyme present in the mitochondrial and chloroplast membranes that plays a vital role in energy conversion. It utilizes the energy derived from the movement of ions to synthesize ATP. The F-ATPase consists of two domains, F1 and F0, which carry out the catalytic and translocation functions, respectively. This enzyme is essential for the production of ATP in various cellular processes.
3. V-ATPase
V-ATPase, also known as vacuolar proton-translocating ATPase, is an ATP-driven pump located in the tonoplast of the cell. It plays a crucial role in transporting protons across intracellular and plasma membranes in eukaryotic cells, leading to increased acidity within various cellular organelles compared to the surrounding cytoplasm. This acidification is essential for the proper functioning of these organelles.
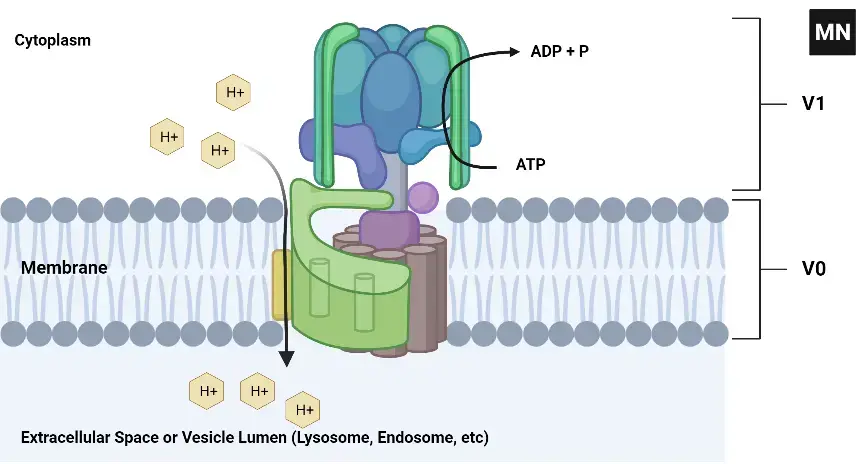
Structurally, V-ATPases are complex multimeric proteins composed of two functionally separate domains:
- V1 Domain: This domain consists of eight subunits and is responsible for ATP hydrolysis. It contains the catalytic site where ATP is broken down to release energy.
- V0 Domain: Comprising five subunits, the V0 domain is involved in the transport of protons across the membrane. It allows the movement of protons, contributing to the acidification of the organelle.
V-ATPases are critical molecular switches that play a role in toggling between anabolic and catabolic metabolism within cells. Disruptions or mutations in the structure or function of V-ATPases can have significant implications for cellular processes and human health. Changes in V-ATPase activity have been associated with conditions such as cancer, neurodegenerative diseases, and aging.
The energy derived from ATP hydrolysis is utilized by V-ATPases to drive the transport of protons across biological membranes. This activity contributes to maintaining the acidic environment necessary for the proper functioning of organelles and cellular processes.
In summary, V-ATPase is an ATP-driven pump located in the tonoplast of cells. It plays a vital role in transporting protons and acidifying various cellular organelles. The complex structure of V-ATPases includes the V1 domain for ATP hydrolysis and the V0 domain for proton transport. Disruptions to V-ATPase function can lead to various diseases, highlighting the importance of this enzyme in cellular homeostasis.
4. ABC (ATP binding cassette) transporter
- ABC (ATP binding cassette) transporters are a diverse class of transporter proteins found in organisms across the domains of bacteria, archaea, and eukarya. These transporters utilize the energy derived from ATP hydrolysis to facilitate the movement of solutes across biological membranes.
- Structurally, ABC transporters consist of four components: two membrane-integral domains that span the membrane six times each, and two ATP-hydrolyzing domains (ABC subunits/domains). The membrane-integral domains are responsible for interacting with the solutes to be transported, while the ATP-hydrolyzing domains provide the energy required for the transport process.
- In humans, approximately 48 ABC genes have been identified. Many of these genes are associated with various diseases, including cystic fibrosis, adrenoleukodystrophy, Stargardt disease, drug-resistant tumors, Dubin-Johnson syndrome, Byler’s disease, progressive familial intrahepatic cholestasis, X-linked sideroblastic anemia, ataxia, and persistent and hyperinsulinemic hypoglycemia. These transporters play crucial roles in disease pathology and have implications for drug resistance and therapeutic interventions.
- ABC transporters are involved in a wide range of biological processes. They participate in signal transduction, protein secretion, drug and antibiotic resistance, antigen presentation, bacterial pathogenesis, and sporulation. Examples of ABC transporters include the Multidrug Resistance Transporter (MDR) and the Cystic Fibrosis Transmembrane Conductance Regulator (CFTR).
- These transporters are found in various cellular locations, including chloroplasts, mitochondria, and plasma membranes. They play important roles in detoxification, phytohormone transport, and response to pathogens. By harnessing the energy released from ATP hydrolysis, ABC transporters enable the passage of solutes across membranes, contributing to vital cellular processes.
- In summary, ABC transporters are a diverse class of proteins that utilize ATP hydrolysis to transport solutes across biological membranes. They have crucial roles in disease development and resistance, as well as in various physiological processes. Understanding the functions and mechanisms of ABC transporters is important for unraveling their contributions to cellular physiology and human health.
