Contents
What is Ear?
- The ear is one of the five sensory organs of the human body and serves the primary functions of hearing and maintaining balance. It is a complex structure consisting of three main sections: the outer ear, middle ear, and inner ear.
- The outer ear is the visible part of the ear that collects sound waves from the environment. It consists of the pinna, which is the external part of the ear, and the ear canal. The sound waves enter the ear canal and travel towards the middle ear.
- The middle ear is located between the outer ear and the inner ear. It contains the eardrum, a thin membrane that vibrates in response to sound waves. The vibrations from the eardrum are then transmitted to three small bones called the ossicles: the malleus (hammer), incus (anvil), and stapes (stirrup). These bones amplify and transmit the vibrations to the inner ear.
- The inner ear is the innermost part of the ear and is responsible for converting sound waves into electrical signals that can be interpreted by the brain. It contains the cochlea, a snail-shaped structure that is the main organ of hearing. The cochlea is filled with fluid and contains tiny hair cells that are sensitive to vibrations. When the vibrations from the middle ear reach the cochlea, the hair cells detect the movements and convert them into electrical impulses. These impulses are then transmitted to the brain through the auditory nerve for further processing and interpretation.
- In addition to hearing, the inner ear also plays a crucial role in maintaining balance. It contains the vestibular labyrinth, which consists of fluid-filled canals and chambers that detect the position of the head and the body in relation to gravity. This information is essential for maintaining equilibrium and coordinating movements.
- The process of hearing involves the transduction of sound waves into electrochemical impulses. Sound is a mechanical energy wave that travels through various mediums, such as air or other physical substances. When sound waves enter the ear, they cause the particles of the medium to vibrate parallel to the direction of the sound wave, creating compressions and refractions. The specific vibration patterns produced by a sound source, such as a human speaker, are converted into auditory signals by the ear.
- Hearing is a vital sense that allows us to perceive and interpret sounds, communicate, and detect environmental signals. The human ear has a hearing range of 20 to 20,000 Hz through air conduction, while bone conduction can transmit much higher frequencies. The structure and functioning of the ear are essential for normal hearing, involving the coordinated interplay of the outer, middle, and inner ear.
- In summary, the ear is a complex organ responsible for both hearing and maintaining balance. Its structure consists of the outer ear, middle ear, and inner ear, with the cochlea being the primary organ of hearing. The ear converts sound waves into electrical impulses, which are then processed by the brain for interpretation. Understanding the structure and mechanisms of the ear is important for comprehending the process of hearing and its integration with the central auditory pathways of the brain.
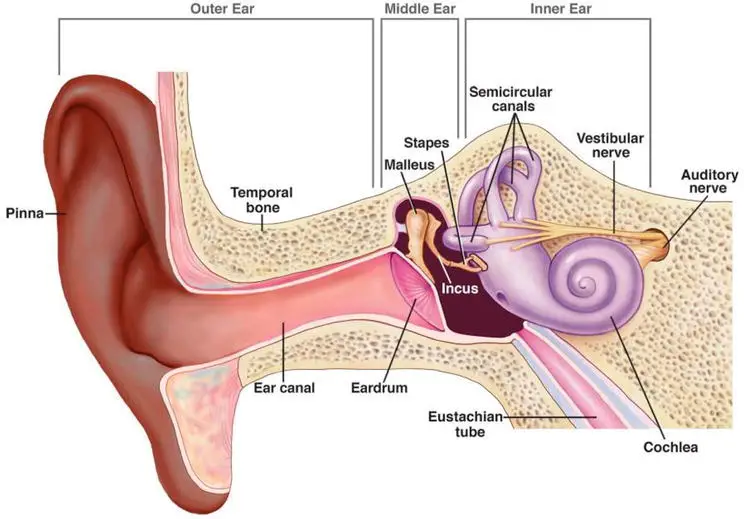
Definition of Ear
The ear is a sensory organ responsible for hearing and maintaining balance in the body. It consists of the outer ear, middle ear, and inner ear, and it converts sound waves into electrical signals that can be interpreted by the brain.
Anatomy of Ear – Structure of Ear
The three anatomical regions of the ear are – the outer ear, the middle ear and the inner ear.
1. Outer Ear
The outer ear consists of several parts that contribute to its overall anatomy and function.
- Pinna: The pinna, also known as the auricle, is the visible outermost part of the ear. It has a curved shape and is composed of cartilage covered with skin. The pinna has fine hairs and glands that secrete wax. Its primary function is to collect sound waves from the environment and direct them into the ear canal.
- External Auditory Canal: The external auditory canal, also called the meatus, is a curved tube that connects the pinna to the tympanic membrane. It is approximately 2.5 centimeters long, with the outer one-third made of elastic cartilage and the inner two-thirds composed of bone. The external auditory canal is lined with skin and also contains wax-producing glands. Its purpose is to channel sound waves towards the eardrum.
- Tympanic Membrane: The tympanic membrane, commonly known as the eardrum, is a thin, circular membrane located at the end of the external auditory canal. It separates the outer ear from the middle ear. The eardrum is composed of connective tissue and is covered by skin on the outer side and a mucous membrane on the inner side. When sound waves reach the eardrum, they cause it to vibrate, transmitting the sound energy to the middle ear.
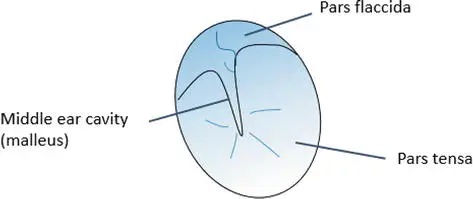
The parts of the outer ear work together to capture and transmit sound waves to the middle ear. The pinna collects sound, the external auditory canal channels the sound waves, and the tympanic membrane vibrates in response to the incoming sound. Understanding the structure and function of the outer ear is crucial, as abnormalities or defects in this region can lead to hearing impairments and various ear-related conditions.
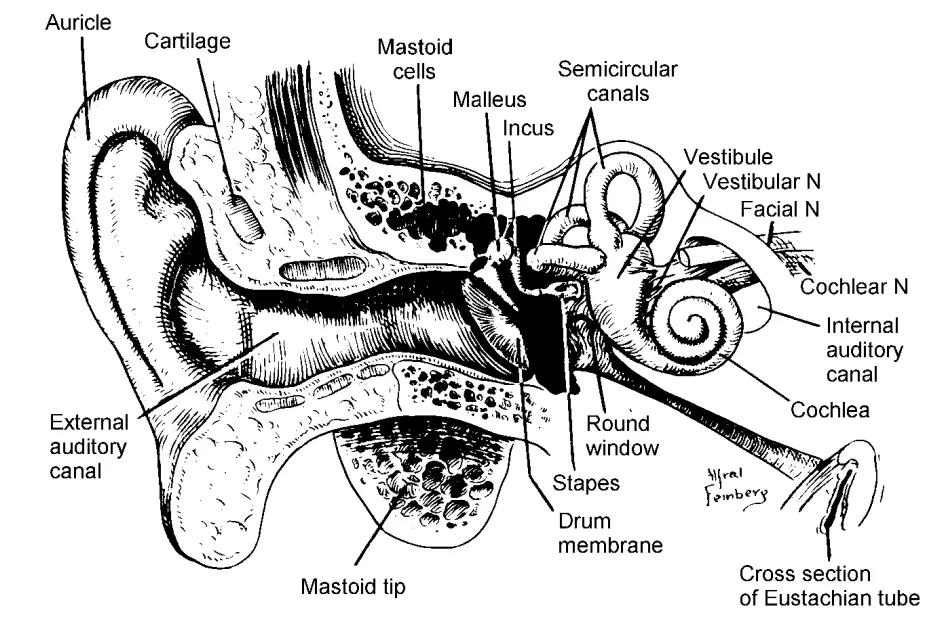
Outer ear Anatomy
- The outer ear consists of various anatomical structures that play a crucial role in receiving and transmitting sound vibrations.
