What is Photosynthesis?
- Photosynthesis is a fundamental biochemical process that harnesses the energy of light to synthesize glucose molecules. This intricate mechanism can be delineated into two primary stages. Initially, light energy is captured and transformed into chemical energy, stored within the molecules of adenosine triphosphate (ATP) and nicotinamide adenine dinucleotide phosphate (NADPH).
- Subsequently, these energy-rich cofactors participate in the Calvin Cycle, a series of reactions that facilitate the synthesis of organic molecules by assimilating carbon atoms from carbon dioxide (CO2). These organic molecules can either be utilized by mitochondria to generate ATP or amalgamated to yield glucose, sucrose, and other carbohydrates.
- Scientifically defined, photosynthesis is a cellular process employed by a myriad of organisms to transmute light energy into chemical energy. This stored chemical energy is embedded within organic compounds, which, upon metabolism via cellular respiration, release energy essential for the organism’s functions.
- Predominantly, the term “photosynthesis” alludes to oxygenic photosynthesis, characterized by the production of oxygen as a byproduct and the storage of some resultant chemical energy within carbohydrate molecules, including sugars, starch, and cellulose.
- This form of photosynthesis is executed by a majority of plants, algae, and cyanobacteria, collectively termed as photoautotrophs. Notably, photosynthesis plays a pivotal role in sustaining Earth’s atmospheric oxygen levels and furnishing the majority of biological energy requisite for Earth’s complex life forms.
- However, certain bacteria undergo anoxygenic photosynthesis, utilizing bacteriochlorophyll to cleave hydrogen sulfide, leading to the production of sulfur instead of oxygen. Furthermore, specific Archaea, such as Halobacterium, engage in a distinct form of non-carbon-fixing anoxygenic photosynthesis, leveraging simpler photopigments like retinal to absorb light and directly synthesize ATP. This archaic form of photosynthesis is postulated to be one of the earliest evolutionary adaptations on Earth.
- Regardless of the organism or method, photosynthesis invariably commences with the absorption of light energy by proteins known as reaction centers, which contain photosynthetic pigments or chromophores. In plants, these proteins, predominantly chlorophyll, are housed within organelles termed chloroplasts. During the light-dependent reactions, electrons are extracted from substances like water, resulting in the release of oxygen gas. The liberated hydrogen aids in the formation of NADPH and ATP.
- In plants, algae, and cyanobacteria, the Calvin cycle subsequently facilitates the synthesis of sugars. Here, atmospheric carbon dioxide is integrated into pre-existing organic carbon compounds, utilizing the ATP and NADPH generated earlier. In certain bacteria, alternative mechanisms achieve carbohydrate synthesis.
- Historically, the earliest photosynthetic organisms likely utilized reducing agents such as hydrogen or hydrogen sulfide. The advent of cyanobacteria marked a significant evolutionary milestone, as the surplus oxygen they generated significantly influenced Earth’s atmospheric composition, paving the way for the evolution of complex life forms.
- Presently, photosynthesis captures energy at an astounding rate of approximately 130 terawatts, surpassing the current global power consumption. Furthermore, photosynthetic organisms assimilate between 100–115 billion tons of carbon annually.
- In conclusion, photosynthesis is not only a cornerstone of life on Earth but also plays a crucial role in global climate processes by sequestering carbon dioxide. This intricate process, first identified by Jan Ingenhousz in 1779, remains a testament to nature’s ingenuity and efficiency.
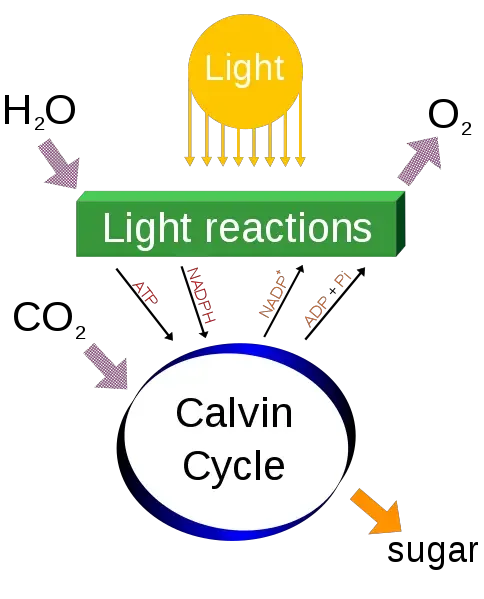
Definition of Photosynthesis
Photosynthesis is the biological process by which plants, algae, and certain bacteria convert light energy into chemical energy, producing oxygen and organic compounds, primarily glucose, from carbon dioxide and water.
Experimental History
The intricate process of photosynthesis, which underpins life on Earth, has been the subject of extensive scientific inquiry over the centuries. This journey of discovery, refinement, and development has been marked by the contributions of numerous scientists, each building upon the work of their predecessors. Below is a summarized account of the experimental history of photosynthesis:
No. | Scientist | Key Contribution |
---|---|---|
1 | Stephen Hales | Identified the significance of air and light in plant growth. |
2 | Joseph Priestley | Highlighted the essential role of air in the growth of green plants. |
3 | Jan Ingenhousz | Elucidated the pivotal role of sunlight in enabling plants to release oxygen. |
4 | Jene Senebier | Established that plants consume carbon dioxide (CO2). |
5 | N.T. de Saussure | Demonstrated the equivalence between the volume of CO2 consumed and O2 released by plants. |
6 | Pelletier and Caventou | Introduced the term “chlorophyll.” |
7 | F.F. Blackman | Proposed the two-step nature of photosynthesis and introduced the “Law of limiting factors.” |
8 | Warburg | Conducted flashing experiments in Chlorella. |
9 | Emerson and Arnold | Identified two distinct photochemical processes in the initial stage of light reactions. |
10 | Robert Hill | Demonstrated that oxygen release by chloroplasts does not necessitate CO2 but requires sunlight, water, and a hydrogen acceptor. His contribution led to the naming of the light reaction as “Hill’s reaction.” |
11 | Van Niel | Confirmed that oxygen originates from water, not CO2, and that hydrogen from oxidizable compounds reduces CO2 to carbohydrates. |
12 | Ruben, Hassid, Kamen | Validated, using radioactive studies, that the oxygen source in photosynthesis is water. |
13 | Julius von Sachs | Identified chlorophyll-containing green parts of plants as the primary sites of photosynthesis. |
14 | T.W. Engelmann | Defined the significance of red and blue light wavelengths and introduced the first action spectrum of photosynthesis. |
15 | Melvin Calvin | Detailed the reactions converting CO2 to sugars, leading to the naming of the C3 cycle in his honor. |
16 | M.D. Hatch and C.R. Slack | Described the C4 cycle, also known as the Hatch and Slack cycle. |
17 | Hill and Bendall | Proposed a scheme for the light reaction. |
18 | Huber et al. | Investigated the three-dimensional structure of the reaction center. |
19 | Charles Reid Barnes | Introduced the terms “Photosyntax” and “photosynthesis.” |
Where Does Photosynthesis take place?
Photosynthesis primarily occurs in the chloroplasts, specialized organelles found predominantly in plant leaves. These chloroplasts belong to a category of organelles known as plastids, which are membrane-bound structures responsible for various vital cellular functions.
- Chloroplast Structure: Chloroplasts are a type of primary plastid characterized by a double-membrane structure. They house the essential green pigment, chlorophyll, which plays a pivotal role in capturing light energy. In contrast, secondary plastids, found in certain plankton species, possess multiple membranes.
- Role of Chlorophyll: Chlorophyll pigments are responsible for absorbing light energy from the sun. Upon absorption, a chlorophyll molecule releases an electron, initiating the process of converting light energy into chemical energy.
- Reaction Centers: These are specialized complexes composed of pigments and proteins. They serve as the primary sites where light energy is transformed into chemical energy, marking the onset of electron transfer. The energy captured by the reaction centers is crucial for driving the subsequent biochemical reactions of photosynthesis.
In summary, photosynthesis is a complex process that takes place within the chloroplasts of plant cells. The chlorophyll pigments housed within these organelles are instrumental in capturing and converting light energy into a form that can be utilized by the plant for growth and sustenance.
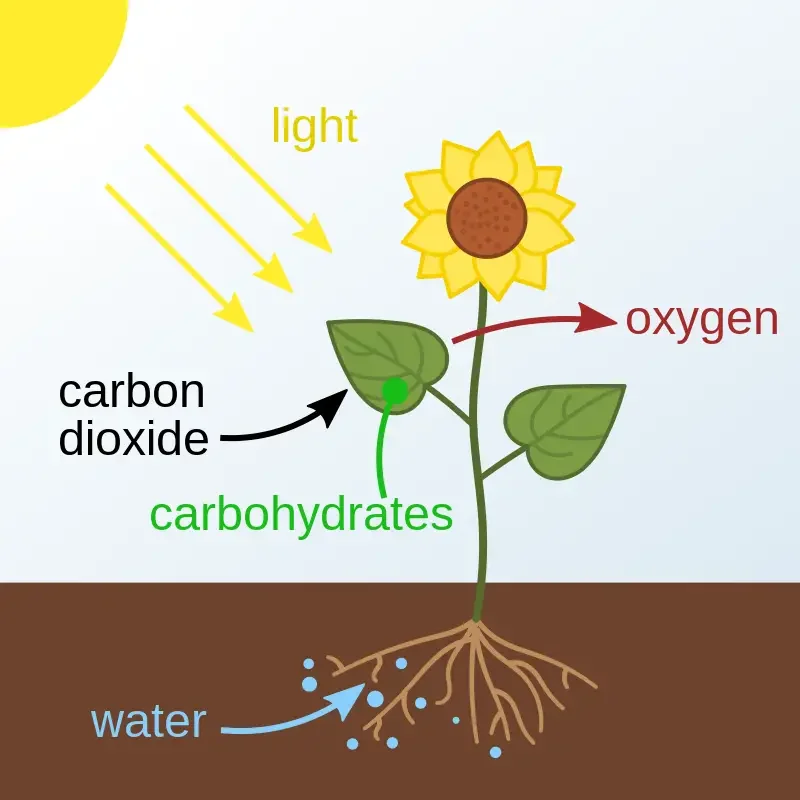
Photosynthesis: a two-stage process
Photosynthesis, a fundamental biochemical process, can be delineated into two distinct stages. Contrary to the traditional classification of these stages as ‘light’ and ‘dark’ reactions, contemporary scientific understanding emphasizes that both stages are influenced by light, albeit in different capacities.
1. Photochemical Reaction Process (Light-Dependent Reactions):
In this initial stage, light energy, primarily from the sun, is harnessed and converted into chemical energy in the form of adenosine triphosphate (ATP). This process, known as photophosphorylation, is contingent upon the presence of light. During this phase:
- Photons are absorbed by photosynthetic pigments, initiating a series of electron transfer events.
- The energy derived from these events drives the synthesis of ATP and the electron carrier molecule, nicotinamide adenine dinucleotide phosphate (NADPH).
- Oxygen is released as a byproduct when water molecules are split.
2. Carbon Fixation Process (Light-Independent Reactions):
While this stage does not directly utilize light energy, it is influenced by the products of the light-dependent reactions. The primary objective here is the conversion of inorganic carbon into organic compounds. This energy-consuming, endergonic process can manifest in two distinct pathways:
- Calvin Cycle: This is the predominant pathway in both oxygenic and anoxygenic photosynthesis. During this cycle:
- Carbon dioxide from the atmosphere is captured and integrated into existing organic molecules.
- The ATP and NADPH produced in the light-dependent reactions provide the energy and electrons, respectively, for the synthesis of glucose and other carbohydrates.
- Non-Calvin Cycle: This alternative pathway is exclusive to certain anoxygenic photosynthetic organisms. It diverges from the Calvin cycle in its mechanism of carbon fixation and the compounds produced.
In summation, photosynthesis is a two-stage process wherein light energy is first converted into chemical energy, which subsequently powers the synthesis of organic compounds from inorganic carbon. Both stages, while functionally distinct, are interdependent and collectively contribute to the sustenance of life on Earth.