B. Secondary Active Transport
Secondary active transport, also known as coupled transport or cotransport, is a mechanism of transportation that involves the coupled movement of ions and solutes across a biological membrane. This process relies on the pre-existing electrochemical gradient generated by the active transport of ions.
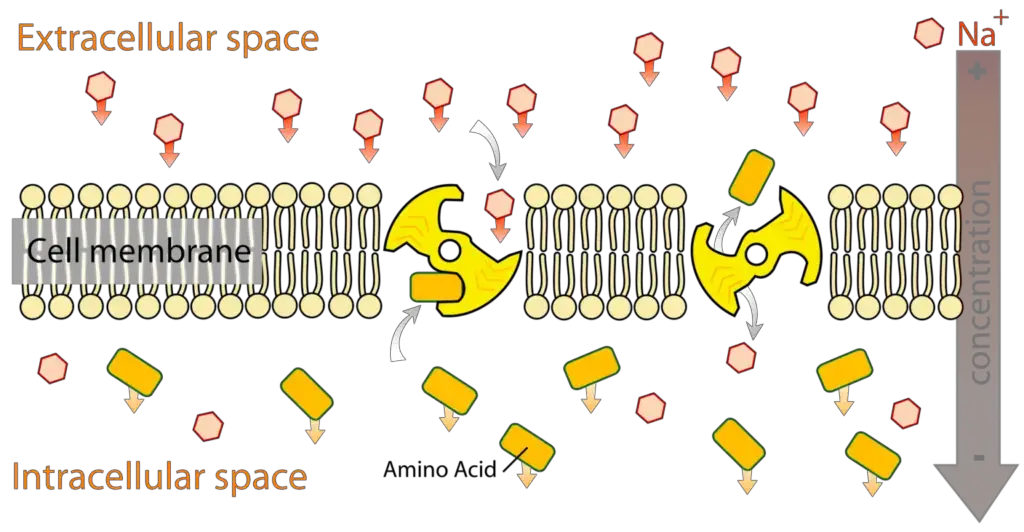
The process of secondary active transport can be summarized in two main steps:
- The generation of an ion’s electrochemical gradient: This is achieved through the active transport of an ion, such as H+, Na+, or K+, across the membrane, utilizing energy from ATP hydrolysis or an ion gradient itself.
- Coupling of the generated ion gradient to solute movement: The electrochemical gradient created by the movement of ions is then used to drive the movement of a solute in either the same direction (symport) or the opposite direction (antiport). In symport, both the driving ion and the solute move in the same direction across the membrane, while in antiport, they move in opposite directions.
The primary driving force for secondary active transport is the electrochemical gradient of ions. The ion gradient serves as the energy source to transport solutes against their concentration gradient, allowing them to be translocated across the membrane. This mode of transport is often observed in various organisms, with hydrogen (H+) ions commonly used in yeast and bacteria, and sodium (Na+) ions widely involved in secondary active transport in humans.
In summary, secondary active transport involves the coupled movement of ions and solutes across a biological membrane. The process utilizes the pre-existing electrochemical gradient generated by active transport of ions to drive the movement of solutes against their concentration gradient. This mode of transport plays a crucial role in the uptake of nutrients, reabsorption of ions in the kidney, and various other physiological processes in organisms.
Types of Secondary Active Transport
- Active transport is divided into two categories, Antiport and Symport, based on the direction of transfer between two molecules/solves across the membrane.
- The concentration capacity of the transport process assesses the level of effective transport for both antiport and symport. How well an ion or molecule is concentrated against a concentration gradient is the concentration capacity.
- For instance, in Na+/glucose cotransporter, the concentration ratio of glucose in and out of the membrane ([Glucose]i/[Glucose]o) determines the transporter’s ability to concentrate glucose within the cell against a concentration gradient.
- The concentration capacity is proportional to the ion/substrate coupling stoichiometry per transport cycle of transporters.
- For instance, the intestinal Na+/glucose cotransporter has a coupling stoichiometry of two Na+ ions to one glucose molecule (2:1) per transport cycle. The higher the ion/substrate coupling ratio, the greater the transporter’s concentration capacity.
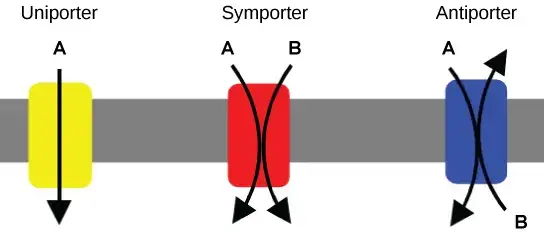
1. Antiport
Antiport, also known as counter-transporter or exchanger, is a transport mechanism in which two ions or solutes are pumped in opposite directions across a membrane. It plays a crucial role in maintaining ion and pH homeostasis in cells. Examples of antiporters include the Na+/Ca2+ exchanger (NCX), Na+/H+ exchanger (NHE), and Cl–/bicarbonate exchanger.