- The auricle, also known as the pinna, is the visible part of the outer ear located on the side of the head. It is composed of a thin plate of yellow elastic cartilage that is shaped into distinct ridges, furrows, and hollows, forming an irregular shallow funnel. The concha, which is the deepest depression of the auricle, leads to the external auditory canal. The helix is a prominent curved ridge that emerges from the base of the concha and continues as the rim of the upper part of the auricle. Inside the helix, there is the antihelix, which is an inner ridge that encircles the concha and is separated from the helix by the scapha, a shallow groove or depression.
- The external auditory canal, also called the ear canal, is a somewhat curved tube that extends inward from the base of the concha and terminates blindly at the tympanic membrane or eardrum. The external third of the canal is composed of cartilage, while the inner two-thirds consist of bone. The lining of the ear canal is covered by skin, which continues onto the outer surface of the tympanic membrane. Thin hairs, directed towards the exterior, as well as modified sweat glands that produce earwax, line the canal. These structures help protect the ear by preventing the entry of foreign particles.
- The pinna, or auricle, receives sound in the form of vibrations. When sound waves enter the outer ear, they travel through the external auditory canal and vibrate the eardrum, which is located at the end of the canal. These vibrations are the initial step in the process of sound transmission, as they set off a chain of events that ultimately lead to the perception and interpretation of sound.
- Understanding the anatomy of the outer ear is crucial for comprehending how sound is captured, funneled, and transmitted towards the middle and inner ear structures. Each component of the outer ear plays a specific role in this process, allowing us to perceive and interpret the sounds around us.
Parts of Outer ear
a. Pinna
- The pinna, also known as the auricle, is a prominent and protruding structure located on the side of the skull. It is composed of cartilage and is completely covered with skin. The primary function of the pinna is to collect sound vibrations from the surrounding environment and direct them into the auditory canal.
- The shape and structure of the pinna play a role in localizing sounds. Due to its angle and position, the pinna is more efficient at catching sounds that come from the front than those coming from behind. This helps in determining the direction of sound sources. However, this effect is more pronounced for high-frequency sounds because of the wavelength of audible sound vibrations and the relative size of the head.
- In addition to the pinna, the head itself contributes to sound localization. For middle frequencies, the head acts as an obstruction and casts a shadow of sound, allowing us to perceive the direction from which the sound is coming. For lower frequencies, the phases of sound arrival between the ears play a crucial role in localizing the sound source.
- Overall, the pinna serves as an important structure in the outer ear, collecting and funneling sound vibrations into the auditory canal. Its shape and position aid in localizing sounds, along with the contributions of the head and the interplay of sound phases between the ears.
b. Auditory Canal
- The auditory canal, also known as the ear canal, is a tube-like structure that extends approximately 4 cm in length. It consists of two distinct parts: the outer hairy part and the inner thinner part.
- The outer part of the auditory canal is covered with hairy skin and contains sebaceous glands (oil glands) and sweat glands. These glands, along with the production of keratin, contribute to the formation of ear wax. Ear wax, also known as cerumen, plays a protective role by trapping dust, debris, and foreign particles that may enter the ear. It also helps to lubricate the ear canal and prevent it from drying out.
- Moving inward, the skin of the auditory canal becomes thinner and is firmly attached to the underlying bone of the ear canal. This bony portion of the canal acts as a hard cavity that helps absorb faint sounds and directs them towards the base of the canal, where the tympanic membrane or eardrum is located.
- The auditory canal serves as a passageway for sound waves to travel from the outer ear to the middle ear. The presence of hair, glands, and ear wax in the outer part of the canal provides a protective barrier, preventing harmful substances from reaching the delicate structures deeper in the ear. The bony portion of the canal contributes to sound transmission by aiding in the absorption and direction of sound towards the eardrum.
- Understanding the anatomy and function of the auditory canal is important for maintaining proper ear hygiene and protecting the ear from potential damage or infections.
c. Tympanic membrane
- The tympanic membrane, commonly known as the eardrum, is a vital component of the middle ear. It consists of two distinct layers: an outer layer continuous with the skin of the auditory canal and an inner layer known as the endoderm.
- The outer layer of the tympanic membrane is composed of stratified and squamous epithelium, which is a type of skin tissue. It exhibits a unique lateral migration of cells from the center to the edges of the membrane, where these cells eventually shed. This process is responsible for the self-cleaning property of the outer ear.
- The inner layer of the tympanic membrane is made up of a simple squamous epithelium, which is a thin, flat layer of cells. Together, these layers form a tri-layered structure with a middle layer of loose connective tissue composed of neural crest cells.
- The tympanic membrane can be divided into two main regions based on its morphology. The first is the ventral pars tensa, which is a tense and vibration-appropriate region. The second is the dorsal pars flaccida, also known as Shrapnell’s membrane, located in the upper part of the tympanic membrane above the malleolar fold. The pars flaccida is relatively more fragile compared to the larger pars tensa.
- Both the pars tensa and pars flaccida consist of three layers: an inner layer of neural crest cells surrounded by two epithelium layers. In the pars tensa, the inner layer, known as the lamina propria, is further divided into two collagen-rich connective tissue layers: the outer radiative layer and the inner circular layer. However, the pars flaccida lacks a regular arrangement of extracellular matrix in its inner layer.
- These structural and functional differences between the pars tensa and pars flaccida explain why conditions like retraction pockets, where the tympanic membrane is pulled deeper into the middle ear cavity, are more commonly found in the pars flaccida and can cause pain.
- The entire tympanic membrane has a thickness of about 0.1 millimeters and covers a round opening of approximately 1 cm in diameter in the middle ear cavity. While the term “eardrum” is often used to refer to the tympanic membrane, technically, it is the middle ear cavity itself that acts as the eardrum, with the tympanic membrane functioning as the drum skin.
- Understanding the structure and composition of the tympanic membrane is crucial for comprehending its role in sound transmission and the development of certain ear conditions.
Functions of Outer Ear
- Pinna: The unique shape of the pinna helps to catch sound waves and funnel them into the auditory canal.
- Auditory Canal: The auditory canal acts as a resonating tube, amplifying sounds within the frequency range of 3000 to 4000 Hz, thereby increasing the sensitivity of the ear at these frequencies.