Photosynthesis equations/reactions/formula

Photosynthesis, a fundamental biochemical process, varies between green plants and sulfur bacteria in terms of the reactants used and the products formed.
Oxygenic Photosynthesis in Plants:
In green plants, photosynthesis utilizes water and carbon dioxide, harnessing solar energy to produce glucose and oxygen. The overarching equation representing this process is:
6CO2+6H2O+light energy→C6H12O6+6O2
Alternatively, it can be represented as:
6CO2+12H2O+light energy→C6H12O6+6O2+6H2O
This equation signifies that six molecules of carbon dioxide and twelve molecules of water, in the presence of light energy, yield one molecule of glucose, six molecules of oxygen, and six molecules of water. It’s noteworthy that while a triose (3-carbon molecule) is the direct product of photosynthesis, glucose, a hexose, is often depicted as the end product due to its foundational role in cellular processes. Additionally, the oxygen produced serves dual purposes: it is utilized by the plant during oxidative phosphorylation and is also released into the atmosphere, facilitating aerobic respiration in other organisms.
Anoxygenic Photosynthesis in Sulfur Bacteria:
Sulfur bacteria employ a distinct form of photosynthesis. Instead of water, they use hydrogen sulfide in conjunction with carbon dioxide. The general equation for this process is:
CO2+2H2S+light energy→(CH2O)+H2O+2S
This equation illustrates that carbon dioxide and hydrogen sulfide, under the influence of light energy, produce carbohydrates, water, and elemental sulfur.
In summation, photosynthesis, whether in plants or sulfur bacteria, is a series of intricate reactions that convert simple molecules into energy-rich compounds, using light as the primary energy source. The specific reactants and products differ based on the organism, but the core principle of harnessing light energy remains consistent.
Types of Photosynthesis
Photosynthesis, the fundamental process by which organisms convert light energy into chemical energy, manifests in various forms across different species. These diverse pathways are tailored to the specific environmental conditions and metabolic needs of the organisms. Here, we delve into the primary types of photosynthesis and their distinctive characteristics.
- Carbon Fixation Pathways:
- C3 Photosynthesis:
- C4 Photosynthesis:
- Organisms: Plants such as sugarcane and maize.
- Process: CO2 is initially fixed into a four-carbon compound, oxaloacetate, which is subsequently converted to malate. This malate is transported to the bundle sheath cells, where it releases CO2. This CO2 is then utilized in the Calvin cycle, akin to the C3 pathway. Notably, C4 plants exhibit enhanced water-use efficiency in hot, arid conditions.
- CAM Photosynthesis:
- Organisms: Adapted to extremely arid environments, plants like cacti and pineapples employ this pathway.
- Process: To mitigate water loss, these plants open their stomata during the cooler nighttime hours, absorbing CO2. This CO2 is fixed into organic acids, which are stored until daylight, when they are utilized in the light-dependent reactions of photosynthesis.
- Classification Based on Cellular Structure:
- Prokaryotic Photosynthesis: Executed by organisms without a defined nucleus, such as certain bacteria.
- Eukaryotic Photosynthesis: Conducted by organisms with a well-defined nucleus, including protists and green plants.
- Classification Based on Oxygen Evolution:
- Oxygenic Photosynthesis:
- Organisms: Cyanobacteria (prokaryotic) and protists like diatoms, dinoflagellates, and Euglena (eukaryotic). Additionally, green plants, spanning from algae to angiosperms, employ this pathway.
- Characteristic: Oxygen is evolved as a byproduct.
- Anoxygenic Photosynthesis:
- Organisms: Exclusively prokaryotic, including green sulfur bacteria and purple bacteria.
- Characteristic: Oxygen is not released during the process.
- Oxygenic Photosynthesis:
In summary, photosynthesis, though universally critical for life on Earth, manifests in a myriad of forms, each tailored to the unique needs and environments of the photosynthesizing organisms.
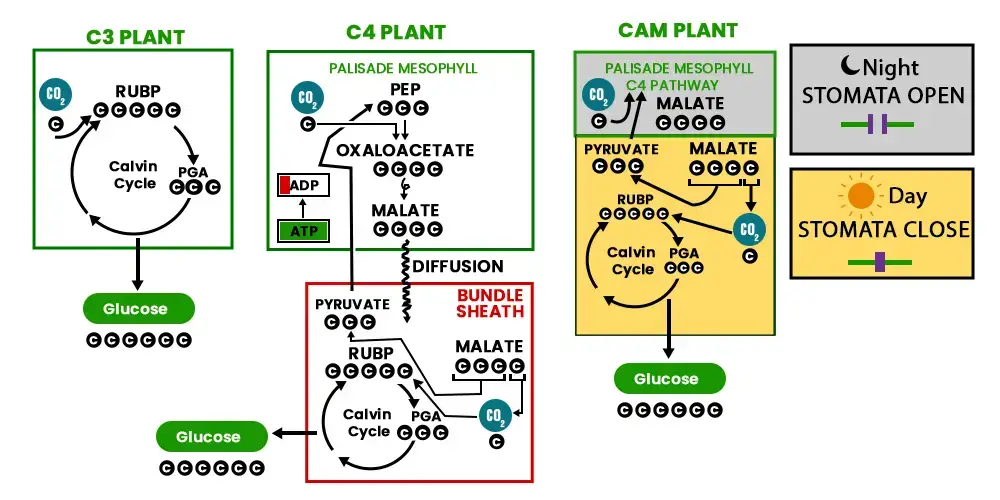
Photosynthetic pigments
Photosynthetic pigments are specialized molecules that absorb and harness light energy, facilitating the conversion of this energy into chemical energy during the process of photosynthesis. These pigments are pivotal for the efficient absorption of light across various wavelengths.
1. Chlorophyll: Chlorophyll, predominantly green in color, is the primary pigment responsible for capturing light energy. Its salient features include:
- Nature: It is a lipid-based molecule.
- Location: Predominantly found within the thylakoid membranes of chloroplasts.
- Types: Several variants exist, including chlorophyll-a, b, c, and d. Among these, chlorophyll-a is the primary photosynthetic pigment.
- Structure: Chlorophyll-a, b, and d are derivatives of “chlorin,” while chlorophyll-c is a “porphyrin” derivative. A unique feature is the presence of a central magnesium ion (Mg^2+).
- Function: Chlorophyll is instrumental in capturing and storing solar energy, and it plays a pivotal role in the photochemical reactions of photosynthesis.
2. Carotenoids: Carotenoids, often yellow, red, or purple, work synergistically with chlorophyll. Their key characteristics are:
- Nature: These are lipid-soluble molecules.
- Types: Over 150 variants of carotenoids have been identified.
- Forms: They exist as simple hydrocarbons (e.g., beta-carotene) or oxygenated hydrocarbons (e.g., lutein).
- Function: Carotenoids assist in energy transfer, act as free-radical scavengers, and provide photoprotection by quenching excess energy.
3. Phycobilins: Distinct from chlorophyll and carotenoids, phycobilins are present in specific oxygenic photosynthetic organisms, particularly cyanobacteria and red algae.
- Types: Notable variants include Phycoerythrobilin, Phycocyanobilins, and Allophycocyanobilins.
- Structure: These pigments possess a tetrapyrrole structure and do not require magnesium ions.
- Location: Being water-soluble, phycobilins, in conjunction with proteins, form phycobiliproteins. These proteins aggregate into clusters known as phycobilisomes, which adhere to membranes.
- Function: Phycobilins are primarily involved in resonance energy transfer.
4. Bacteriorhodopsin: Exclusive to halobacteria, bacteriorhodopsin is a pigment that consists of a protein linked to a retinal prosthetic group. It plays a role in light absorption, leading to proton expulsion from the cell.
In summary, photosynthetic pigments are integral to the process of photosynthesis, ensuring efficient light absorption and energy conversion. Each pigment type has a distinct role and absorption spectrum, collectively ensuring that a broad range of light wavelengths is harnessed for photosynthesis.
Structure Of Chlorophyll
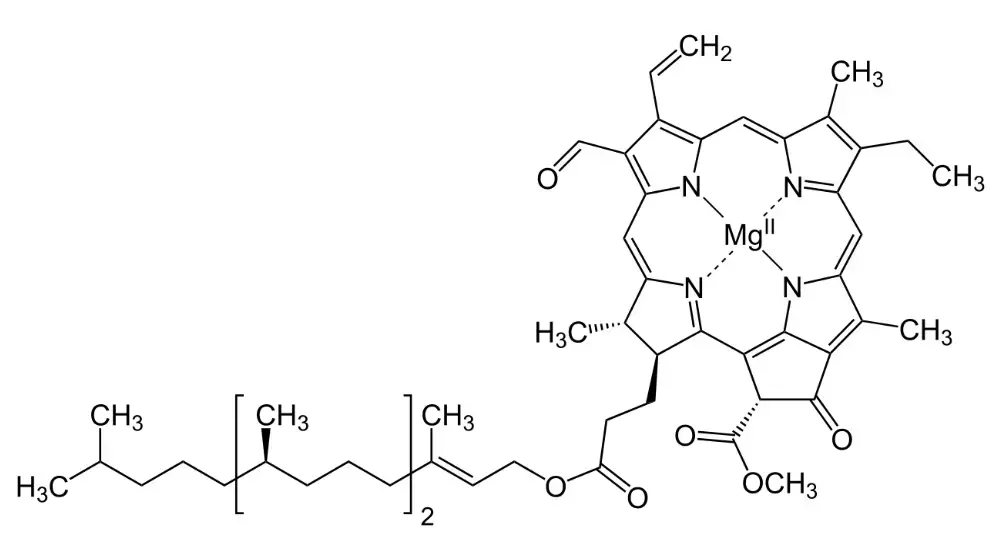
Chlorophyll, the quintessential green pigment in plants, possesses a unique molecular structure that facilitates its primary function: the absorption of sunlight for photosynthesis.
- Central Composition: At the core of chlorophyll’s structure lies a magnesium atom, encircled by four nitrogen atoms. This configuration is pivotal for its light-absorbing properties.
- Hydrocarbon Tail: Attached to this core is a hydrocarbon tail, which anchors the molecule to the thylakoid membrane within the chloroplasts.
- Chlorophyll Variants: While chlorophyll-a is the most prevalent type and plays a direct role in photosynthesis, there are other variants, including:
- Chlorophyll-b: This assists chlorophyll-a in capturing light energy and is found alongside it in many plants.
- Chlorophyll-c1 and c2: Typically found in certain algae species.
- Chlorophyll-d: Present in some cyanobacteria, it absorbs energy from different wavelengths of light compared to chlorophyll-a.
- Chlorophyll-f: Unique for its ability to absorb near-infrared light, making it more efficient than chlorophyll-a in certain conditions.
- Distribution: While green plants predominantly contain chlorophyll-a and chlorophyll-b, other photosynthesizing organisms, like certain algae and cyanobacteria, have the other variants, allowing them to optimize light absorption in diverse environments.
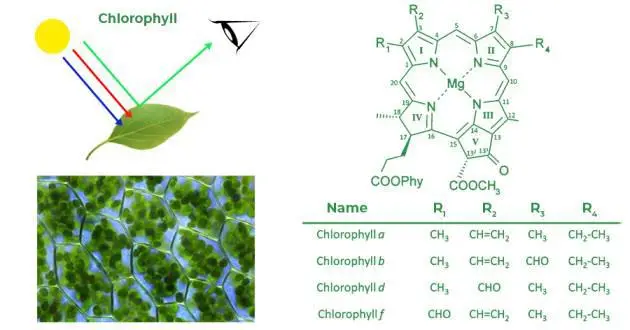
In essence, the structure of chlorophyll, with its central magnesium atom and various molecular variants, is ingeniously designed to maximize the absorption of sunlight, driving the life-sustaining process of photosynthesis across diverse organisms.