- The Na+/Ca2+ exchanger (NCX) is ubiquitously found in many cells and tissues and is essential for regulating cytoplasmic Ca2+ levels. This antiporter transports three Na+ ions down their electrochemical gradient while transporting one Ca2+ ion against its electrochemical gradient.
- The Na+/H+ exchanger (NHE) is also widely distributed and has an important role in regulating cytoplasmic pH. It transports one Na+ ion down its electrochemical gradient while transporting one H+ ion against its gradient with a coupling stoichiometry of 1:1.
- The Cl–/bicarbonate exchanger is an electroneutral exchanger. It transports one Cl– ion down its electrochemical gradient while transporting one HCO3– ion against its electrochemical gradient.
Antiporters are essential for maintaining ion balance, pH regulation, and other physiological processes in cells. By transporting ions or solutes in opposite directions, they contribute to the overall homeostasis of the cell.
In summary, antiport is a transport mechanism in which two ions or solutes are pumped in opposite directions across a membrane. Antiporters such as the Na+/Ca2+ exchanger, Na+/H+ exchanger, and Cl–/bicarbonate exchanger play vital roles in regulating ion concentrations and maintaining pH balance in cells. These antiporters ensure the proper functioning of cellular processes and contribute to overall cellular homeostasis.
2. Symport
Symport, also known as co-transport, is a transport mechanism in which two molecules are transported simultaneously across a membrane in the same direction. One molecule moves downhill, from an area of high concentration to low concentration, while the other molecule moves uphill, against its concentration gradient, from low concentration to high concentration.
Examples of symporters include:
- Na+/glucose cotransporter (SGLT1): This transporter is found in the intestinal epithelium. It transports two Na+ ions down their concentration gradient, providing the energy to transport one glucose molecule into the bloodstream across the apical membrane against its concentration gradient.
- GABA transporter (GAT): GAT belongs to a family of Na+ and Cl–-coupled transporters. It regulates the basal concentration of the inhibitory neurotransmitter γ-aminobutyric acid (GABA) in the nervous system and is involved in regulating its concentration and lifespan in the synaptic cleft.
- H+/oligopeptide transporter (PepT): PepT is located in the apical membrane of small intestine epithelial cells and proximal renal tubules. It transports one H+ ion down its concentration gradient while transporting one dipeptide or tripeptide across the membrane in the same direction against the concentration gradient. PepT also serves as the entry route for peptidomimetic drugs such as β-lactam antibiotics and plays a significant role in nitrogen absorption in the small intestine and reabsorption in the kidney tubules.
- Sodium-coupled bicarbonate cotransporters (NBC): NBC belongs to the family of Cl–/bicarbonate exchangers and is involved in maintaining acid-base balance in the body. The basolateral membrane of proximal kidney tubules mediates the outward translocation of one Na+ ion and three HCO3– ions into the interstitial fluid.
Symporters play important roles in various physiological processes by allowing the coupled movement of molecules against their concentration gradients. They contribute to nutrient absorption, neurotransmitter regulation, and ion balance in the body.
In summary, symport is a transport mechanism in which two molecules are transported in the same direction across a membrane. This process relies on the downhill movement of one molecule and the uphill movement of another molecule against its concentration gradient. Symporters are involved in the transport of important substances and play essential roles in various biological processes.
C. Bacterial Lactose Transport
- Bacterial lactose transport is a process that allows bacteria to take up lactose, a disaccharide composed of glucose and galactose. It operates in a manner similar to secondary active transport, utilizing the proton (H+) gradient generated by the bacterial electron transport system.
- Lactose transport is facilitated by a membrane protein called lactose permease, which belongs to the major facilitator superfamily. This protein spans the bacterial membrane with its 12 transmembrane alpha-helices and has a molecular weight of approximately 45,000 Daltons.
- The transport of lactose relies on the combination of electrical and pH gradients. The proton gradient, created by the electron transport system, provides the energy needed for lactose uptake. The electrical and pH gradients across the bacterial membrane contribute to the movement of lactose molecules into the cell.
- Through the activity of lactose permease, bacteria can efficiently transport and utilize lactose as a source of energy and carbon. This process is crucial for bacteria that can ferment lactose to produce energy, such as certain strains of Escherichia coli.
- In summary, bacterial lactose transport is mediated by lactose permease, a membrane protein that utilizes the proton gradient generated by the bacterial electron transport system. This process allows bacteria to take up lactose and utilize it as a nutrient source.
D. Bulk Transport
Endocytosis and exocytosis are bulk transport processes that use vesicles to transport materials across cell membranes.