- Sound Localization: The crinkle shape of the pinna, along with the natural barrier created by the head, assists in localizing sound by analyzing differences in intensity and arrival time between the ears.
2. Structure and physiology of middle ear
The middle ear is an air-filled cavity located at the back of the nose, connected to it by a slender tube called the Eustachian tube. It is bordered by the tympanic membrane on the outer side and the cochlea on the inner side. The floor of the middle ear consists of a thin bony plate that covers the initial part of the jugular bulb, a major vein responsible for draining blood from the head. At its upper boundary, the middle ear forms the bone beneath the middle lobe of the brain. Towards the front of the middle ear, there is the opening of the Eustachian tube, while the posterior end leads to the mastoid cells, a group of air cells present within the temporal bone.
The lining of the middle ear is composed of a respiratory membrane, which is essentially an extension of the respiratory air spaces found in the sinuses and nose. This membrane is thicker near the Eustachian tube and becomes thinner as it passes through the mastoid region. It has the ability to produce mucus. While the Eustachian tube is bony as it leaves the middle ear, it consists of cartilage and muscle in the nasopharynx. Contraction of the muscles actively opens the tube, enabling the equalization of air pressure between the middle ear and the nose.
Understanding the anatomical features of the middle ear, including its connection to the nasal cavity through the Eustachian tube, its relationship with the tympanic membrane and cochlea, and its association with important structures like the jugular bulb and mastoid cells, is essential for comprehending its function in hearing and its role in maintaining proper air pressure within the ear.
a. Auditory ossicles
- The middle ear is home to a set of three small bones known as the auditory ossicles: the malleus (hammer), incus (anvil), and stapes (stirrup) (Figure 1). These ossicles play a crucial role in transmitting sound vibrations from the tympanic membrane to the inner ear.
- Starting with the malleus, it is shaped like a club with its handle embedded in the tympanic membrane. The handle runs along the center of the membrane, extending upward, while its head rests in the middle ear cavity above the tympanic membrane. The malleus is suspended through a ligament from a bone that covers the brain. The head of the malleus articulates with the cone-shaped incus. The base of the cone articulates with the malleus head, positioned above the tympanic membrane.
- The incus, positioned between the other two ossicles, has a thin projection called the long process that extends outward. It hangs freely within the middle ear and is connected to the stapes at its tip, which has a right-angle bend.
- The stapes, the third ossicle, has an arch-like shape and consists of a footplate and an arch. The footplate is connected to the oval window, an opening that leads to the vestibular system of the inner ear or cochlea, through the stapedio-vestibular joint.
- Together, the malleus, incus, and stapes form a chain of bones that efficiently transmit sound vibrations from the tympanic membrane to the oval window, initiating the process of sound perception in the inner ear.
Middle Ear – Parts of the Middle Ear Summery
The middle ear consists of several important components:
- The three tiny bones: The middle ear contains three small bones known as the malleus (hammer), incus (anvil), and stapes (stirrup). They are arranged in the order of malleus, incus, and stapes. The malleus is attached to the tympanic membrane, while the incus is positioned between the malleus and stapes. The stapes, the smallest bone in the body, is stirrup-shaped and connected to the oval window of the cochlea.
- The Eustachian tube: This tube serves as the connection between the middle ear and the pharynx (throat). It plays a crucial role in equalizing the pressure between the middle ear and the outer atmosphere, ensuring optimal functioning of the ear.
- Amplification and transmission of sound: The middle ear is responsible for amplifying sound waves and transmitting them to the inner ear. The chain of ossicles (malleus, incus, and stapes) efficiently transfers sound vibrations from the tympanic membrane to the oval window of the cochlea.
The middle ear cavity is an air-filled space with distinct chambers. The upper chamber is called the tympanum, while the lower chamber is known as the epitympanum. These chambers are separated by a small constriction and are referred to as the atrium and attic, respectively. The middle ear cavity can be visualized as a rectangular room with walls, a roof, and a floor.
The lateral wall is formed by the tympanic membrane, while the superior wall is a bony partition separating the cranial and middle ear cavities from the brain. The inferior wall is a thin plate that separates the middle ear from the jugular vein and carotid artery. The posterior wall separates the middle ear cavity from the mastoid antrum. The anterior wall contains the opening of the Eustachian tube, which connects the middle ear to the nasopharynx. Finally, the inner wall separates the middle ear from the inner ear and forms a section of the otic capsule.
Overall, the middle ear plays a crucial role in amplifying and transmitting sound vibrations from the outer ear to the inner ear, enabling us to perceive and interpret auditory stimuli.
Functions of middle ear
- Conducting Sound: The middle ear contains the three tiny bones called ossicles (malleus, incus, and stapes). These bones transmit sound vibrations from the tympanic membrane (eardrum) to the inner ear. They act as a lever system to amplify the sound signal.
- Pressure Equalization: The Eustachian tube connects the middle ear to the nasopharynx and helps equalize air pressure on both sides of the tympanic membrane. It opens periodically during swallowing or yawning, ensuring the middle ear remains at the same pressure as the outside environment.
- Amplification: The ossicular chain, along with the hydraulics of the middle ear system, amplifies sound vibrations. The large surface area of the tympanic membrane and the leverage of the ossicles result in the conversion of smaller movements over a larger area into larger movements over a smaller area, effectively amplifying the sound signal.
- Protection: The middle ear cavity contains mastoid air cells, which act as air reservoirs to cushion the pressure changes. They help maintain a stable environment in the middle ear and protect against conductive hearing loss caused by prolonged negative pressure.
The combined effect of the outer and middle ear functions results in the amplification of sound by approximately 30 decibels (dB) as the sound travels from the external environment to the inner ear. This amplification enhances the sensitivity of the auditory system and allows us to perceive a wide range of sound frequencies and intensities.
3. Inner ear
The inner ear, located between the middle ear and the acoustic meatus, consists of a complex structure known as the labyrinth. The labyrinth can be divided into two types: the bony labyrinth and the membranous labyrinth, each with its own subdivisions.
The bony labyrinth is composed of three main parts: the semicircular canals, the vestibule, and the cochlea. The semicircular canals are responsible for detecting rotational movements and help maintain balance. The vestibule contains two sac-like structures called the saccule and the utricle, which play a role in detecting linear acceleration and the position of the head. The cochlea, on the other hand, is involved in the process of hearing.
The membranous labyrinth consists of the semicircular ducts, which are continuous with the semicircular canals of the bony labyrinth, as well as the cochlear duct and the sac-like structures of the vestibule (saccule and utricle). The space between the bony and membranous labyrinths is filled with perilymph, a watery fluid similar to the aqueous humor of the eyes and cerebrospinal fluid. The composition of perilymph is low in potassium and high in sodium ions.
Within the membranous labyrinth, there is another fluid called endolymph, which has a high potassium concentration and a different composition from perilymph. Endolymph is produced by vestibular dark cells, which have similarities to the stria vascularis found in the cochlea. The interaction between the endolymph and hair cells within the membranous labyrinth leads to the depolarization of hair cells, resulting in the transmission of nerve signals.