Factors affecting photosynthesis
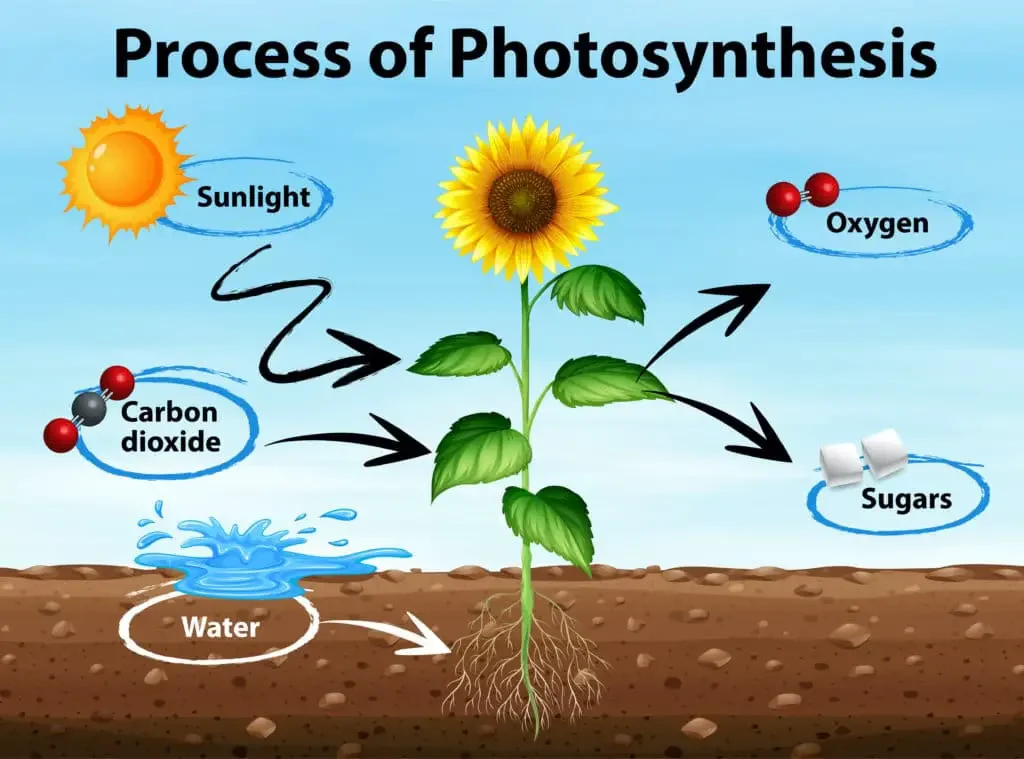
Photosynthesis, the process by which green plants and certain other organisms transform light energy into chemical energy, is influenced by a myriad of both external and internal factors. A comprehensive understanding of these factors is pivotal for optimizing the photosynthetic efficiency of plants.
1. Light Intensity, Quality, and Duration:
- Intensity: The rate of photosynthesis is directly proportional to light intensity up to a certain point. Beyond this threshold, the process plateaus, indicating the saturation of photosynthetic machinery. Plants have evolved into two categories based on their light intensity preference:
- Sciophytes: Flourish under diffused light conditions. (e.g., Oxalis)
- Heliophytes: Thrive under direct sunlight. (e.g., Dalbergia)
- Quality: Photosynthetically Active Radiation (PAR) denotes the fraction of light that actively participates in photosynthesis, typically ranging between 400-700nm in wavelength.
- Duration: While the duration of light exposure doesn’t directly alter the rate of photosynthesis, it does influence the total photosynthetic output.
2. Temperature:
Temperature plays a pivotal role in enzymatic activities associated with photosynthesis. For C3 plants, the optimal temperature range lies between 20-25°C, while C4 plants exhibit peak efficiency between 30-45°C. Beyond these ranges, enzymatic activities can diminish, potentially hampering the photosynthetic rate.
3. Carbon Dioxide Concentration:
Carbon dioxide (CO2) is a primary substrate in the photosynthetic process. An increase in its concentration can enhance the photosynthetic rate in C3 plants. However, C4 plants, equipped with a CO2 enrichment mechanism, exhibit a plateau in their photosynthetic rate even with elevated CO2 levels. The CO2 Compensation Point represents a threshold where the illuminated plant part ceases to absorb CO2.
4. Water Availability:
Water is indispensable for photosynthesis, participating in both light-dependent and light-independent reactions. A deficiency can impede the electron flow in Photosystem II and disrupt the Calvin cycle, thereby affecting the overall photosynthetic efficiency.
5. Genetic Factors:
Intrinsic genetic factors can also influence photosynthesis. Variations in genes encoding for photosynthetic machinery can lead to differences in their efficiency and functionality. Some species have evolved unique mechanisms to enhance photosynthetic efficiency, adapting to their specific environmental conditions.
In conclusion, photosynthesis is a multifaceted process influenced by a confluence of environmental and genetic factors. Understanding these factors and their interplay can provide insights into optimizing photosynthetic efficiency, with potential implications for agriculture and bioenergy production.
Photosynthetic Membranes and Organelles
Photosynthesis, the fundamental process by which energy from sunlight is converted into chemical energy, is facilitated by specific pigment molecules that absorb photons of light. The efficiency of this process is contingent upon the absorption of light within a precise wavelength range, ensuring the optimal energy required for photosynthesis.
To achieve this specificity in light absorption, phototrophic organisms have evolved specialized structures known as reaction center proteins. These proteins house the pigment molecules and are strategically positioned within the membranes of the organisms to optimize light absorption.
- Prokaryotic Photosynthetic Organisms:
- In prokaryotes, the photosynthetic machinery is embedded directly within the cell or thylakoid membranes present in the cytosol.
- Unlike eukaryotes, prokaryotes lack specialized organelles like chloroplasts. Instead, their photosystems are integrated within the cellular architecture, ensuring efficient light capture and energy conversion.
- Eukaryotic Photosynthetic Organisms (e.g., Green Plants):
- Eukaryotic organisms, such as green plants, possess specialized organelles known as chloroplasts. These chloroplasts are the hubs of photosynthetic activity.
- Within the chloroplasts, the thylakoid membranes house the photosystems. These membranes are laden with chlorophyll and other pigments, facilitating the absorption of light and the subsequent conversion of this energy into chemical forms.
In essence, the spatial organization of pigment molecules within specific membranes and organelles is a testament to the evolutionary adaptations of organisms to harness light energy efficiently. This intricate arrangement ensures that photosynthesis proceeds optimally, sustaining life on Earth.
Organelle for Photosynthesis
- The chloroplast is a pivotal organelle in eukaryotic cells, serving as the primary site for photosynthesis. Eukaryotic organisms, which encompass a diverse range of life forms, rely on chloroplasts to harness light energy and convert it into chemical energy.
- A typical plant cell is endowed with approximately 10 to 100 chloroplasts, underscoring their significance in the photosynthetic process. Delving deeper into the structure of the chloroplast, one encounters the thylakoids. These are specialized, membrane-bound compartments housed within the chloroplast.
- The thylakoid is not exclusive to eukaryotic chloroplasts; it is also found in the cytosol of cyanobacteria. However, it’s worth noting that while cyanobacteria possess thylakoids, they lack chloroplasts as a distinct organelle. The thylakoid is instrumental in the initial stages of photosynthesis, specifically the light-dependent or photochemical reactions.
- This compartment is intricately structured, comprising the membrane, lumen, and lamellae. Embedded within the thylakoid membranes are chlorophyll molecules, the green pigments responsible for capturing light energy.
- These molecules play a central role in the absorption and conversion of light energy, initiating the cascade of reactions that culminate in the synthesis of organic molecules. In summary, the chloroplast, with its resident thylakoids, stands as the cornerstone of photosynthesis in eukaryotic organisms, orchestrating the intricate processes that transform light into life-sustaining energy.
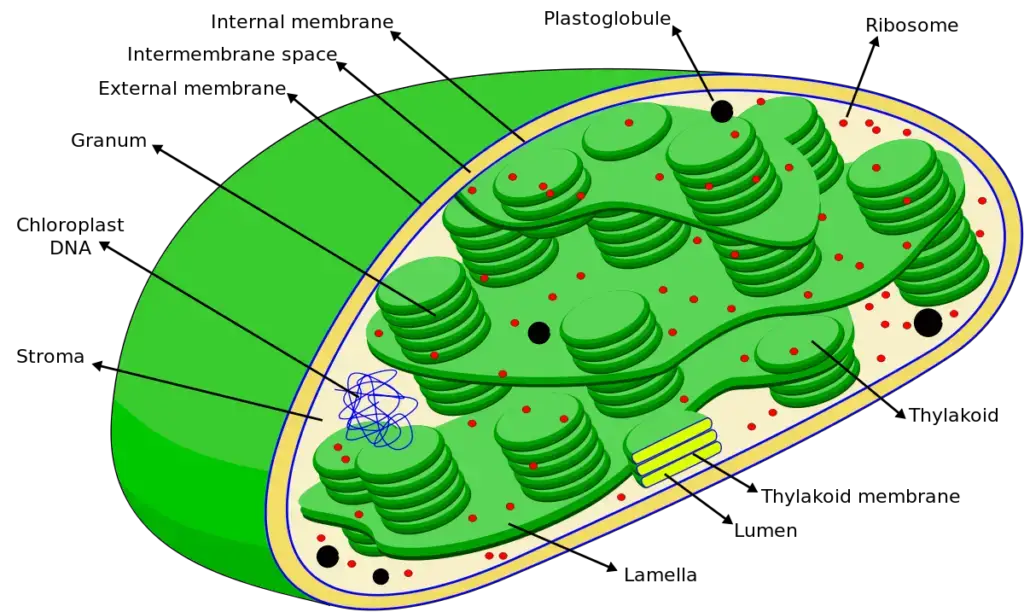
Process/ Steps of Photosynthesis – Mechanisms of Photosynthesis
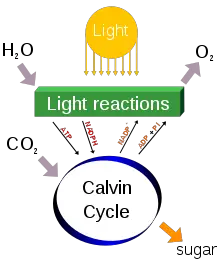
Photosynthesis, a cornerstone of plant physiology, is a systematic process that facilitates the conversion of light energy into chemical energy stored in organic molecules. This intricate mechanism can be elucidated through the following sequential stages:
- Light Absorption: Within the chloroplasts, thylakoid membranes house chlorophyll molecules and other pigments. These molecules are adept at capturing light energy. Once absorbed, this energy facilitates the extraction of electrons from a donor, typically water. This electron removal results in the formation of oxygen. The primary electron acceptor in this phase is quinine (Q), akin to CoQ present in the electron transport chain.
- Electron Transport: Post the initial absorption, the electrons are relayed from the primary electron acceptor to a series of molecular intermediaries located within the thylakoid membrane. The culmination of this transfer sees the electrons being accepted by NADP+, the terminal electron acceptor. Concurrently, as electrons traverse the membrane, protons are actively extricated, engendering a proton gradient across the membrane.
- ATP Synthesis: Leveraging the established proton gradient, protons traverse from the thylakoid lumen back to the stroma via the F0F1 complex. This movement is instrumental in the phosphorylation of ADP, resulting in the generation of ATP. This phase mirrors the ATP synthesis observed in the mitochondrial electron transport chain.
- Carbon Reduction: With the energy and electrons furnished by NADP and ATP from the preceding steps, carbon dioxide undergoes a reduction process, culminating in the formation of six-carbon sugar molecules. It’s imperative to note that while the initial three stages are contingent on light, rendering them “light reactions”, the carbon reduction step operates independently of light, earning it the designation of “dark reactions”.
In summation, photosynthesis is a meticulously orchestrated sequence of events, harmonizing light-dependent and light-independent reactions to produce organic compounds vital for plant sustenance and growth.