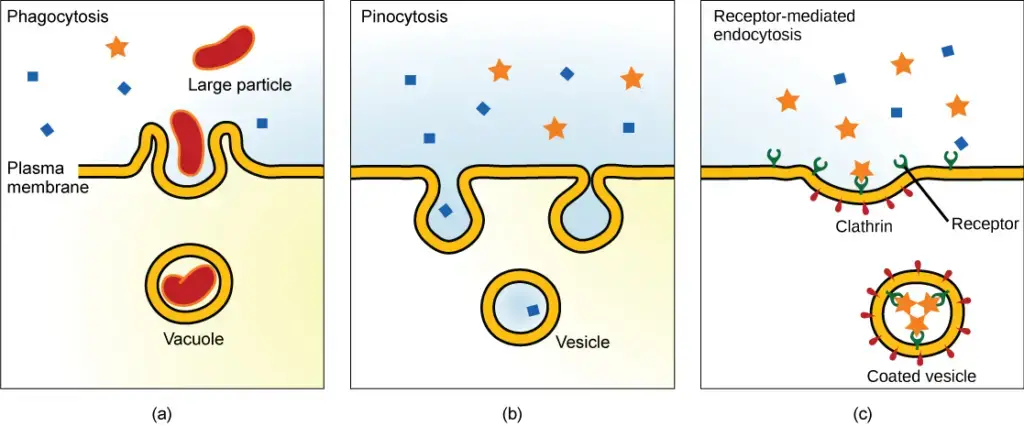
Endocytosis
- Endocytosis is a fundamental cellular process that enables the uptake of various substances into a cell by inward folding of the cell membrane. This intricate mechanism plays a crucial role in maintaining cellular homeostasis and facilitating the transportation of essential molecules.
- One type of endocytosis is phagocytosis, where solid particles are engulfed by the cell. This process is exemplified by the defense mechanism executed by white blood cells to combat bacteria and other pathogens. Neutrophils, for instance, surround, engulf, and destroy microorganisms that invade the human body, thereby safeguarding our health.
- Another type of endocytosis is pinocytosis, which involves the uptake of liquid particles by the cell. This process allows cells to absorb extracellular fluids and the dissolved substances within them. Pinocytosis is a non-selective form of endocytosis and is important for nutrient uptake and the removal of waste materials from the extracellular environment.
- Receptor-mediated endocytosis is a specialized variation of endocytosis that facilitates the targeted uptake of specific molecules. In this process, receptors present on the cell membrane bind to particular particles or protein molecules, initiating the invagination of the plasma membrane. Consequently, the targeted substances, along with associated proteins, are internalized into the cell. An example of receptor-mediated endocytosis is the removal of low-density lipoproteins (LDL) from the bloodstream. LDL receptors on the cell membrane bind to LDL particles, leading to their internalization and subsequent processing within the cell.
- Endocytosis is an essential cellular process that allows cells to regulate their internal environment, acquire nutrients, eliminate waste materials, and maintain proper signaling and communication. By understanding the different types of endocytosis, scientists can gain insights into various physiological processes and potentially develop therapeutic strategies targeting these mechanisms.
Exocytosis
- Exocytosis is a vital cellular process responsible for transporting molecules from the inside of the cell to the extracellular fluid, effectively releasing them into the surrounding environment. This mechanism involves the fusion of a vesicle with the plasma membrane, ensuring the targeted delivery of specific molecules to their intended destinations.
- During exocytosis, intracellular vesicles containing various substances, such as proteins, hormones, neurotransmitters, or waste materials, fuse with the cell’s plasma membrane. This fusion event allows the contents of the vesicle to be expelled into the extracellular fluid. The process of exocytosis plays a fundamental role in several biological functions, including intercellular communication, secretion of hormones and enzymes, synaptic transmission, and the removal of waste products.
- The fusion of the vesicle membrane with the plasma membrane is a precisely regulated process involving multiple molecular interactions. Specific proteins, known as SNARE proteins, play a crucial role in mediating the fusion event. These proteins help facilitate the docking and fusion of the vesicle membrane with the plasma membrane, ensuring the efficient release of the vesicle’s contents.
- Exocytosis serves as a key mechanism for cells to communicate with their surroundings and maintain proper physiological balance. For example, in neuronal cells, neurotransmitters stored within synaptic vesicles are released through exocytosis at the synapse, allowing for signal transmission between neurons. Similarly, in endocrine cells, hormones are synthesized, packaged into vesicles, and released into the bloodstream through exocytosis, regulating various physiological processes in distant target tissues.
- Moreover, exocytosis plays a critical role in the elimination of waste materials from cells. It enables cells to remove unwanted or toxic substances by expelling them into the extracellular space, where they can be further processed or eliminated by other cells or organs.
- Understanding the intricate process of exocytosis provides valuable insights into cellular communication, intercellular signaling, and the overall functioning of organisms. Further research into the mechanisms and regulation of exocytosis can contribute to the development of new therapeutic approaches targeting various diseases, including neurological disorders, endocrine dysfunctions, and immune system imbalances.
Active Transport in Plants
- In plants, just like in humans and animals, transport systems are crucial for the distribution of essential materials such as water, minerals, and nutrients to support their growth and survival.
- Active transport is a vital mode of transportation in plants, involving the utilization of stored energy to move particles against the concentration gradient. This process is particularly significant in root cells, where plants absorb water and minerals from the soil. Unlike passive transport, which relies on diffusion and does not require energy, active transport allows plants to accumulate molecules and ions on one side of the membrane, even when the concentration gradient favors the opposite direction.
- Active transport in plants is facilitated by specific membrane proteins that actively transport substances from areas of lower concentration to areas of higher concentration. These membrane proteins, often referred to as transporters or pumps, are responsible for the movement of various ions, minerals, and other necessary molecules across cell membranes.