The inner ear is divided into two systems: the vestibular system and the cochlear system. The vestibular system is involved in maintaining balance and equilibrium and is proprioceptive in nature, meaning it relies on feedback loops within the sensory organs and the nervous system rather than external stimuli. On the other hand, the cochlear system is responsible for hearing and is considered exteroceptive, as the sensation in the cochlea is triggered by external stimuli, such as sound waves.
Overall, the inner ear is a complex structure that plays a crucial role in both the sense of balance and the perception of sound.
a. Vestibular system
- The vestibular system, a sensory system within the inner ear, plays a crucial role in maintaining postural equilibrium and coordinating head and eye movements. It consists of five organs: three semicircular canals and two otolith organs.
- The semicircular canals are positioned at right angles to each other and are responsible for detecting angular (head) rotation. Each canal opens into the vestibule through an expanded end called the ampulla. Within the ampulla, there is a sensory neuroepithelium known as the crista ampullaris, which contains a ridge of tissues. The ampulla is divided into equal parts by a gelatinous protein-polysaccharide structure called the cupula, which helps to keep the hair cells in place. When there is rotational acceleration, the endolymph displaces the cupula, causing the hair cells to bend in the opposite direction of the acceleration.
- The vestibule contains two membranous structures called the utricle and saccule, which are known as otolith organs. These structures have patches of sensory neuroepithelium called maculae. The utricle’s macula is located in the horizontal plane and originates from the anterior wall of the sac, while the saccule’s macula is in the vertical plane and covers the bone of the vestibular inner wall. The maculae have a gelatinous otolithic membrane containing calcium carbonate crystals called otoconia. In mammals, these otoconia are arranged in layers that help the hair cells respond to the movement of endolymph. The sensory cells in the vestibular region have hair-like cilia that project from their apical end, including flexible motile kinocilia and stiff non-motile stereocilia. The stereocilia are arranged along a curvilinear line called the striola, which is an area of thickening in the saccule and thinning in the utricle. When the endolymph pressure is toward the kinocilium, it opens cation channels and allows potassium influx, resulting in the depolarization of hair cells. This depolarization leads to the release of glutamate, which activates afferent nerve receptors and facilitates neurotransmission. Signals from the vestibular nucleus are then sent to the cortex, thalamus, and cerebellum, which in turn send efferent signals to the ocular and postural muscles.
- In summary, the vestibular system, located above the cochlea in the membranous labyrinth of the inner ear, is responsible for maintaining equilibrium. It consists of the saccule, utricle, and three semicircular canals. The maculae in the otolith organs contain hair cells with stereocilia, which are stimulated by the movement of endolymph. The semicircular canals detect angular rotation through the crista ampullaris and the movement of endolymph within the canals. Together, these structures and mechanisms enable spatial orientation and contribute to our sense of balance.
b. Cochlea
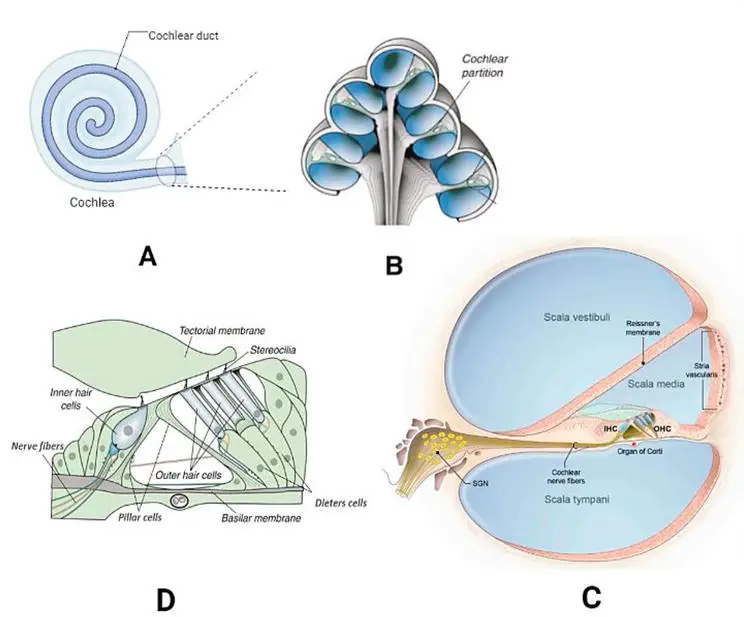
- The cochlea is a crucial component of the auditory system and plays a vital role in the process of hearing. It is a coiled, hollow, and bony structure that is lined with epithelial tissue. Its name is derived from the Greek word “kokhliās,” meaning snail, due to its distinctive spiral shape.
- The cochlea’s spiral shape is instrumental in its ability to differentiate between different frequencies of sound. Within the cochlear spiral, there are three canals lined with specialized epithelial cells. These canals are filled with fluids that contribute to the transmission and processing of sound signals.
- One of the key structures within the cochlea is the organ of Corti. This sensory organ is responsible for converting sound energy into neural signals. It contains hair cells that are vital for detecting sound vibrations. These hair cells respond to specific frequencies of sound and convert the mechanical vibrations into electrical signals. The neural signals generated by the hair cells are then transmitted through nerve fibers, such as the auditory nerve, to the brain for further processing and interpretation.
- The cochlea’s precise structure and the functioning of the organ of Corti are essential for our ability to perceive and interpret different sounds. The differentiation of frequencies along the cochlear spiral allows us to distinguish various pitches and tones, contributing to our overall auditory experience.
- In summary, the cochlea is a coiled, hollow bony structure that is lined with epithelial tissue. It plays a crucial role in the auditory system by differentiating between different frequencies of sound. The organ of Corti within the cochlea converts sound energy into neural signals, which are then transmitted to the brain for processing and interpretation.