Types/ Stages/ Parts of photosynthesis
Photosynthesis can be split into two stages that are based on the use of light energy
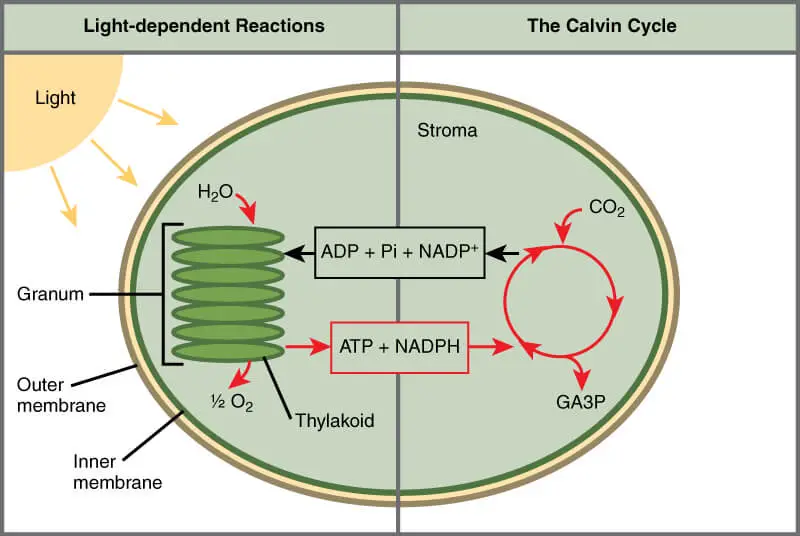
1. Light-dependent reactions
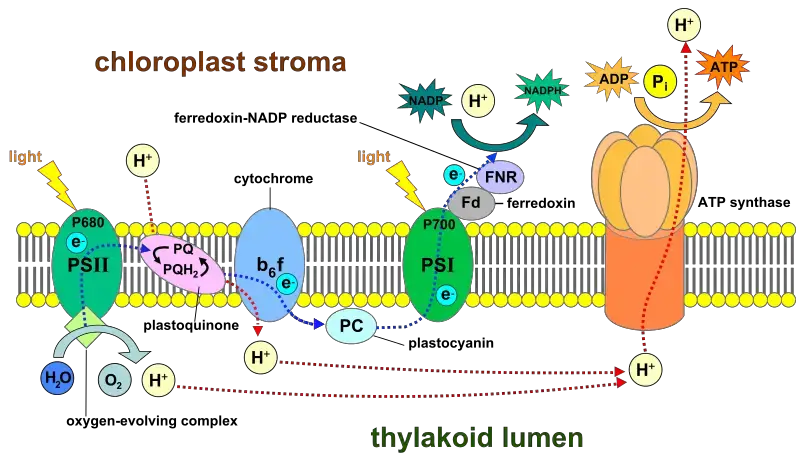
Photosynthesis, a pivotal biochemical process, encompasses a series of reactions that convert light energy into chemical energy. The light-dependent reactions, as the name suggests, are contingent on the presence of light and primarily transpire within the thylakoid membranes of chloroplasts. These reactions can be systematically delineated into the following stages:
1. Photon Absorption and Light Harvesting
The initial phase involves the capture of photons by chlorophyll molecules, accessory pigments, and associated proteins, collectively termed photoreceptors. These photoreceptors are organized into intricate assemblies, comprising a photosynthetic reaction center, core antenna complexes, and light-harvesting complexes (LHC). The core antenna and LHC, constituted by accessory pigments and chlorophyll-bound proteins, function as photon traps, capturing diverse wavelengths of light. Sequentially, the absorbed photon energy is channeled towards the photosynthetic reaction center.
Two distinct photosynthetic reaction centers are present in plants, green algae, and cyanobacteria:
- Photosystem I (PSI): Primarily utilizing chlorophyll a, PSI is excited by light with a peak wavelength of 700 nanometers (P700).
- Photosystem II (PSII): Incorporating both chlorophyll a and b, PSII can absorb light up to a wavelength of 680 nanometers (P680).
2. Electron Transport Dynamics:
Photon absorption culminates in the elevation of an electron to a heightened energy state, engendering a negatively charged radical. This unstable state prompts the electron’s spontaneous transfer to a proximate acceptor molecule, rendering the photoreceptor positively charged. Subsequent replenishment of the photoreceptor is achieved through electrons derived either from water or another electron transfer chain.
Electron transfer ensues through a series of complexes:
- PSI (Plastocyanin-Ferredoxin Oxidoreductase): Here, the excited electron from P700 is relayed to plastocyanin (PC), which subsequently donates the electron to ferredoxin (Fd). Concurrently, NADP+ is reduced to NADPH in the stroma.
- PSII: The excited P680 electron is sequentially transferred to pheophytin and plastoquinone. The resultant plastoquinol diffuses to the Cytochrome b6f complex. The oxygen-evolving complex (OEC) facilitates the replenishment of P680 by catalyzing water oxidation, releasing oxygen and protons.
- Cytochrome b6f Complex: Serving as a bridge between the photosystems, this complex facilitates linear electron flow, adhering to the Z scheme. The electron transfer is concomitant with proton translocation, engendering a proton gradient vital for ATP synthesis.
3. Photophosphorylation:
Two pathways exist for ATP synthesis:
- Z Scheme Pathway (Non-Cyclic Photophosphorylation): The proton gradient established during electron transfer drives ATP synthesis via ATP-H+ synthase, converting ADP to ATP.
- Cyclic Photophosphorylation: Here, electron flow is circumscribed between PSI and the Cytochrome b6f complex. The resultant proton gradient similarly facilitates ATP synthesis.
In essence, light-dependent reactions are a harmonious interplay of photon absorption, electron transport, and ATP synthesis, laying the foundation for the subsequent light-independent reactions of photosynthesis.
Wavelengths of light involved and their absorption
Light, a fundamental driver of photosynthesis, is composed of a spectrum of wavelengths, each with its distinct properties. The entirety of this spectrum, spanning from 390 to 760 nanometers (nm), constitutes the visible light spectrum perceivable by the human eye. However, not all these wavelengths are equivalently harnessed by photosynthetic organisms.
Within the broad spectrum of visible light lies a critical subset termed PAR, or Photosynthetically Active Radiation. This segment, ranging from 400 to 760 nm, is of paramount importance to photosynthetic processes. Within PAR, specific wavelengths play distinct roles:
- Blue Light (470-500 nm): This segment of the spectrum is actively absorbed and utilized by photosynthetic organisms. It plays a pivotal role in phototropic responses and chlorophyll production, thereby influencing plant growth and development.
- Red Light (660-760 nm): Another crucial segment for photosynthesis, red light is absorbed efficiently by chlorophyll pigments, driving the photosynthetic process.
- Green Light (500-580 nm): Contrary to the active absorption of blue and red lights, green light is predominantly reflected by plants. This reflection imparts the characteristic green hue to most plants. It’s worth noting that while the green light is majorly reflected, it is not entirely unused; a fraction is absorbed, albeit less efficiently than blue or red light.
- Blue-Green Light: While the spectrum does encompass blue-green wavelengths, it is the blue light, not the blue-green, that is harnessed for photosynthesis.
In summation, while the visible spectrum is vast, photosynthetic organisms exhibit selectivity in the wavelengths they absorb and utilize. This selective absorption and reflection not only drive the photosynthetic process but also influence the very appearance of plants in our environment.
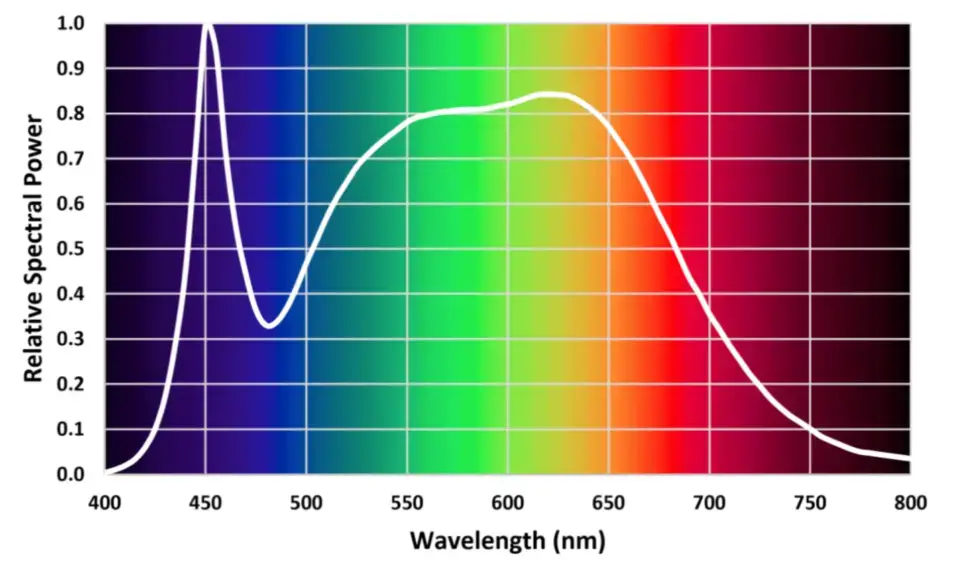
Absorption spectrum and action spectrum
In the realm of photosynthesis, understanding the interaction between light and pigments is paramount. Two critical spectra, the absorption spectrum and the action spectrum, provide insights into this interaction. These spectra elucidate the efficiency of different wavelengths in the photosynthetic process and the role of specific pigments.
- Absorption Spectrum:
- Definition: The absorption spectrum is a graphical representation that delineates the efficiency with which a specific pigment absorbs various wavelengths of light.
- Graphical Representation: On the X-axis, the graph plots the wavelengths of light (measured in nanometers/nm), while the Y-axis represents the percentage of light absorption by the pigment.
- Characteristics: Each pigment possesses a unique absorption spectrum, making it a signature representation of that pigment. For instance, chlorophyll-a and chlorophyll-b have distinct absorption peaks, indicating their preferential absorption of specific wavelengths.
- Example: Chlorophyll-a predominantly absorbs light at 430 nm (blue) and 660 nm (red), with a higher absorption efficiency at 660 nm. In contrast, chlorophyll-b exhibits optimal absorption at 430 nm (blue) and 660 nm (red), with a pronounced peak at 430 nm.
- Action Spectrum:
- Definition: The action spectrum offers a depiction of the effectiveness of different light wavelengths in instigating the photosynthetic process.
- Graphical Representation: The X-axis plots the wavelengths of light (in nanometers/nm), while the Y-axis showcases the rate of photosynthesis, typically quantified by the amount of oxygen released.
- Interrelation with Absorption Spectrum: Overlaying the action spectrum with the absorption spectrum of a pigment can elucidate the contribution of specific wavelengths to photosynthetic efficiency and productivity.
- Significance: While the absorption spectrum can be determined for any photosynthetic pigment, the action spectrum is specifically associated with the primary photochemical reaction-performing pigment, chlorophyll-a. This pigment is located at the reaction center, where the evolution of oxygen gas, indicative of photosynthetic activity, predominantly occurs. Given its direct link to the excitation of the chlorophyll-a molecule, the action spectrum is exclusively attributed to this pigment.
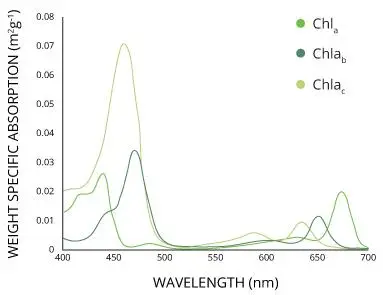
In summary, while the absorption spectrum provides insights into the light-absorbing capabilities of pigments, the action spectrum reveals the functional efficacy of these absorbed wavelengths in driving photosynthesis. Together, these spectra offer a comprehensive understanding of the intricate interplay between light and photosynthetic pigments.
What actually happens in the Light-dependent reaction
The light-dependent reaction is a pivotal phase in photosynthesis, transpiring within the chloroplasts. This process is initiated by the absorption of light energy and culminates in the synthesis of ATP and NADPH, which are quintessential for the subsequent light-independent reactions. Here’s a detailed elucidation of the events that transpire during the light-dependent reaction:
- Photon Absorption and Electron Excitation:
- Upon the absorption of a photon by a chlorophyll molecule, specifically P680, the molecule becomes photoexcited and releases an electron.