- By actively transporting substances against the concentration gradient, plants can absorb essential nutrients, such as nitrate ions and minerals like potassium and calcium, from the soil into their root cells. This process enables plants to obtain the necessary elements for growth and development, even when the concentration of these substances in the soil is lower than in the plant cells.
- The energy required for active transport in plants is derived from adenosine triphosphate (ATP), the primary energy currency in cells. ATP provides the necessary energy to power the transport proteins and drive the movement of substances across cell membranes.
- Active transport is a crucial mechanism for plants to maintain homeostasis, regulate nutrient uptake, and respond to environmental cues. It allows plants to adapt to varying conditions and optimize their nutrient acquisition, even in nutrient-deficient soils.
- Understanding the mechanisms and regulation of active transport in plants is of great importance in agriculture and crop production. By studying how plants efficiently absorb and transport nutrients, researchers can develop strategies to enhance nutrient uptake in crops, improve their resilience to environmental stresses, and ultimately increase agricultural productivity.
- In conclusion, active transport is a vital process in plants that enables them to absorb water, minerals, and nutrients against the concentration gradient. Through the utilization of energy and specific membrane proteins, plants can accumulate essential substances in their cells, supporting their growth and survival in various environments.
Differences Between Primary active transport and Secondary active transport
Primary active transport, also known as direct active transport, and secondary active transport, also referred to as coupled transport or cotransport, are two distinct mechanisms by which substances are transported across cell membranes. While they share some similarities, they differ in several key aspects.
- Synonyms: Primary active transport is synonymous with direct active transport, highlighting the direct coupling of ATP to the transport process. Secondary active transport is synonymous with coupled transport or cotransport, emphasizing the reliance on an existing electrochemical gradient.
- Energy Source: In primary active transport, the energy used for transportation comes from metabolic energy in the form of ATP. This can also involve other forms of energy such as redox energy or photon energy. In contrast, secondary active transport utilizes the energy stored in an electrochemical gradient, which is typically established by primary active transport.
- Direct Coupling of ATP: Primary active transport directly couples ATP to the transport process. Membrane protein transporters, including P-type ATPases (e.g., sodium-potassium pump, calcium pump, proton pump), F-ATPases (e.g., mitochondrial ATP synthase, chloroplast ATP synthase), V-ATPase (vacuolar ATPase), and ATP-binding cassette transporters (e.g., MDR, CFTR), are involved in this mechanism. They use ATP to drive the movement of ions or molecules across the membrane.
- No Direct Coupling of ATP: Secondary active transport does not directly couple ATP to the transport process. Instead, it relies on the pre-established electrochemical gradient generated by primary active transport. The energy stored in this gradient is harnessed to transport substances against their concentration gradient.
- Membrane Protein Transporters: Primary active transport involves specific membrane protein transporters such as P-type ATPases, F-ATPases, V-ATPases, and ATP-binding cassette transporters. These transporters actively move ions or molecules across the membrane by utilizing ATP as an energy source. In contrast, secondary active transport relies on cotransporters, including antiporters and symporters, to facilitate the movement of substances in conjunction with the movement of ions down their electrochemical gradient.
- Examples: An example of primary active transport is the ATP-dependent sodium-potassium pump, which transports three sodium ions out of the cell while moving two potassium ions into the cell. This process directly uses ATP to fuel ion movement.
In secondary active transport, a common example involves the transport of a second substrate while another ion, typically sodium, potassium, or hydrogen ions, moves down its concentration gradient. The energy derived from the pre-existing ion gradient drives the cotransport of the second substrate.
In summary, primary active transport relies on the direct coupling of ATP to fuel the movement of substances, while secondary active transport utilizes the energy stored in an electrochemical gradient established by primary active transport. These mechanisms play crucial roles in maintaining proper cellular function and are essential for various physiological processes.
Aspects | Primary Active Transport | Secondary Active Transport |
---|---|---|
Synonym(s) | Direct Active Transport | Coupled Transport or Cotransport |
Direct Coupling of ATP | Yes | No |
Energy Used | Metabolic energy (ATP), Redox energy, Photon energy | Electrochemical gradient |
Membrane Protein Transporter | P-type ATPase (e.g., sodium-potassium pump, calcium pump, proton pump), F-ATPase (e.g., mitochondrial ATP synthase, chloroplast ATP synthase), V-ATPase (vacuolar ATPase), ATP-binding cassette transporter (e.g., MDR, CFTR) | Cotransporters: Antiporters, Symporters |
Examples | Active transport using ATP via sodium-potassium pump to move 3 Na+ ions out while moving 2 K+ ions into the cell | Active transport of a second substrate while another ion, typically Na+, K+, or H+ ions, move down the concentration gradient |
Difference Between Active Transport and Passive Transport
Active transport and passive transport are two fundamental processes that play essential roles in the movement of molecules across cell membranes. While both processes involve the transportation of molecules, they differ in several key aspects. Let’s explore the differences between active transport and passive transport.