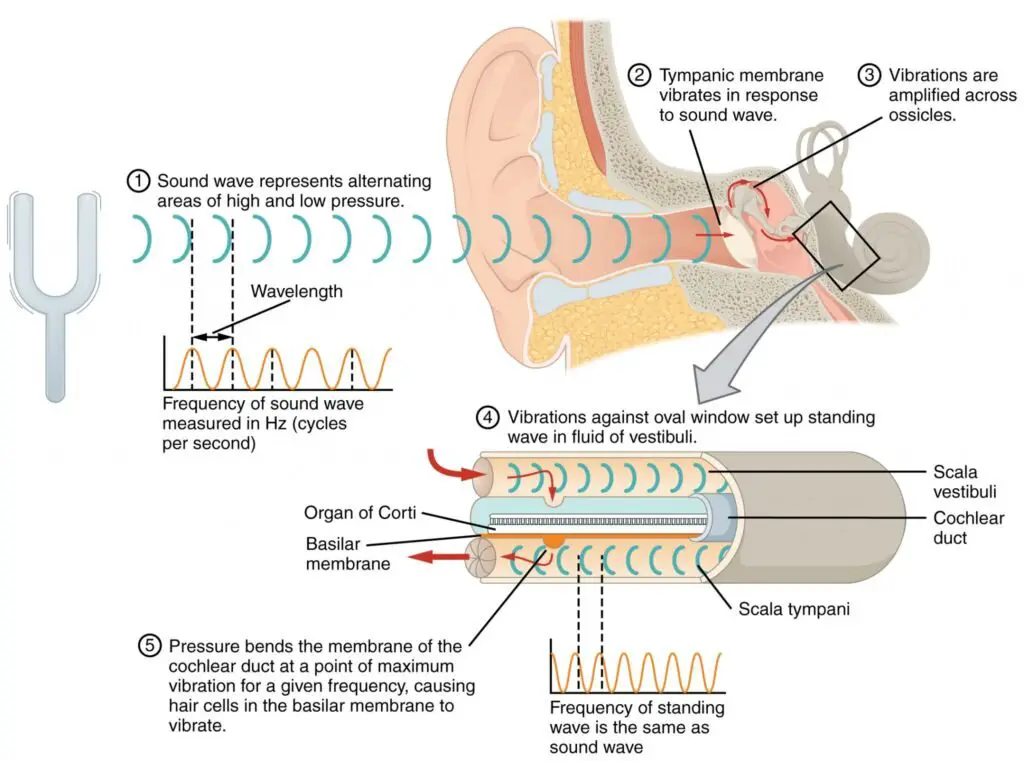
i. Canals of cochlea
The cochlea, an essential part of the inner ear responsible for hearing, consists of three canal systems: the scala vestibuli, the scala media, and the scala tympani. These canals form a spiral stairway around the modiolus, a bony axis within the cochlea.
- Scala vestibuli: The scala vestibuli is an outer canal filled with lymph and connected to the vestibules of the inner ear. At its base, it has the oval window, which receives vibrations transmitted from the middle ear via the stapes. The scala vestibuli, along with the scala tympani, detects changes in pressure caused by different sound frequencies.
- Scala tympani: The scala tympani is an inferior canal that connects to the tympanic membrane, forming the cochlea’s two-and-a-half-coiled structure. Its upper end is connected to the scala vestibuli, while its lower end separates the cochlea from the round window. The meeting point of the scala vestibuli and the scala tympani at the cochlear apex is called the helicotrema.
- Scala media: Between the scala vestibuli and the scala tympani lies the scala media. It contains the organ of Corti and the basilar membrane. The basilar membrane acts as a separation between the scala media and the scala tympani. Within the scala media, there are spiral ganglions, which are extended neurons from the hair cells. The stria vascularis of the scala media plays a role in regulating the flow of potassium ions into the scala media, thereby maintaining the endo-cochlear potential.
Together, these three canal systems and their components form the complex structure of the cochlea, allowing for the transmission and processing of sound signals, ultimately enabling us to perceive and interpret auditory information.
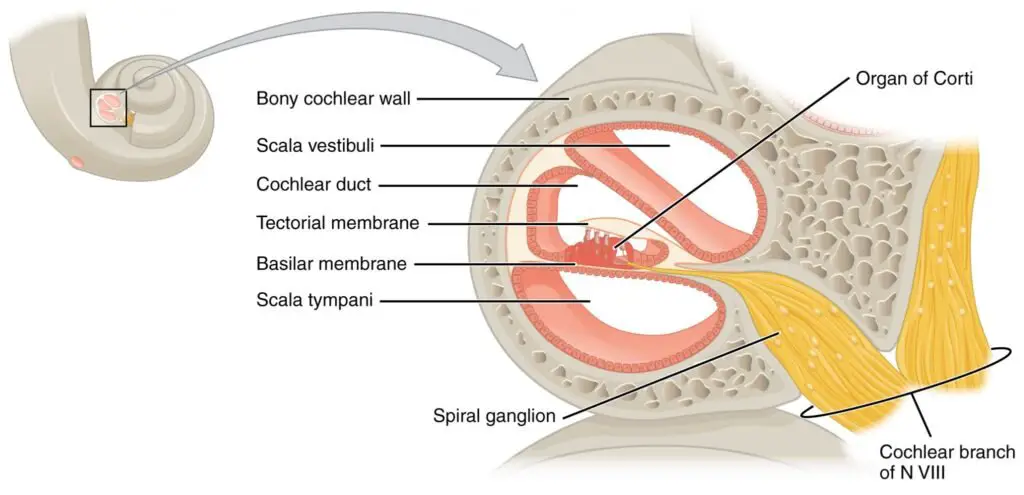
ii. Fluids of cochlea
The cochlea contains three types of fluids that fill its chambers: perilymph, endolymph, and intrastrial fluid. These fluids play crucial roles in maintaining the endo-cochlear potential and facilitating sensory transduction within the cochlea.
- Perilymph:
- Present in the scala vestibuli and scala tympani.
- Composition similar to extracellular fluid in the body.
- High concentration of sodium (140 mM) and low concentrations of calcium (1.2 mM) and potassium (5 mM).
- Perilymph in scala media is continuous with cerebrospinal fluid (CSF) while that in scala media comes from the plasma of blood.
- Endolymph:
- Present exclusively in the scala media.
- Unique ionic composition characterized by high potassium (K+) concentration (150 mM) not found elsewhere in the body.
- High concentration of K+ helps maintain the endo-cochlear potential.
- Relatively low concentrations of sodium (1 mM) and calcium (0.002 mM).
- Intrastrial fluid:
- Fills the cavities within the stria vascularis.
- Exact composition and functions not explicitly mentioned in the provided information.
Overall, the fluids of the cochlea, including perilymph and endolymph, contribute to the proper functioning of the inner ear by establishing the necessary electrochemical environment for sound transduction and signal processing.
iii. Reissner’s membrane
Reissner’s membrane is a membrane located between the scala vestibuli and scala media within the cochlea. It plays a crucial role in ion regulation and the maintenance of pressure balance between fluids. Together with the basilar membrane, Reissner’s membrane creates a cavity within the cochlear duct, which is filled with endolymph.
Here are some key points about Reissner’s membrane:
- Location and Composition:
- Positioned between the scala vestibuli and scala media.
- Consists of two types of cells: fibroblasts, which line the scala vestibuli side, and epithelial cells, which line the scala media side.
- It is an avascular membrane, meaning it lacks blood vessels.
- Role in Fluid Regulation:
- Creates a cavity within the cochlear duct, filled with endolymph.
- Plays a part in regulating ions within the cochlea.
- Maintains pressure balance between endolymph and perilymph, the fluids in the scala media and scala vestibuli, respectively.
- Presence of Ion Channels:
- Reissner’s membrane contains two types of ion channels: potassium ion channels and non-selective cation channels.
- These channels are involved in the movement of ions, particularly potassium ions, which are essential for maintaining the endo-cochlear potential.
- The ion channels on Reissner’s membrane contribute to the overall pressure regulation between endolymph and perilymph.