- This event marks the commencement of the photochemical electron flow.
- Electron Transfer Sequence:
- The excited electron is initially relayed to the D1/D2 protein complex.
- Subsequently, it is transferred to a modified chlorophyll variant and then to “pheophytin.”
- The electron journey continues as it moves to plastoquinone A and eventually to plastoquinone B.
- Significance of the Electron Flow:
- The orchestrated electron flow sets in motion an electron transport chain, which plays a pivotal role in the light-dependent reactions.
- One of the primary outcomes of this electron flow is the reduction of NADP to NADPH. This molecule, NADPH, is instrumental in the subsequent light-independent reactions of photosynthesis.
- Concurrently, the electron flow engenders a proton gradient across the chloroplast membrane.
- ATP Synthesis:
- The established proton gradient is harnessed by the ATP synthase enzyme.
- Utilizing this gradient, ATP synthase facilitates the phosphorylation of ADP to generate ATP.
- The synthesized ATP serves as an energy reservoir for the ensuing light-independent reactions.
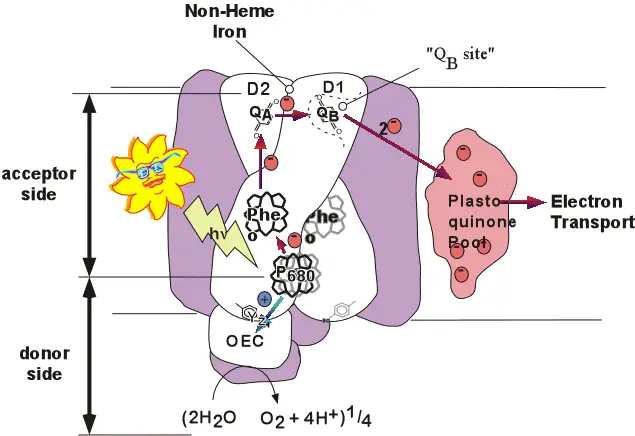
In essence, the light-dependent reaction is a meticulously orchestrated sequence of events that harnesses light energy to produce ATP and NADPH. These molecules are indispensable for the sustenance of the photosynthetic process and the plant’s overall energy requirements.
Water photolysis
Water photolysis, also known as the oxygen-evolving process, is a fundamental mechanism within the photosynthetic pathway. This process is responsible for replenishing the electron deficit experienced by the chlorophyll molecule during the initial stages of photosynthesis. Here’s a comprehensive overview of water photolysis:
- Electron Replenishment:
- During photosynthesis, the chlorophyll molecule loses an electron upon photon absorption. To sustain the continuity of the photosynthetic electron transport chain, this electron deficit must be addressed.
- The mechanism that compensates for this electron loss is the photolysis of water molecules, facilitated by the “oxygen-evolving complex” situated in the thylakoid membrane.
- Oxygen Evolution:
- As a consequence of water photolysis, oxygen is evolved. This process not only replenishes the lost electron to the chlorophyll molecule but also results in the liberation of oxygen gas, a byproduct of photosynthesis.
- Scientific Exploration:
- The origin of the oxygen released during photosynthesis was a subject of scientific debate. Initial hypotheses posited that the oxygen atom from CO2 might be the source of the evolved oxygen.
- However, through collaborative research, the mystery was unraveled. C.B. Van Niel, studying purple photosynthetic bacteria, provided indirect evidence suggesting that the oxygen source was water molecules, not CO2.
- This assertion was further corroborated by Ruben, Hassid, and Kamen, who employed isotopic studies to furnish direct evidence that the oxygen evolved during photosynthesis indeed originates from H2O molecules.
- Chemical Implication:
- The photolysis process involves the hydrolysis of two water molecules, culminating in the release of one molecule of oxygen gas. This can be represented by the following equation for the light-dependent reactions: 2H2O+2NADP++3ADP+3Pi+light→2NADPH+2H++3ATP+O2
In essence, water photolysis is a pivotal process in photosynthesis, ensuring the continuity of the electron transport chain and facilitating the release of oxygen, which is vital for aerobic life forms on Earth.
Z scheme
In the realm of photosynthesis, the photochemical reactions can be categorized into two primary types: cyclic and non-cyclic reactions. These reactions are distinguished by the involvement and flow of electrons through photosystems.
- Cyclic Reaction:
- This reaction involves solely Photosystem I (PS1).
- Upon photon absorption, the P700 chlorophyll molecule in PS1 becomes excited. The excited electron then traverses a sequence of molecules: from Fe-S to Ferredoxin, followed by Plastoquinone, the Cytochrome b6f complex, and finally to Plastocyanin.
- Given the exclusive involvement of PS1, the electron flow forms a loop, rendering it cyclic. This process culminates in what is termed cyclic phosphorylation.
- This reaction predominantly occurs in the stroma lamellae, especially when light with a wavelength exceeding 680nm is available.
- Non-Cyclic Reaction (Z-Scheme):
- This reaction encompasses both Photosystem I (PS1) and Photosystem II (PS2).
- In PS2, the absorption of a photon excites the P680 chlorophyll molecule. This excitement leads to the loss of an electron, which is subsequently transferred to pheophytin.
- The electron then embarks on a unique trajectory, often visualized as a zigzag or ‘Z’ pattern, hence the name “Z-Scheme.”
- Within this Z-Scheme, the electron undergoes a series of redox reactions, culminating in the reduction of NADP+ to NADPH.
- Concurrently, the chemiosmotic potential is established by pumping protons across the membrane into the thylakoid lumen. This gradient drives the synthesis of ATP.
In summary, the Z-Scheme is a crucial component of the non-cyclic photochemical reactions in photosynthesis. It delineates the intricate electron flow through both photosystems, facilitating the production of essential energy molecules, NADPH and ATP, vital for the subsequent stages of photosynthesis.
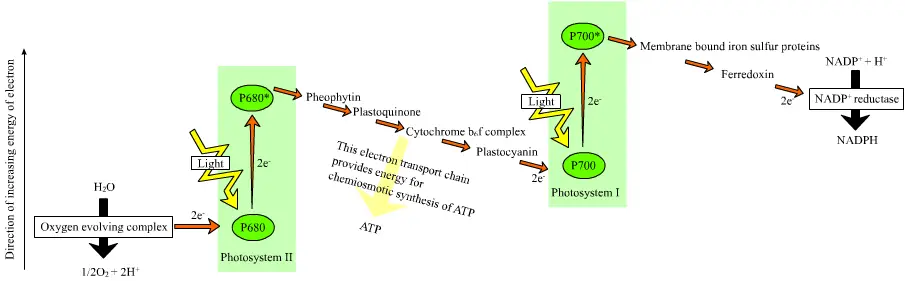
Cyclic vs. Non-cyclic phosphorylation
In the intricate process of photosynthesis, photochemical reactions play a pivotal role. These reactions can be broadly categorized into cyclic and non-cyclic phosphorylation. Each type has distinct characteristics and functions. Here’s a detailed comparison between the two:
Feature | Cyclic Phosphorylation | Non-cyclic Phosphorylation |
---|---|---|
Oxidation of Water | Absent | Present |
Generation of Oxygen Gas | Absent | Present |
Reduction of NADP | Absent | Present |
Requirement for Final Electron Donor/Acceptor | Absent | Present (Donor: H2O, Acceptor: NADP+) |
ATP Production | Present | Present |
Photosystems Involved | Only PS1 | Both PS1 and PS2 |
Key Insights:
- Cyclic Phosphorylation: This process involves only Photosystem I (PS1). As the name suggests, the electron flow is cyclic, meaning the electrons return to their original position after completing the cycle. Notably, this process does not lead to the oxidation of water or the generation of oxygen gas. Its primary function is to produce ATP.
- Non-cyclic Phosphorylation: This is a more complex process involving both Photosystem I (PS1) and Photosystem II (PS2). Here, electrons are transferred from water molecules to NADP+, resulting in the production of NADPH. This process leads to the oxidation of water, releasing oxygen gas as a byproduct. Both ATP and NADPH are produced, which are essential for the subsequent stages of photosynthesis.
In essence, while both cyclic and non-cyclic phosphorylation contribute to the production of ATP, only non-cyclic phosphorylation results in the generation of NADPH and oxygen gas, making it integral to the overall photosynthetic process.
2. Light independent reactions (Calvin cycle)
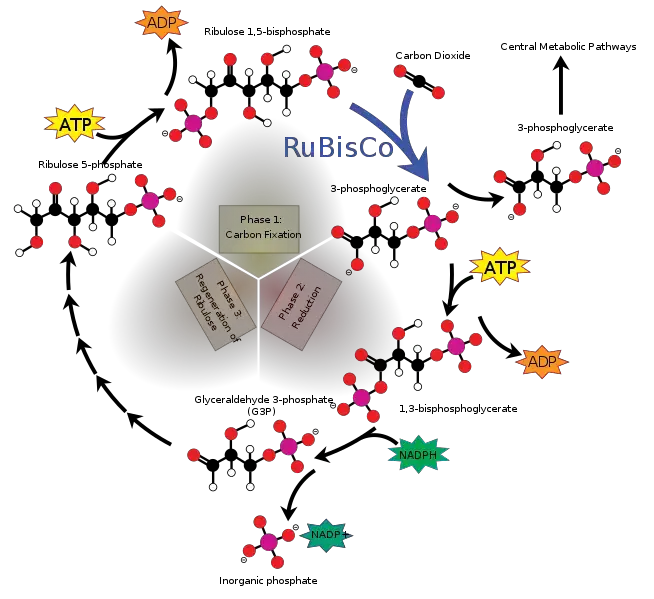
The Calvin Cycle, also known as the light-independent reactions, is a crucial phase of photosynthesis that operates in the stroma of chloroplasts. Contrary to its name, this cycle doesn’t directly rely on light; however, it is dependent on the ATP and NADPH produced during the light-dependent reactions.
- Carbon Fixation: The cycle commences with the fixation of a single carbon dioxide molecule to a five-carbon sugar called ribulose 1,5-bisphosphate. This reaction is facilitated by the enzyme ribulose 1,5-bisphosphate carboxylase, commonly referred to as rubisco. The immediate product is an unstable six-carbon compound, which rapidly splits into two molecules of 3-phosphoglycerate.
- Reduction Phase: The 3-phosphoglycerate molecules undergo a series of enzymatic reactions. Initially, ATP donates a phosphate group to 3-phosphoglycerate, forming 1,3-bisphosphoglycerate. Subsequently, NADPH provides electrons, converting 1,3-bisphosphoglycerate into glyceraldehyde 3-phosphate. While a portion of the produced glyceraldehyde 3-phosphate contributes to the synthesis of glucose and other sugars, the majority is utilized to regenerate ribulose 1,5-bisphosphate.
- Regeneration of Ribulose 1,5-bisphosphate: The remaining glyceraldehyde 3-phosphate undergoes a complex series of enzymatic reactions, resulting in the production of ribulose 1,5-bisphosphate. This regeneration ensures the continuity of the Calvin Cycle.
Overall Reaction: 3CO2+9ATP+6NADPH+6H+→glyceraldehyde−3−phosphate(G3P)+9ADP+8Pi+6NADP++3H2O
To synthesize one molecule of glucose, which contains six carbon atoms, the Calvin Cycle must operate six times, fixing six molecules of carbon dioxide.
In essence, the Calvin Cycle is a metabolic pathway that transforms carbon dioxide and other compounds into glucose, providing energy and structural integrity to plants. This intricate process underscores the importance of both light-dependent and light-independent reactions in sustaining life on Earth.
Order and kinetics of Photosynthesis
Photosynthesis, a fundamental biological process, is an intricate sequence of events that culminates in the conversion of light energy into chemical energy in the form of glucose. Understanding the order and kinetics of photosynthesis is crucial to unraveling the mechanisms that drive this essential phenomenon. This informative discourse provides insight into the sequential stages and time scales involved in the photosynthetic process, as outlined below.