- Energy Requirement: Active Transport: Active transport requires cellular energy, usually in the form of ATP (adenosine triphosphate). This energy is used to transport molecules against their concentration gradient, from an area of lower concentration to an area of higher concentration. Passive Transport: In contrast, passive transport does not require cellular energy. It occurs spontaneously, driven by the concentration gradient, and molecules move from an area of higher concentration to an area of lower concentration.
- Types of Molecules Transported: Active Transport: Active transport is responsible for the transportation of various molecules, including proteins, large cells, complex sugars, and ions. It is involved in the movement of specific molecules necessary for cellular processes. Passive Transport: Passive transport is involved in the transportation of soluble molecules such as oxygen, water, carbon dioxide, lipids, and sex hormones. It facilitates the movement of these molecules across the cell membrane.
- Process Characteristics: Active Transport: Active transport is a dynamic process. It requires the participation of carrier proteins embedded in the cell membrane, which actively pump molecules against the concentration gradient. This process is highly selective and allows the cell to regulate the movement of specific substances. Passive Transport: Passive transport is a physical process that occurs through diffusion or facilitated diffusion. It is partly non-selective, meaning it allows various molecules to pass through the membrane. Passive transport is generally slower compared to active transport.
- Direction of Transport: Active Transport: Active transport transpires in one direction, from an area of lower concentration to an area of higher concentration. Passive Transport: Passive transport can transpire bidirectionally. Molecules can move in either direction depending on the concentration gradient.
- Influence of Temperature: Active Transport: Active transport is influenced by temperature. As the temperature increases, the rate of active transport generally increases as well. Passive Transport: Passive transport is not significantly influenced by temperature.
- Requirement of Carrier Proteins: Active Transport: Active transport relies on carrier proteins present in the cell membrane. These proteins undergo conformational changes to transport molecules across the membrane. Passive Transport: Carrier proteins are not required for passive transport. Molecules move across the membrane through simple diffusion or facilitated diffusion.
- Oxygen Content Effect: Active Transport: Active transport is affected by the level of oxygen content. As the oxygen content decreases, the process of active transport reduces or halts. Passive Transport: Passive transport is not influenced by the level of oxygen content.
- Influence of Metabolic Inhibitors: Active Transport: Active transport can be influenced or stopped by metabolic inhibitors. These inhibitors disrupt the cellular energy production required for active transport. Passive Transport: Passive transport is not influenced by metabolic inhibitors.
Examples of Active Transport: Active transport includes processes such as exocytosis, endocytosis, and the sodium-potassium pump.
Examples of Passive Transport: Passive transport includes processes such as osmosis, diffusion, and facilitated diffusion.
In conclusion, active transport and passive transport differ in terms of energy requirement, directionality, selectivity, involvement of carrier proteins, and sensitivity to factors like temperature and oxygen content. Understanding these differences is crucial for comprehending the intricate mechanisms by which cells transport molecules across their membranes.
Aspects | Active Transport | Passive Transport |
---|---|---|
Energy Requirement | Requires cellular energy | Does not require cellular energy |
Direction of Transport | Lower concentration to higher | Higher concentration to lower |
Molecules Transported | Proteins, large cells, complex sugars | Oxygen, water, carbon dioxide, etc. |
Process Characteristics | Dynamic process | Physical process |
Selectivity | Highly selective | Partly non-selective |
Speed of Transport | Rapid | Comparatively slow |
Transport Direction | Unidirectional | Bidirectional |
Influence of Temperature | Influenced by temperature | Not influenced by temperature |
Requirement of Carrier Proteins | Required | Not required |
Oxygen Content Effect | Reduces/halts at low oxygen content | Not affected by oxygen content |
Influence of Metabolic Inhibitors | Can be influenced/stopped | Not influenced by inhibitors |
Examples of Transport Processes | Exocytosis, endocytosis, sodium-potassium pump | Osmosis, diffusion, facilitated diffusion |
Examples of Active Transport
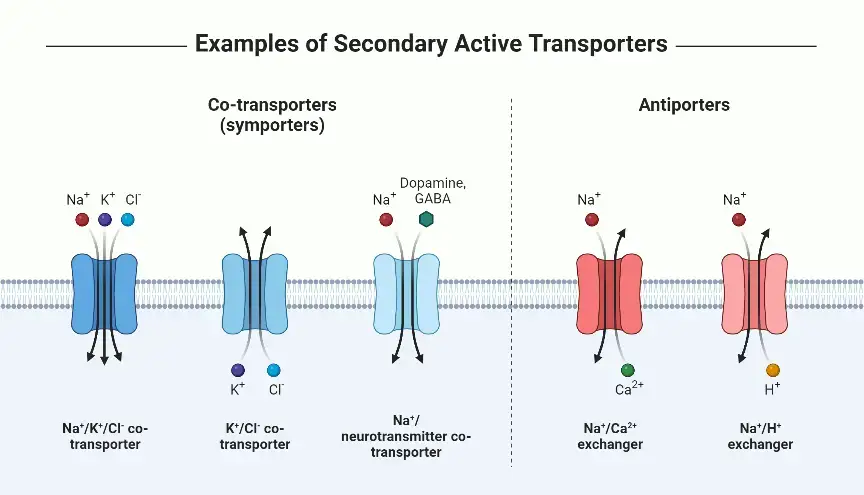
1. Sodium Potassium Pump
- The sodium-potassium pump is a crucial active transport protein found in animals, and it plays a vital role in maintaining the ion concentrations necessary for the proper functioning of the nervous system.