Reissner’s membrane, along with its role in fluid regulation and ion transport, is an integral component of the cochlea. It helps create the necessary environment for auditory transduction and contributes to the overall function of the inner ear.
iv. Organ of Corti
The organ of Corti, located on the basilar membrane, serves as the auditory organ. It comprises outer and inner hair cells, which are mechanosensory cells, along with supporting cells. The hair cells within the organ of Corti possess stereocilia that connect them to the tectorial membrane, a soft ribbon-like structure situated above it. Changes in the basilar and tectorial membranes facilitate the motion of stereocilia, leading to the stimulation of the hair cell receptors.
Tectorial membrane
The tectorial membrane is a structure that envelops the mechanosensory and supporting cells within the organ of Corti. It possesses a viscous composition comprising collagen, as well as non-collagen proteins such as glycoproteins and proteoglycans. One of the important functions of the tectorial membrane is its ability to store calcium ions for the sensory organs of the inner ear. Additionally, the stereocilia found in the organ of Corti are embedded within the tectorial membrane, further emphasizing its role in the auditory process.
Mechanosensory cells
- Hair cells: The mechanosensory cells responsible for detecting sound waves and converting them into neural signals.
- Hair cells have micro-projections called stereocilia that are filled with F-actin.
- They are arranged in the cochlea and respond to different ranges of sound frequencies.
- Tonotopy is the orderly coding of sound based on frequency by hair cells and their afferents (spiral ganglion neurons).
- Hair cells at the apex of the cochlea respond to lower frequencies, while those near the base respond to higher frequencies, creating a tonotopic gradient.
- Outer hair cells (OHCs):
- Oblong cells containing myosin and actin proteins.
- Contract in rhythmic movements in response to sound stimuli.
- Approximately 12,000 OHCs arranged in three rows.
- Stereocilia of OHCs are embedded in the tectorial membrane.
- OHCs play a role in mechanoelectrical stimulation and amplification of low-frequency sounds.
- Possess a unique motor protein called prestin involved in the active amplifier mechanism, amplifying weak sounds.
- Inner hair cells (IHCs):
- Primary organ for auditory sensation.
- Pear-shaped cells with stereocilia weakly connected to the tectorial membrane.
- Approximately 3500 IHCs arranged in a single row, surrounded by supporting cells.
- Transmit electrical signals to the auditory cortex of the brain through nerve fibers.
- About 95% of auditory nerve projection to the brain is through IHCs.
- Outer hair cells assist IHCs in generating synaptic nerve conduction to cochlear nerve fibers.
- Supporting cells:
- Rigid sensory epithelial cells organized in a mosaic pattern.
- Maintain the integrity of sensory hair cells during head movement and sound stimulation.
- Different types of supporting cells include Hensen’s cells, Deiters’ cells, pillar cells, Claudius cells, Boettcher cells, and border cells.
- Arranged from the outer edge to the inner edge of the organ of Corti.
- Play a vital role in maintaining the microenvironment for proper hair cell function.
- Maintain the structure of the organ of Corti and composition of the endolymph in the scala media.
- Supporting cells have a negative resting potential and transport Na+ out and K+ into the scala media through channels.
v. Mechanotransduction in cochlea
- Mechanotransduction is a crucial process that occurs in the cochlea, the auditory organ of the inner ear, responsible for converting sound waves into electrical signals that can be interpreted by the brain. This intricate process relies on the mechanosensitive hair cells within the cochlea, which detect and transduce mechanical vibrations into electrical signals.
- The key players in mechanotransduction within the cochlea are the stereocilia, which are tiny hair-like structures present on the surface of the hair cells. The stereocilia are arranged in rows, with their heights progressively increasing. These rows of stereocilia are interconnected by specialized structures called tip links. The tip links consist of proteins that connect the upper and lower regions of adjacent stereocilia, forming a delicate and precise mechanical linkage (Figure 4).
- In the resting state, a small number of transduction channels located at the tips of the stereocilia are open. These transduction channels allow for the flow of ions, including potassium (K+), between the extracellular and intracellular environments of the hair cells. When sound waves enter the ear and create vibrations, the cochlear fluids and tissues move. This movement causes the tectorial membrane, which overlies the hair cells, to displace.
- As the tectorial membrane moves, it exerts a force on the stereocilia, causing them to bend. The bending of the stereocilia, particularly the largest one embedded in the tectorial membrane, leads to the displacement of the shorter stereocilia. This mechanical deflection of the stereocilia opens up additional transduction channels at their tips, allowing for an influx of potassium ions from the surrounding fluids.
- The entry of potassium ions into the hair cells leads to depolarization, a change in the electrical potential across the cell membrane. This depolarization triggers the opening of voltage-gated calcium ion channels. Calcium ions (Ca2+) flow into the hair cells, initiating a cascade of intracellular events. The influx of calcium ions plays a vital role in the release of neurotransmitters, such as glutamate, from the hair cells.
- Glutamate, the primary neurotransmitter in the auditory system, is released from the hair cells into the synaptic cleft, where it binds to receptors on the auditory nerve fibers. This binding initiates a series of electrical impulses, which are then transmitted along the auditory nerves to the brain. These electrical signals travel through various auditory processing centers in the brain, ultimately allowing us to perceive and interpret sound.
- The cochlea is a finely tuned structure, and the regulation of its extracellular ionic environment is crucial for the accurate conversion of sound signals into electrical impulses. The apical and basal regions of the cochlea are separated by membranes that help maintain distinct ionic compositions. This regulation ensures that the mechanotransduction process remains sensitive and efficient, enabling the precise encoding of sound information.
- In summary, mechanotransduction in the cochlea relies on the deflection of stereocilia caused by the movement of the tectorial membrane. This deflection opens transduction channels, leading to the influx of potassium ions, depolarization of the hair cells, and subsequent release of neurotransmitters. These events ultimately result in the transmission of sound signals to the brain, allowing us to perceive and interpret auditory stimuli.
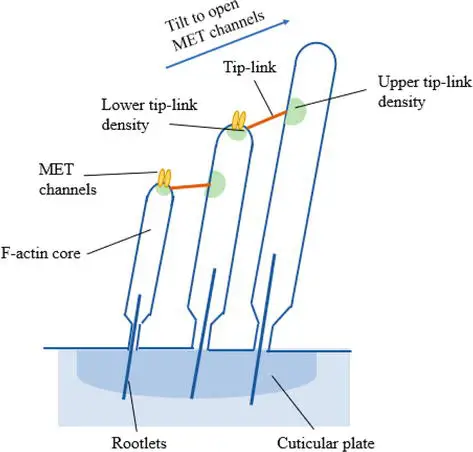
Mechanism of Maintaining Equilibrium
- The maintenance of equilibrium, or the sense of balance, relies on the intricate mechanisms within the inner ear. The vestibule of the inner ear houses specialized mechanoreceptors called hair cells, which are responsible for sensing head position, head movement, and the body’s motion. The information detected by these hair cells is essential for maintaining our balance.
- The utricle and saccule, located within the vestibule, sense head position. They are primarily composed of maculae, which are patches of specialized tissue. Each macula contains hair cells surrounded by support cells. The hair cells in the macula have stereocilia that extend into a gel-like substance called the otolith, which contains calcium carbonate crystals. The otolith is denser and more inert than the surrounding macula.