Stage 1: Energy Transfer in Antenna Chlorophyll (Thylakoid Membranes)
The inception of photosynthesis begins with the absorption of light energy by chlorophyll and other pigments located in the antenna complex of thylakoid membranes. This critical step occurs with astonishing rapidity, unfolding on the femtosecond to picosecond timescale. During this minuscule time frame, the excited electrons within the chlorophyll molecules undergo rapid energy transfer, initiating the photosynthetic cascade.
Stage 2: Transfer of Electrons in Photochemical Reactions (Thylakoid Membranes)
Following energy absorption, the second stage encompasses the transfer of these energized electrons through a series of photochemical reactions within the thylakoid membranes. This process operates at a slightly longer timescale, ranging from picoseconds to nanoseconds. As electrons shuttle through protein complexes, such as Photosystem II and Photosystem I, they undergo redox reactions that ultimately result in the generation of ATP and the reduction of NADP+ to NADPH.
Stage 3: Electron Transport Chain and ATP Synthesis (Thylakoid Membranes)
The third phase of photosynthesis involves the electron transport chain, which propels electrons through a series of membrane-bound protein complexes. This intricate molecular machinery operates on a timescale spanning from microseconds to milliseconds. As electrons flow through the transport chain, protons are pumped across the thylakoid membrane, establishing a proton gradient that fuels the synthesis of ATP via chemiosmotic coupling. This stage is pivotal in harnessing the energy from light and converting it into a biologically useful form.
Stage 4: Carbon Fixation and Export of Stable Products
The culmination of photosynthesis transpires in the fourth stage, where the acquired ATP and NADPH molecules, along with carbon dioxide, are utilized for the fixation of carbon into organic compounds. This final, but no less intricate, phase operates on a timescale ranging from milliseconds to seconds. The Calvin-Benson cycle, a series of enzyme-catalyzed reactions, assimilates carbon dioxide and generates sugars, primarily glucose, which serve as the ultimate product of photosynthesis. These stable products are subsequently exported to various parts of the plant for energy storage and growth.
In conclusion, the order and kinetics of photosynthesis encompass a meticulously orchestrated sequence of events, spanning a wide range of time scales from femtoseconds to seconds. This process showcases the remarkable efficiency of nature in harnessing light energy to sustain life on Earth, underlining its significance in the realm of biological science.
Carbon Concentrating Mechanisms in Photosynthesis
In the realm of photosynthesis, carbon concentrating mechanisms (CCMs) play a pivotal role in optimizing the carbon fixation process, especially in environments with fluctuating carbon dioxide (CO2) levels. These mechanisms are primarily observed in certain angiosperm families and are categorized based on their operational strategies.
1. C4 Photosynthesis: The C4 pathway, also known as the Hatch and Slack cycle, is a specialized mechanism observed in approximately 4% of angiosperm families, notably Poaceae and Cyperaceae. It was elucidated by scientists Hatch and Slack, who studied its manifestation in maize plants.
- Mechanism: In C4 plants, photosynthesis is spatially separated into two cell types: mesophyll cells and bundle sheath cells. The initial CO2 fixation occurs in the mesophyll cells, where CO2 is combined with phosphoenolpyruvate (PEP) by the enzyme PEP carboxylase, producing a four-carbon compound, oxaloacetic acid or malate. This compound is then transported to the bundle sheath cells, where it undergoes decarboxylation, releasing CO2. This CO2 is subsequently fixed into glucose through the conventional C3 cycle.
- Significance: The C4 mechanism is an adaptive strategy for plants in semi-arid regions. Under high temperatures and light conditions, these plants can efficiently fix CO2, even when stomata are partially closed to minimize water loss. This spatial separation reduces the oxygenase activity of RuBisCo, thereby decreasing photorespiration and enhancing carbon fixation.
2. CAM Photosynthesis: Crassulacean Acid Metabolism (CAM) is another carbon concentrating mechanism predominantly found in xerophytes like cacti and succulents, with around 16,000 species employing this strategy.
- Mechanism: Unlike C4 plants that exhibit spatial separation, CAM plants demonstrate a temporal separation of CO2 uptake and fixation. During the night, when the stomata are open, CO2 is fixed into malate by PEP carboxylase. During the day, when the stomata are closed, malate is decarboxylated to release CO2, which is then fixed through the C3 cycle.
- Significance: This mechanism allows CAM plants to efficiently conduct photosynthesis under water-limited conditions, as they can take up CO2 during cooler nights and minimize water loss during the day.
Aquatic Adaptations: In aquatic environments, cyanobacteria exhibit a unique carbon concentrating mechanism. They possess specialized structures called carboxysomes, which enrich the CO2 concentration around the RuBisCo enzyme. The enzyme carbonic anhydrase within these carboxysomes can release CO2 from dissolved bicarbonate ions, ensuring efficient carbon fixation.
In summary, carbon concentrating mechanisms are adaptive strategies that enhance the efficiency of photosynthesis under varying environmental conditions. Whether through spatial or temporal separation, or specialized cellular structures, these mechanisms ensure optimal carbon fixation, catering to the diverse needs of plants across different habitats.
Regulation of the cycle
Photosynthesis is not possible in the night, however, glycolysis, a process that utilizes the same reactions as those in the Calvin-Benson cycles, with the exception of the reverse, takes place. This means that certain steps in the cycle would be inefficient when they are allowed to take place in darkness, as they could impede the process of glycolysis. In this regard, certain enzymes in the Calvin-Benson cycle can be “turned off” (i.e. they become inactive) in darkness.
In the absence of sunlight, changes in physiological conditions often require adjustments to the rate of reaction in the Calvin-Benson cycle such that enzymes that are involved in some reactions alter their catalytic activities. These changes in enzyme activity are typically caused through changes in the levels of chloroplast components such as reduced ferredoxin and acids and the soluble components (e.g., Pi and magnesium ions).
Products of Photosynthesis
Photosynthesis, the fundamental process by which plants convert light energy into chemical energy, yields a variety of essential products that sustain life on Earth. These products can be categorized based on the specific reactions involved:
- Light-Dependent Reactions:
- ATP (Adenosine Triphosphate): A primary energy currency of cells, facilitating energy transfer for various cellular processes.
- NADPH (Nicotinamide Adenine Dinucleotide Phosphate): A coenzyme that carries electrons, crucial for the synthesis of organic molecules.
- Oxygen (O2): Released into the atmosphere as a byproduct when water molecules are split.
- Protons (H+ ions): These play a role in creating a proton gradient across the thylakoid membrane, driving ATP synthesis.
- Light-Independent Reactions (Calvin Cycle):
- Glyceraldehyde-3-Phosphate (G3P): A three-carbon sugar molecule, which is a precursor to glucose and other carbohydrates.
- Protons (H+ ions): Involved in the reduction of 3-phosphoglycerate to G3P.
- Overall Products of Photosynthesis:
- Glucose (Carbohydrates): The primary energy storage molecule, which can be further converted into other organic compounds or used for energy by organisms.
- Water (H2O): Some water molecules are produced during the Calvin cycle.
- Oxygen (O2): A vital byproduct released into the atmosphere, supporting aerobic respiration in organisms.
- Sulfur: Specifically produced in photosynthetic sulfur bacteria, contributing to the sulfur cycle in ecosystems.
These products, especially glucose and oxygen, are fundamental to the survival and growth of most organisms on Earth.
Process of Photosynthesis – Overview
Photosynthesis, a vital physiological process, transpires within specialized cellular structures termed chloroplasts. These organelles house chlorophyll, the green pigment responsible for the characteristic hue of plant leaves. The leaf’s lamina facilitates the absorption of both sunlight and carbon dioxide, essential components for photosynthesis.
This intricate process can be delineated into two primary phases based on light dependency:
- Light-Dependent Reactions:
- Temporal Occurrence: As the name suggests, these reactions are contingent upon sunlight and predominantly occur during daylight hours.
- Location: The thylakoid membrane, within the chloroplast, is the site for these reactions.
- Grana: Situated inside the thylakoid, grana are sac-like structures that capture and store light.
- Photosystems: Integral membrane proteins, termed photosystems, play a pivotal role in this phase. There are two primary types:
- Photosystem-I (PS-I)
- Photosystem-II (PS-II)
- Electron Excitation: Upon photon absorption by chlorophyll within the reaction center, an electron is excited and subsequently released.
- Energy Conversion: This phase culminates in the conversion of solar energy into chemical energy, yielding ATP and NADPH.
- Chemical Representation: 2H2O + 2NADP+ + 3 ADP + 3Pi → O2 + 2NADPH + 3ATP
- Light-Independent Reactions (Calvin Cycle):
- Alternative Names: Often referred to as the dark reaction or carbon fixation.
- Location: These reactions transpire within the stroma of the chloroplast.
- Carbon Dioxide Uptake: Plants assimilate carbon dioxide via stomata, initiating the Calvin cycle.
- Sugar Formation: Through the Calvin cycle, six carbon dioxide molecules are utilized to synthesize a single sugar molecule.
- Chemical Representation: 3CO2 + 6 NADPH + 5H2O + 9ATP → G3P + 2H+ + 6NADP+ + 9ADP + 8Pi
Light-dependent reactions vs. light-independent reactions
Photosynthesis, the process by which plants convert light energy into chemical energy, is delineated into two primary phases: the light-dependent reactions and the light-independent reactions. Each phase plays a distinct role in the overall process, and they are characterized by their dependency on light and their respective locations within the chloroplast.
1. Light-Dependent Reactions:
- Location: These reactions transpire in the thylakoid membrane of the chloroplast.
- Dependency on Light: As the name suggests, light-dependent reactions necessitate the direct absorption of sunlight.
- Mechanism: Within the thylakoid membrane, chlorophyll and other pigments absorb photons from sunlight. This absorption triggers a series of electron transport chains, leading to the synthesis of energy-rich molecules, adenosine triphosphate (ATP) and nicotinamide adenine dinucleotide phosphate (NADPH).
- Outcome: The primary products of light-dependent reactions are ATP and NADPH, which store the energy harnessed from sunlight in chemical form.
2. Light-Independent Reactions (Calvin Cycle):
- Location: These reactions occur in the stroma, the fluid-filled space between the thylakoid membranes and the chloroplast envelope.
- Dependency on Light: Contrary to light-dependent reactions, the Calvin Cycle operates independently of direct light exposure, hence the designation “light-independent.”
- Mechanism: Utilizing the energy stored in ATP and NADPH, the Calvin Cycle facilitates the fixation of atmospheric carbon dioxide (CO2) into organic molecules. Through a series of enzymatic reactions, CO2 is converted into carbohydrate molecules, such as glucose.
- Outcome: The culmination of the Calvin Cycle is the synthesis of glucose and other carbohydrates, which serve as energy reservoirs for the plant and are crucial for growth and development.
Aspect | Light-Dependent Reactions | Light-Independent Reactions (Calvin Cycle) |
---|---|---|
Location | Thylakoid membrane | Stroma |
Dependency on Light | Requires sunlight | Operates independently of direct light |
Mechanism | Chlorophyll absorbs sunlight, triggering electron transport chains, leading to ATP and NADPH synthesis. | Uses ATP and NADPH to fix atmospheric CO2 into organic molecules, producing carbohydrates like glucose. |
Outcome | Production of ATP and NADPH | Synthesis of glucose and other carbohydrates |
Role in Photosynthesis | Energy capture and conversion | Carbon fixation and carbohydrate synthesis |
Photosynthesis examples
Photosynthesis, the process by which organisms convert light energy into chemical energy, manifests in various forms across different species. Here are specific examples:
- Photosynthesis in Green Plants and Oxygenic Bacteria:
- Organisms: Green plants and oxygenic bacteria, notably cyanobacteria.
- Pigment Involved: Chlorophyll, a green pigment, plays a pivotal role in capturing light energy.
- Location: This process occurs within the thylakoids of chloroplasts.
- Reactants: Carbon dioxide and water.