- The functioning of our nervous system relies on maintaining a difference in ion concentrations between the inside and outside of nerve cells. This difference, known as the ion gradient, is essential for various physiological processes, including muscle contractions, sensations, and neuronal communication.
- The sodium-potassium pump is responsible for establishing and maintaining this ion gradient by actively transporting potassium ions into the cells while simultaneously transporting sodium ions out of the cells. This process requires energy in the form of adenosine triphosphate (ATP), which is derived from the breakdown of nutrients obtained from food.
- The importance of the sodium-potassium pump is highlighted by the significant amount of energy dedicated to its functioning. Estimates suggest that approximately 20-25% of the energy derived from our food is utilized solely for the activity of this pump. In neurons, a majority of the cell’s energy is devoted to powering the sodium-potassium pumps.
- The sodium-potassium pump performs a monumental task that impacts various aspects of our lives. It enables us to move by maintaining the ion gradients necessary for muscle contractions. It also supports our cognitive functions, allowing us to think and process information. Additionally, the pump plays a critical role in maintaining the rhythm and contractility of our heart muscle, contributing to the proper circulation of blood throughout our bodies.
- While the energy expenditure associated with the sodium-potassium pump might seem substantial, its importance cannot be overstated. This pump is essential for our ability to interact with the world around us, perceive sensory information, initiate voluntary movements, and carry out vital physiological functions.
2. Sodium-Glucose Transport Protein
- The sodium-glucose transport protein is a well-known example of a symport pump, a type of protein that transports two different molecules simultaneously. This specific protein binds to two sodium ions and one glucose molecule, allowing for their coordinated movement across the cell membrane. It plays a crucial role in sugar transport within the body, providing the necessary energy for cellular respiration.
- The symport pump works by taking advantage of the natural diffusion of sodium ions inside the cell. Sodium ions tend to move into the cell due to a concentration gradient. By binding to two sodium ions, the sodium-glucose transport protein creates a favorable environment for glucose transport.
- The glucose molecule, which would normally prefer to stay outside the cell, is drawn along with the sodium ions due to their combined movement. This coordinated transport allows glucose to enter the cell without the transport protein expending adenosine triphosphate (ATP), the cellular energy currency. However, it is important to note that ATP is still required elsewhere in the cell to maintain the sodium gradient necessary for the symport pump to function properly.
- The sodium-potassium pump, a separate protein, utilizes ATP to actively transport sodium ions out of the cell and potassium ions into the cell. This process helps maintain the concentration gradient of sodium ions across the cell membrane, which is essential for the functioning of the sodium-glucose transport protein. Without a proper sodium gradient, the symport pump would not be able to transport glucose effectively.
- The sodium-glucose transport protein is of great significance in the body’s energy metabolism. By facilitating the transport of glucose into cells, it ensures a constant supply of this vital energy source for cellular respiration. This mechanism is particularly important in tissues with high energy demands, such as the brain and muscles.
3. WBCs Destroying Pathogens
- White blood cells play a crucial role in our immune system’s defense against pathogens, and their ability to destroy these invaders is facilitated by a process called endocytosis. When white blood cells detect the presence of foreign objects, such as bacteria or other pathogens, they employ endocytosis to engulf and eliminate these threats.
- Upon recognizing a pathogen, white blood cells initiate the process by folding their cell membrane around the invader. This inward folding forms a pocket, effectively enveloping the pathogen and enclosing it within the white blood cell’s cytoplasm. This process is akin to the cell membrane creating a protective bubble around the pathogen.
- Once the pathogen is internalized within the white blood cell, a crucial step occurs: the merging of the vesicle containing the invader with a lysosome. Lysosomes are specialized vesicles that contain potent chemicals and enzymes capable of breaking down and digesting organic matter. In this case, the white blood cell effectively creates a cellular “stomach” by merging the vesicle containing the pathogen with the lysosome.
- The lysosome’s strong chemicals and enzymes are then unleashed upon the pathogen, initiating a process of degradation and digestion. The enzymes within the lysosome break down the components of the pathogen into smaller, more manageable pieces. This breakdown allows the white blood cell to neutralize and eliminate the pathogen effectively.
- By employing this cellular “stomach” mechanism, white blood cells can destroy pathogens and prevent their further spread within the body. This process is crucial for maintaining a healthy immune system and protecting against infectious diseases.
- The ability of white blood cells to use endocytosis and the subsequent merging with lysosomes to destroy pathogens showcases the intricate and efficient nature of our immune system. This mechanism highlights the remarkable cellular processes at play, demonstrating how our body defends itself against harmful invaders.
Functions of Active Transport
Active transport is a vital process in cellular physiology that allows the movement of substances against their concentration gradient, requiring the expenditure of energy in the form of ATP. Here are some key functions of active transport:
- Nutrient Absorption: Active transport plays a crucial role in the absorption of essential nutrients from the environment or the digestive tract. For example, in the small intestine, active transport mechanisms are responsible for the uptake of ions such as sodium, potassium, calcium, and iron, as well as the absorption of glucose and amino acids.