- When the head is tilted, gravity causes the otolith to move independently of the macula in response to the movement. The otolith slides over the macula in the direction of gravity. This movement of the otolith layer bends the stereocilia of the hair cells, resulting in the depolarization of some hair cells and the hyperpolarization of others. The specific pattern of hair cell depolarization is interpreted by the brain to determine the exact tilt of the head.
- In addition to head position, the semicircular canals play a crucial role in sensing head movement. There are three semicircular canals: one oriented in the horizontal plane and two oriented in the vertical plane. Each semicircular canal connects to an enlarged region called the ampulla, which contains hair cells responsible for detecting rotational movement.
- The stereocilia of these hair cells extend into a structure called the cupula, which is a membrane attached to the top of the ampulla. As the head rotates in a plane parallel to the semicircular canal, the fluid within the canal lags behind, causing the cupula to bend in the opposite direction to the head movement. The deflection of the cupula by the fluid movement stimulates the hair cells, generating neural signals.
- The semicircular canals contain multiple ampullae, some oriented horizontally and others vertically. By comparing the movements of both the horizontal and vertical ampullae, the vestibular system can detect the direction of most head movements within three-dimensional space. This allows for the precise perception of rotational movements in various planes.
- The neural signals generated by the hair cells in the vestibule and semicircular canals are transmitted through the vestibulocochlear nerve to the brain stem and cerebellum, where they are processed and integrated with other sensory information. This information contributes to our overall sense of balance and helps coordinate motor responses to maintain stability and posture.
- In summary, the maintenance of equilibrium relies on the sensory input from hair cells within the inner ear. The utricle and saccule sense head position, while the semicircular canals detect head movement. The movements of the otolith and cupula, in response to changes in head position and movement, respectively, stimulate the hair cells and generate neural signals that are interpreted by the brain to maintain a sense of balance and coordinate motor responses.
Sound Wave Transmission and its Physics
- Sound wave transmission and its physics involve the process of converting external sound vibrations into electrical signals that can be interpreted by the brain. Sound is produced when objects vibrate, creating pressure pulses in the surrounding air molecules, forming sound waves. The ear plays a crucial role in distinguishing various characteristics of sound, such as pitch and loudness.
- Pitch refers to the frequency of sound waves, measured in hertz (Hz), which represents the number of cycles per second. The human ear can detect frequencies ranging from 1000 to 4000 Hz, while a young ear can hear frequencies between 20 and 20,000 Hz. On the other hand, loudness refers to the intensity of sound and is measured in decibels (dB). The range of human hearing on a decibel scale extends from 0 to 130 dB, beyond which sound becomes painful.
- To transmit sound waves into the central nervous system, a series of transformations occur. The initial transformation involves the conversion of air vibrations into vibrations of the tympanic membrane (eardrum). These vibrations are then transmitted to the middle ear, where they are amplified by the ossicles (small bones) located within.
- Next, the vibrations are transformed into liquid vibrations within the inner ear, particularly in the cochlea. The cochlea contains the basilar membrane and the organ of Corti, which play essential roles in sound perception. The vibrations stimulate the basilar membrane and the hair cells in the organ of Corti.
- Finally, these vibrations are converted into nerve impulses, which travel through the auditory nerve to the central nervous system for further processing and interpretation. This intricate process of converting sound waves into electrical signals allows us to perceive and understand the sounds around us.
- Understanding the physics of sound wave transmission is crucial for comprehending how the ear functions and how sound is processed by the auditory system.
Sound Conducting Mechanisms
The Outer Ear
- The outer ear plays a crucial role in transmitting sound to the middle ear. Comprising of the pinna and the ear canal, it collects and channels sound waves towards the tympanic membrane, commonly known as the eardrum.
- Starting with the pinna, which is the visible part of the outer ear, it protrudes from the side of the skull and is made of cartilage covered by skin. The unique shape of the pinna allows it to capture sounds coming from the front more effectively than those from behind, contributing to sound localization. However, this effect is most pronounced at higher frequencies due to the relative size of the head and the wavelength of audible sound. In the middle frequencies, the head itself creates a sound shadow, while in lower frequencies, the phase of sound arrival between the ears aids in sound localization.
- Moving into the ear canal, it extends approximately 4 centimeters in length and consists of an outer and inner part. The outer portion is lined with hairy skin containing sweat glands and oily sebaceous glands, which produce earwax. The hair and earwax act as a protective barrier and disinfectant. As the ear canal progresses inward, the skin becomes thinner and simpler, firmly attaching to the bone of the deeper ear canal. This bony cavity absorbs little sound but guides it towards the tympanic membrane at its base.
- The tympanic membrane, also referred to as the eardrum, separates the ear canal from the middle ear and serves as the initial component of the sound transducing mechanism. It has a shape resembling a loudspeaker cone, which is ideal for transmitting sound between solids and air. The membrane is remarkably thin, measuring less than a tenth of a millimeter in thickness. It consists of a delicate outer layer of skin, a thin lining membrane of respiratory epithelium on the inner surface, and a stiffening fibrous middle layer. The tympanic membrane covers a round opening, about 1 centimeter in diameter, leading to the middle ear cavity.
- It’s worth noting that although the term “ear drum” is commonly used to refer to the tympanic membrane, technically, the entire middle ear space can be considered the drum, with the tympanic membrane functioning as the drum skin.
- To maintain the health and functionality of the outer ear, it’s important not to insert cotton buds or other objects into the ear canal. The shedding of skin in the outer part of the canal, combined with the production of earwax, naturally cleans the ear. Using cotton buds can inadvertently push the shed skin and wax deeper into the canal, leading to impaction and hearing obstruction.
- In summary, the outer ear, composed of the pinna, ear canal, and tympanic membrane, collects and directs sound waves towards the middle ear. Each component of the outer ear has specific functions that contribute to sound localization, protection, and the transduction of sound into the middle ear.
The Middle Ear
- The middle ear serves as an air-filled space that connects to the back of the nose through a slender tube called the Eustachian tube. Within the middle ear, three small bones known as the hammer, anvil, and stirrup (malleus, incus, and stapes) play a crucial role in conducting sound from the tympanic membrane to the inner ear. The outer wall of the middle ear is formed by the tympanic membrane, while the inner wall is connected to the cochlea.
- The upper boundary of the middle ear is formed by the bone beneath the middle lobe of the brain, and the floor of the middle ear covers the beginning of the jugular bulb, which is responsible for draining blood from the head. Positioned at the front of the middle ear is the opening of the Eustachian tube, while a passageway at the posterior end leads to a group of air cells within the temporal bone called the mastoid air cells. One can envision the middle ear space as resembling a frying pan on its side, with a handle pointing downwards and forwards (representing the Eustachian tube), and a hole in the back wall leading to a spongy bone containing multiple air cells (the mastoid air cells).