- Products: Oxygen gas, glucose, and water molecules. In plants, glucose units are often linked to form compounds like starch, fructose, or sucrose.
- Photosynthesis in Sulfur Bacteria:
- Organisms: Purple sulfur bacteria and green sulfur bacteria.
- Pigment Involved: While green sulfur bacteria utilize chlorophyll, purple sulfur bacteria predominantly use carotenoids as their photosynthetic pigments.
- Location: Within specialized structures in bacterial cells.
- Reactants: Carbon dioxide and hydrogen sulfide (H2S) serve as the primary reactants, replacing water.
- Products: Carbohydrates (which may not always be glucose), sulfur gas, and water molecules.
- Photosynthesis in Red Algae:
- Organisms: Red algae (Rhodophyta).
- Pigment Involved: Phycobiliproteins, especially phycoerythrin, give red algae its distinctive color and play a role in capturing light energy.
- Location: Within the chloroplasts of red algae cells.
- Reactants: Carbon dioxide and water.
- Products: Oxygen gas and carbohydrates. Red algae often produce floridean starch as their primary carbohydrate storage molecule.
- Photosynthesis in Diatoms:
- Organisms: Diatoms, a major group of microalgae found in oceans, waterways, and soils.
- Pigment Involved: Chlorophyll-a and chlorophyll-c, along with fucoxanthin, a brown pigment that gives diatoms their golden-brown color.
- Location: Within the chloroplasts of diatom cells, which are often encased in intricate silica shells.
- Reactants: Carbon dioxide and water.
- Products: Oxygen gas and carbohydrates. Diatoms store energy primarily as chrysolaminarin, a water-soluble polysaccharide.
These examples underscore the diversity of photosynthetic processes across different organisms, each adapted to their unique environments and energy requirements.
Importance of photosynthesis
- Energy Conversion for Autotrophs:
- Photosynthesis serves as the fundamental energy conversion mechanism for autotrophic organisms. Through this process, they harness solar energy, converting it into chemical energy stored in the form of glucose and other carbohydrates. This self-sustenance allows them to thrive in diverse ecosystems.
- Foundation of the Food Chain:
- Autotrophs, primarily plants and certain bacteria, form the base of the food chain. Heterotrophic organisms, including animals and humans, rely on these primary producers for their energy and nutritional needs. Without photosynthesis, the foundation of this chain would collapse, disrupting the balance of ecosystems.
- Oxygen Production and Atmospheric Balance:
- Photosynthesis is a critical contributor to the planet’s oxygen levels. As plants and photosynthetic bacteria convert carbon dioxide into glucose, they release oxygen as a byproduct. This oxygen replenishes the atmosphere, ensuring its availability for aerobic respiration in various organisms.
- Carbon Sequestration:
- Through photosynthesis, plants play a pivotal role in the global carbon cycle. They absorb atmospheric carbon dioxide, converting it into organic compounds. This process aids in mitigating the effects of excessive carbon dioxide, which is a major greenhouse gas contributing to global warming.
- Symbiotic Relationships:
- Photosynthesis facilitates various symbiotic relationships in nature. For instance, plants provide oxygen and nutrients to animals, which in turn produce carbon dioxide and other organic matter that plants utilize. This mutualistic relationship underscores the interconnectedness of life.
- Harnessing Solar Energy:
- The sun is the ultimate energy source for Earth. Through photosynthesis, this radiant energy is captured and transformed into a form that can be used by a myriad of organisms. This process underscores the significance of solar energy in sustaining life on the planet.
In essence, photosynthesis is not just a biological process but a cornerstone of life on Earth. It interlinks various biogeochemical cycles, supports biodiversity, and ensures the continuity of energy flow in ecosystems.
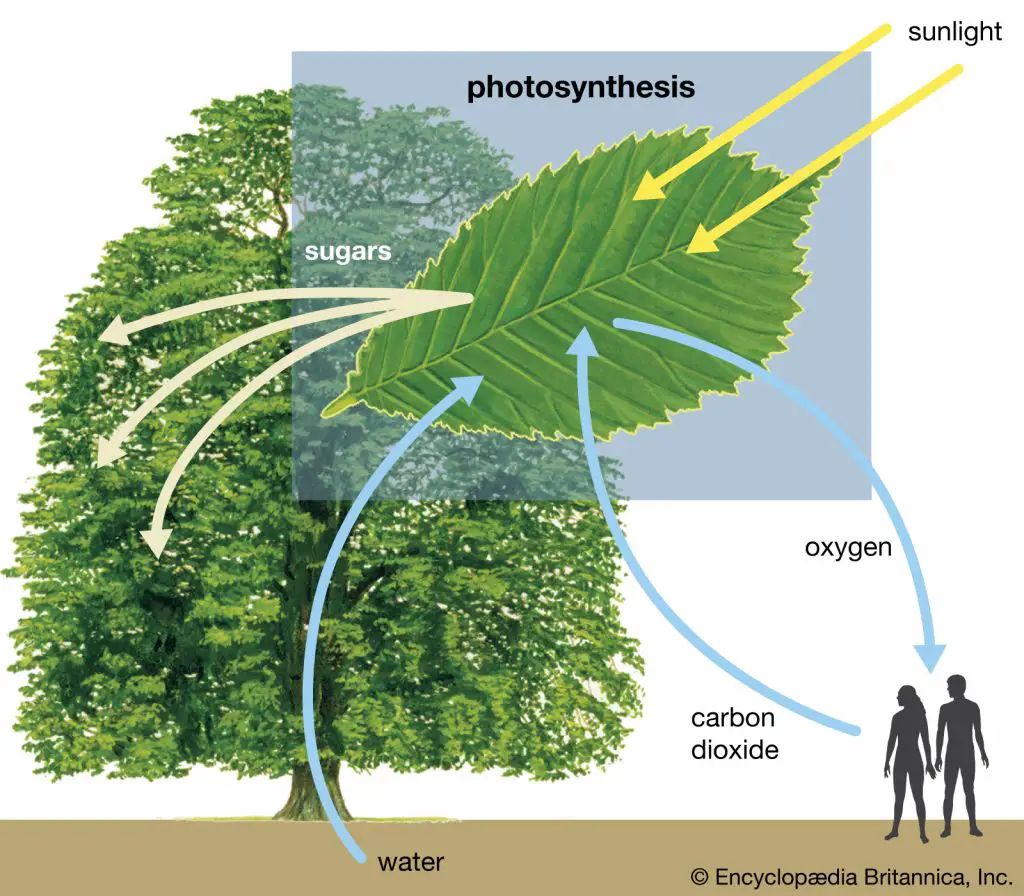
What is Artificial photosynthesis?
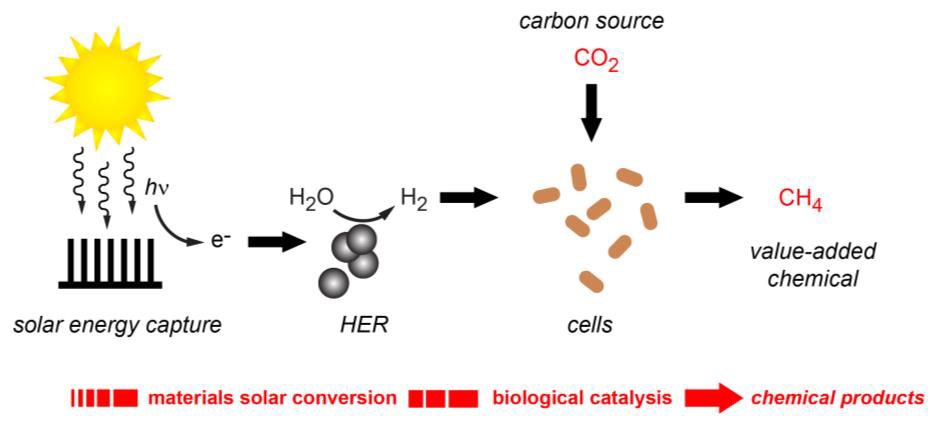
- Definition and Inspiration:
- Artificial photosynthesis refers to a chemically engineered process that emulates the natural mechanism by which plants, algae, and certain bacteria harness sunlight to convert water and carbon dioxide into oxygen and energy-rich carbohydrates.
- Role of Photocatalysts:
- Central to artificial photosynthesis is the use of photocatalysts. These are specialized compounds designed to facilitate the oxidation-reduction reactions analogous to those in natural photosynthesis. Their role is pivotal in capturing and converting solar energy efficiently.
- Objective: Solar Fuel Production:
- The primary aim of artificial photosynthesis is the generation of solar fuels. These are energy carriers synthesized directly from sunlight, allowing for energy storage and utilization even in the absence of direct sunlight.
- Clean Energy Generation:
- One of the significant outcomes of artificial photosynthesis is the production of oxygen from water and sunlight. This process offers a sustainable and environmentally friendly approach to energy generation, minimizing carbon emissions and other pollutants.
- Photocatalytic Water Splitting:
- A crucial component of artificial photosynthesis is the photocatalytic division of water molecules. This process yields oxygen and a substantial volume of hydrogen gas, a potential clean fuel source with myriad applications.
- Carbon Reduction and Fixation:
- Beyond water splitting, artificial photosynthesis can also drive carbon reduction, mirroring the natural carbon fixation pathway. This results in the synthesis of carbohydrate molecules, providing another avenue for energy storage.
- Applications and Potential:
- The implications of artificial photosynthesis are vast. It holds promise in diverse fields such as photoelectrochemistry, enzyme engineering, and the development of photoautotrophic microorganisms. These applications can lead to the production of microbial biofuels and biohydrogen, offering sustainable energy solutions derived directly from sunlight.
Photosynthesis vs Cellular respiration
Photosynthesis and cellular respiration are two fundamental biochemical processes that sustain life on Earth. While they are interconnected and interdependent, they serve distinct roles in the energy dynamics of living organisms. Here, we elucidate the key differences between these two processes:
- Nature of the Process:
- Photosynthesis:
- Type: An anabolic process, which means it involves the synthesis of complex molecules from simpler ones.
- Energy Dynamics: Endergonic and endothermic, signifying that it absorbs energy.
- Cellular Respiration:
- Type: A catabolic process, implying the breakdown of complex molecules to release energy.
- Energy Dynamics: Exergonic and exothermic, indicating that it releases energy.
- Photosynthesis:
- Site of Occurrence:
- Photosynthesis: Takes place in the chloroplasts of eukaryotic phototrophic cells, specifically within the thylakoids.
- Cellular Respiration: Primarily occurs in the mitochondria of cells.
- Reactants and Products:
- Photosynthesis:
- Reactants: Carbon dioxide, water, and light energy.
- Products: Glucose (a carbohydrate), oxygen, and in some instances, water.
- Cellular Respiration:
- Reactants: Glucose and oxygen.
- Products: Carbon dioxide, water, and energy in the form of adenosine triphosphate (ATP).
- Photosynthesis:
- Chemical Reactions:
- Photosynthesis: 6CO2 + 6H2O (in the presence of light energy) → C6H12O6 + 6O2
- Cellular Respiration: C6H12O6 + 6O2 → 6CO2 + 6H2O
- Organisms Involved:
- Photosynthesis: Conducted by green plants, certain algae, and some photosynthetic bacteria.
- Cellular Respiration: A universal process that occurs in all living organisms.
- Dependency on Sunlight:
- Photosynthesis: Exclusively occurs in the presence of sunlight.
- Cellular Respiration: A continuous process that does not necessitate sunlight.