- Ion Homeostasis: Active transport maintains proper ion concentrations within cells, contributing to the maintenance of cellular homeostasis. Sodium-potassium pumps, for instance, actively transport sodium ions out of the cell and potassium ions into the cell, establishing and preserving the electrochemical gradient necessary for various cellular processes.
- Maintenance of Membrane Potential: Active transport mechanisms, including sodium-potassium pumps and proton pumps, help establish and maintain the membrane potential across cell membranes. This electrical potential difference is vital for the transmission of nerve impulses, muscle contractions, and the functioning of excitable cells.
- Removal of Waste Products: Active transport is involved in the elimination of waste products from cells. For instance, in the kidneys, active transport mechanisms reabsorb valuable molecules such as glucose and amino acids from the filtrate while actively secreting waste products like urea and uric acid into the urine.
- Cell Signaling and Communication: Active transport systems, such as the sodium-calcium exchanger and sodium-hydrogen exchanger, contribute to cell signaling and communication. These transporters regulate the intracellular concentrations of calcium and hydrogen ions, which play crucial roles in various signaling pathways and cellular responses.
- Drug Efflux: Active transporters, such as ATP-binding cassette (ABC) transporters, are involved in the efflux of drugs and toxins from cells. These transporters actively pump drugs out of cells, contributing to the development of drug resistance in certain diseases.
- Absorption of Nutrients by Plants: Active transport is essential for nutrient uptake by plant roots. Active transport mechanisms in root cells actively absorb essential ions, such as potassium, nitrate, and phosphate, from the soil against their concentration gradient.
- Maintenance of pH and Ionic Balance: Active transport systems help regulate pH and ionic balance within cellular compartments. For instance, active proton pumps in the stomach lining secrete hydrogen ions (H+) to maintain the acidic environment required for digestion.
Active transport is a dynamic process that enables cells to maintain proper nutrient levels, control ion concentrations, generate electrical potentials, and perform various physiological functions essential for cellular health and survival.
FAQ
What is active transport?
Active transport is a cellular process that moves molecules or ions across a cell membrane against their concentration or electrochemical gradient, requiring the expenditure of energy in the form of ATP.
How does active transport differ from passive transport?
Active transport differs from passive transport in that it moves substances against their concentration gradient, while passive transport moves substances along their concentration gradient without the need for energy.
What is the role of ATP in active transport?
ATP provides the energy needed for active transport. ATP is hydrolyzed, releasing a phosphate group, and this energy is utilized by carrier proteins or pumps to transport molecules or ions against their gradient.
What are the types of carrier proteins involved in active transport?
There are three types of carrier proteins involved in active transport: uniporters, symporters, and antiporters. Uniporters transport a single ion or molecule, while symporters and antiporters transport two ions or molecules in the same or opposite directions, respectively.
Can active transport transport small, uncharged organic molecules?
Yes, active transport can transport small, uncharged organic molecules such as glucose. Carrier proteins involved in active transport are capable of facilitating the movement of these molecules across the cell membrane.
How do active transport mechanisms maintain ion concentrations in cells?
Active transport mechanisms actively maintain ion concentrations by pumping ions against their concentration gradient. For example, the Na+-K+ ATPase pump helps maintain low intracellular sodium and high intracellular potassium concentrations.
Are active transport processes selective?
Yes, active transport processes are highly selective. Carrier proteins involved in active transport exhibit specificity for certain molecules or ions, allowing them to transport specific substances across the membrane.
How is active transport affected by metabolic inhibitors?
Active transport processes are sensitive to metabolic inhibitors that interfere with ATP production or utilization. Inhibition of ATP supply can disrupt the energy source required for active transport, leading to impaired transport activity.
Can active transport occur in both directions?
Active transport typically occurs in one direction, as it moves substances against their concentration gradient. However, there are cases where active transport can occur bidirectionally, depending on the specific transport mechanism and cellular requirements.
What are some examples of active transport processes?
Examples of active transport processes include the Na+-K+ pump, which maintains ion gradients in nerve cells and muscle cells, and the H+-K+ ATPase pump, involved in the secretion of stomach acid. Additionally, endocytosis and exocytosis are active transport processes involved in the uptake and release of large molecules or particles by cells.
References
- https://conductscience.com/active-transport-definition-types-and-examples/
- https://www.biologyonline.com/dictionary/active-transport
- https://openstax.org/books/biology-2e/pages/5-3-active-transport
- https://biologydictionary.net/active-transport/#examples-of-active-transport
- https://courses.lumenlearning.com/suny-biology1/chapter/active-transport/
- https://www.aakash.ac.in/important-concepts/biology/active-transport
- https://openstax.org/books/concepts-biology/pages/3-6-active-transport
- https://teachmephysiology.com/biochemistry/molecules-and-signalling/active-transport/
- https://www.geeksforgeeks.org/what-is-active-transport-definition-types-and-examples/
- https://studymind.co.uk/notes/transport-across-membranes-active-transport/