Criteria | Photosynthesis | Cellular Respiration |
---|---|---|
Location of Occurrence | Green plants, algae, and certain photosynthetic bacteria. | All living organisms. |
Cellular Organelle Involved | Occurs in the thylakoids of chloroplasts. | Takes place in the mitochondria. |
Reactants | Light energy, carbon dioxide (CO2), and water (H2O). | Glucose (C6H12O6) and oxygen (O2). |
Chemical Equation | 6CO2 + 6H2O → C6H12O6 + 6O2 | 6O2 + C6H12O6 → 6CO2 + 6H2O |
Products | Glucose (C6H12O6) and oxygen (O2). | Carbon dioxide (CO2) and water (H2O). |
Nature of the Process | Anabolic – involves the synthesis of organic molecules from simpler precursors. | Catabolic – involves the breakdown of organic molecules to release energy. |
Energy Dynamics | Endergonic – consumes energy from sunlight to drive the synthesis of glucose. | Exergonic – releases energy through the oxidation of glucose. |
Dependency on Sunlight | Operates only in the presence of sunlight. | Continuously active, independent of sunlight. |
Evolution of Photosynthesis
The evolution of photosynthesis is a fascinating journey through Earth’s history, representing a pivotal moment in the development of life on our planet. This process has not only shaped the composition of the Earth’s atmosphere but has also played a fundamental role in the emergence of complex life forms. In this scientific exploration, we delve into the key milestones and mechanisms underlying the evolution of photosynthesis.
Early Photosynthetic Organisms:
The fossil record provides intriguing insights into the origins of photosynthesis. Fossils of filamentous photosynthetic organisms, dating back approximately 3.4 billion years, suggest that photosynthesis may have commenced around this time. These ancient organisms likely laid the foundation for the photosynthetic processes we observe today.
Oxygenic Photosynthesis Emergence:
The most significant shift in Earth’s history was the rise of oxygenic photosynthesis, which ultimately led to the oxygenation of our planet. Geological evidence points to the emergence of oxygenic photosynthesis, notably in cyanobacteria, during the Paleoproterozoic era, roughly 2 billion years ago. This marked the inception of an era often referred to as the “oxygen catastrophe,” as oxygen levels in the atmosphere began to rise significantly.
Symbiosis and Chloroplast Origins:
One intriguing aspect of photosynthesis evolution is the symbiotic relationships that have developed between photosynthetic organisms and various life forms. Notably, corals, sponges, sea anemones, and even certain mollusks, such as Elysia viridis and Elysia chlorotica, have formed symbiotic connections with photosynthetic algae. These relationships are often attributed to the simplicity of the host organisms’ body plans and their ability to maximize surface area for photosynthesis.
Endosymbiotic Theory and Chloroplasts:
A pivotal moment in the evolution of photosynthesis occurred with the origin of chloroplasts. Chloroplasts, which bear striking similarities to photosynthetic bacteria, are thought to have originated through endosymbiosis. According to this theory, early eukaryotic cells engulfed photosynthetic bacteria, eventually forming the first plant cells. The evidence for this theory lies in the presence of chloroplast DNA, separate from the host cell’s nucleus, resembling the genetic makeup of cyanobacteria. This genetic legacy supports the notion that chloroplasts evolved from photosynthetic bacteria.
Diversity of Photosynthetic Lineages:
Photosynthesis has diversified over time, resulting in various lineages of photosynthetic organisms. These include:
- Archaeplastida (uni- and multicellular): Comprising glaucophytes, red and green algae.
- Cryptista (unicellular): Encompassing cryptophytes.
- Haptista (unicellular): Represented by haptophytes.
- Alveolata (unicellular): Incorporating dinoflagellates, chromerids, and Pseudoblepharisma.
- Stramenopila (uni- and multicellular): Encompassing ochrophytes.
- Rhizaria (unicellular): Comprising chlorarachniophytes and select Paulinella species.
- Excavata (unicellular): Including euglenids.
These lineages, whether uni- or multicellular, reveal the diversification of photosynthetic organisms and their adaptive strategies.
Prokaryotic Photosynthesis:
Early photosynthetic systems, particularly those of green and purple sulfur and green and purple nonsulfur bacteria, were likely anoxygenic and utilized various molecules, such as hydrogen, sulfur, and organic acids, as electron donors. These systems were consistent with the highly reducing conditions of the early Earth’s atmosphere. Archaea, including haloarchaea, can harness energy from the sun but do not perform oxygenic photosynthesis.
In conclusion, the evolution of photosynthesis is a captivating narrative of life’s adaptation to Earth’s changing conditions. From the emergence of early photosynthetic organisms to the rise of oxygenic photosynthesis and the formation of symbiotic relationships, photosynthesis has played a pivotal role in shaping our planet’s history and continues to be a cornerstone of life as we know it. This intricate process underscores the remarkable complexity and diversity of life on Earth.
Quiz Practice
MCQ 1: What is the primary pigment responsible for capturing light energy in photosynthesis?
A) Chlorophyll
B) Carotenoid
C) Xanthophyll
D) Phycobilin
MCQ 2: In which organelle does photosynthesis primarily occur in plant cells?
A) Nucleus
B) Mitochondria
C) Chloroplast
D) Endoplasmic reticulum
MCQ 3: During the light-dependent reactions of photosynthesis, what molecule is produced as a byproduct?
A) Oxygen
B) Carbon dioxide
C) Glucose
D) Water
MCQ 4: What is the primary source of carbon dioxide for photosynthesis in most plants?
A) Soil
B) Air
C) Water
D) Other plants
MCQ 5: Which of the following colors of light is least effective in driving photosynthesis?
A) Red
B) Blue
C) Green
D) Yellow
MCQ 6: What is the ultimate goal of the Calvin cycle in photosynthesis?
A) Produce ATP
B) Convert glucose to starch
C) Generate carbon dioxide
D) Synthesize glucose
MCQ 7: In C4 plants, what is the primary function of mesophyll cells?
A) Storing water
B) Capturing light energy
C) Fixing carbon dioxide
D) Producing oxygen
MCQ 8: What gas is necessary for the opening of stomata during photosynthesis?
A) Oxygen
B) Carbon dioxide
C) Nitrogen
D) Hydrogen
MCQ 9: Which environmental factor can limit the rate of photosynthesis?
A) High oxygen levels
B) Low carbon dioxide levels
C) Warm temperatures
D) Bright sunlight
MCQ 10: What is the primary purpose of the light-independent reactions (Calvin cycle) in photosynthesis?
A) Generate oxygen
B) Produce ATP
C) Fix carbon dioxide
D) Capture light energy
FAQs
Where does photosynthesis take place?
In plants, photosynthesis takes place in chloroplasts, which contain the chlorophyll. Chloroplasts are surrounded by a double membrane and contain a third inner membrane, called the thylakoid membrane, that forms long folds within the organelle.
What are the reactants of photosynthesis?
The process of photosynthesis is commonly written as: 6CO2 + 6H2O → C6H12O6 + 6O2. This means that the reactants, six carbon dioxide molecules and six water molecules, are converted by light energy captured by chlorophyll (implied by the arrow) into a sugar molecule and six oxygen molecules, the products.
How are photosynthesis and cellular respiration related?
Photosynthesis is the process by which atmospheric carbon dioxide is assimilated and converted to glucose and oxygen is released. CO2 and H2O are utilised in the process. In the cellular respiration, glucose is broken down to CO2 and energy is released in the form of ATP, which is utilised in performing various metabolic processes. Oxygen is utilised in the process. Energy is stored in the process of photosynthesis, whereas it is released in the process of cellular respiration.
The process of cellular respiration and photosynthesis complement each other. These processes help cells to release and store the energy respectively. They are required to keep the atmospheric balance of carbon dioxide and oxygen concentrations.
What is the equation for photosynthesis?
The process of photosynthesis is commonly written as: 6CO2 + 6H2O → C6H12O6 + 6O2
Where does photosynthesis occur?
chloroplasts
What are the products of photosynthesis?
Let’s look at the products of photosynthesis! During the process of photosynthesis plants break apart the reactants of carbon dioxide and water and recombine them to produce oxygen (O2) and a form of sugar called glucose (C6H12O6).
Why is photosynthesis important?
Photosynthesis is the main source of food on earth. It releases oxygen which is an important element for the survival of life. Without photosynthesis, there will be no oxygen on earth. The stored chemical energy in plants flows into herbivores, carnivores, predators, parasites, decomposers, and all life forms.
Which of these equations best summarizes photosynthesis?A. C6H12O6 + 6 O2 → 6 CO2 + 6 H2O + EnergyB. C6H12O6 + 6 O2 → 6 CO2 + 12 H2OC. 6 CO2 + 6 H2O → C6H12O6 + 6 O2D. 6 CO2 + 6 O2 → C6H12O6 + 6 H2OE. H2O → 2 H+ + 1/2 O2 + 2e-
Ans: C. 6 CO2 + 6 H2O → C6H12O6 + 6 O2
What are the raw materials of photosynthesis?
The raw materials of photosynthesis, water and carbon dioxide, enter the cells of the leaf. Oxygen, a by-product of photosynthesis, and water vapor exit the leaf.
Which gas is removed from the atmosphere during photosynthesis?
Photosynthesis removes CO2 from the atmosphere and replaces it with O2.
Which of the following sequences correctly represents the flow of electrons during photosynthesis?A. NADPH → O2 → CO2B. H2O → NADPH → Calvin cycleC. NADPH → chlorophyll → Calvin cycleD. H2O → photosystem I → photosystem II
The correct option is B
H2O → NADPH → Calvin cycle
Electrons flow from water through the photosystem II, electron transport chain, and photosystem I to NADP+. The electrons of NADPH thus formed are then used in the Calvin cycle.
What organelle does photosynthesis occur in
chloroplasts
what are the inputs of photosynthesis?
In photosynthesis, water, carbon dioxide, and energy in the form of sunlight are inputs, and the outputs are glucose and oxygen.
References
- Leegood, R. C. (2013). Photosynthesis. Encyclopedia of Biological Chemistry, 492–496. doi:10.1016/b978-0-12-378630-2.00049-9
- Leegood, R. C. (2004). Photosynthesis. Encyclopedia of Biological Chemistry, 330–335. doi:10.1016/b0-12-443710-9/00487-7
- Boyer, R. (2006). Concepts in Biochemistry, 3rd edition. John Wiley & Sons.
- Heldt, H. W. (2005). Plant Biochemistry, 3rd edition. Academic Press.
- Martin, W. F., Garg, S., & Zimorski, V. (2015). Endosymbiotic theories for eukaryote origin. Philosophical Transactions of the Royal Society B: Biological Sciences, 370(1678), 20140330. doi:10.1098/rstb.2014.0330
- Milne, B. F., Toker, Y., Rubio, A., & Nielsen, S. B. (2015). Unraveling the Intrinsic Color of Chlorophyll. Angewandte Chemie International Edition, 54(7), 2170-2173. doi:10.1002/anie.201410899
- Gao, J., Wang, H., Yuan, Q., & Feng, Y. (2018). Structure and Function of the Photosystem Supercomplexes. Frontiers in Plant Science, 9. doi:10.3389/fpls.2018.00357
- Rutherford, A. W., & Faller, P. (2003, January). “Photosystem II: evolutionary perspectives.” Philosophical Transactions of the Royal Society of London. Series B, Biological Sciences, 358(1429), 245–253. doi:10.1098/rstb.2002.1186. PMC 1693113. PMID 12594932.
- Arnon, D. I., Whatley, F. R., & Allen, M. B. (1954). “Photosynthesis by isolated chloroplasts. II. Photophosphorylation, the conversion of light into phosphate bond energy.” Journal of the American Chemical Society, 76(24), 6324–6329. doi:10.1021/ja01653a025.
- Ehrenberg, R. (2017, December 15). “The photosynthesis fix.” Knowable Magazine. Annual Reviews. doi:10.1146/knowable-121917-115502. Retrieved April 3, 2018.
- El-Sharkawy, M. A., & Hesketh, J. D. (1965). “Photosynthesis among species in relation to characteristics of leaf anatomy and CO2 diffusion resistances.” Crop Science, 5(6), 517–521. doi:10.2135/cropsci1965.0011183x000500060010x.
- Earl, H., & Said Ennahli, S. (2004). “Estimating photosynthetic electron transport via chlorophyll fluorometry without Photosystem II light saturation.” Photosynthesis Research, 82(2), 177–186. doi:10.1007/s11120-004-1454-3. PMID 16151873. S2CID 291238.