- The middle ear is an extension of the respiratory air spaces found in the nose and sinuses, and it is lined with a respiratory membrane. The thickness of the respiratory membrane varies, being thicker near the Eustachian tube and becoming thinner as it transitions into the mastoid region. The middle ear also possesses the ability to secrete mucus. The Eustachian tube starts off as a bony structure within the ear but gradually transforms into cartilage and muscle as it approaches the back end of the nose, specifically in the nasopharynx. Muscular contractions actively open the Eustachian tube, enabling equalization of air pressure between the middle ear and the nose.
- Sound transmission from the tympanic membrane to the inner ear is facilitated by the three aforementioned bones: the malleus, incus, and stapes. The malleus resembles a club, with its handle embedded in the tympanic membrane and extending upward from its center. The head of the malleus is situated in a cavity of the middle ear called the attic, where it is suspended by a ligament from the bone covering the brain. In the attic, the head of the malleus articulates with the cone-shaped incus. The incus extends backward from the malleus and features a small, thin projection known as the long process, which hangs freely in the middle ear. At the tip of the long process, the incus forms a right-angle bend and connects to the stapes (or stirrup), the third bone in the chain. The stapes has an arched shape and a footplate that covers the oval window—an opening into the vestibule of the inner ear or cochlea. The stapes articulates with the cochlea through the stapedio-vestibular joint.
- Together, these components of the middle ear form a crucial pathway for sound conduction, transmitting vibrations from the tympanic membrane to the inner ear, where sound is further processed and perceived.
The Sound Transducing Mechanism – The Inner Ear
- The inner ear, specifically the bony cochlea, derives its name from its resemblance to a snail shell. It consists of two and a half turns and houses the membranous labyrinth, which is the organ of hearing. Surrounding the membranous labyrinth is a fluid called perilymph. The cochlea has a volume of approximately 0.2 milliliters and contains up to 30,000 hair cells responsible for transducing vibrations into nervous impulses, as well as about 19,000 nerve fibers that transmit signals to and from the brain.
- To understand the structure of the membranous labyrinth, one can imagine the cochlea as a straightened bony tube that is closed at the apex and open at the base, with connections to the round and oval windows and a link to the vestibular labyrinth. The vestibular labyrinth, or the organ of balance, acts as both a linear and angular accelerometer, providing information to the brain about the position of the head in relation to gravity and the surroundings. However, we will focus solely on the sound transducing mechanism within the cochlea.
- When the footplate of the stapes vibrates, it sets the perilymph in the bony cochlea into motion. Since the perilymph is essentially incompressible, a counter opening is necessary within the labyrinth to allow the fluid space to expand when the footplate moves inward and vice versa. This counter opening is provided by the round window membrane, which lies beneath the oval window on the inner wall of the middle ear. The round window membrane is covered by a fibrous membrane that moves in synchrony but in opposite phase to the footplate of the oval window.
- The membranous labyrinth within the cochlea is divided into three sections by a membranous sac of triangular cross-section that runs along the length of the cochlea. The two outer sections are known as the scala vestibuli, which is connected to the oval window, and the scala tympani, which is connected to the round window. These sections are filled with perilymph and connect at the apex through a small opening called the helicotrema, which serves as a pressure equalizing mechanism at frequencies below the audible range. They also connect with the fluid surrounding the brain at the vestibular end through a small channel called the perilymphatic aqueduct. The membranous labyrinth, also referred to as the cochlear duct, is filled with a different fluid called endolymph. It is separated from the scala vestibuli by Reissner’s membrane and from the scala tympani by the basilar membrane.
- The basilar membrane is composed of numerous taut, radially parallel fibers sealed between a gelatinous material with weak shear strength. These fibers have progressively lower resonant frequencies as one moves from the basal to the apical ends of the cochlea. Positioned on top of the basilar membrane are four rows of hair cells, along with supporting cells. The single inner row is located medially, closest to the central core of the cochlea, and it has a rich nerve supply that carries messages to the brain. The three outer rows, which mainly receive afferent nerve supply, are separated from the inner row by tunnel cells, forming a stiff triangular structure known as the tunnel of Corti. Any natural displacement of the cochlear partition results in a rocking motion of the tunnel of Corti, causing a lateral displacement of the inner hair cells.
- The hair cells derive their name from the presence of stereocilia at their free ends. Stereocilia are tiny, stiff hair-like structures, a few micrometers in length. The stereocilia of the hair cells are arranged in rows within a narrow cleft called the subtectorial space, which is formed by the presence of the radially stiff tectorial membrane above the hair cells. The cilia of the outer hair cells are firmly attached to the tectorial membrane, while the cilia of the inner hair cells are either free-standing or loosely attached.
- In summary, the inner ear serves as the sound transducing mechanism, converting vibrations transmitted through the perilymph via the ossicular chain into a nervous impulse that is then carried to the brain, where it is perceived as sound. The inner hair cells play a crucial role in this process, as they transduce the vibration into a nervous impulse. The basilar membrane acts as an efficient frequency analyzer, responding resonantly to different frequencies along its length. The cochlea has an abundant nerve supply, both afferent fibers transmitting signals from the cochlea to the brain and efferent fibers conveying impulses from the brain to the cochlea. The active feedback loop involving the outer hair cells helps maintain the displacement of stereocilia within acceptable limits.
The Physiology Of Hearing (How Does This All Work?)
The Outer and Middle Ears
- The physiology of hearing involves both the outer and middle ears, which contribute to the amplification of sound signals before reaching the inner ear.
- The audible range of sound spans approximately 10 octaves, from around 16 to 32 Hz up to 16,000 to 20,000 Hz. Sensitivity to sound is lower at the extremes but becomes more pronounced between 128 Hz and 4,000 Hz, gradually diminishing with age. The head itself acts as a natural barrier between the two ears, causing a sound source on one side to stimulate the ear nearest to it more intensely and arrive there sooner. This mechanism aids in sound localization based on differences in intensity and time of arrival. High-frequency hearing is particularly important for sound localization, explaining why it becomes challenging when there is a high-frequency hearing loss. Although the human head is relatively large compared to the size of the pinna, the crinkled shape of the pinna catches higher-frequency sounds and directs them into the ear canal. Additionally, it helps block higher-frequency sounds from behind, assisting in identifying whether the sound is coming from the front or back.
- The ear canal functions as a resonating tube, amplifying sounds between 3,000 and 4,000 Hz, thereby increasing sensitivity (and vulnerability to damage) to sounds within this frequency range. The ear is highly sensitive and can respond to sounds of very low intensity, even vibrations only slightly greater than the natural random movement of air molecules. To achieve this, the air pressure on both sides of the tympanic membrane must be equal. This is evident to anyone who experiences their ear being blocked, such as during a rapid elevator ride. The Eustachian tube plays a vital role in pressure equalization. It periodically opens, usually every 3rd or 4th swallow, to equalize the pressure. If the Eustachian tube were open all the time, one would hear their own breath continuously. The lining membrane of the middle ear acts as a respiratory membrane and can absorb gases. If the Eustachian tube remains closed for an extended period, it can lead to the absorption of carbon dioxide and oxygen from the air in the middle ear, resulting in negative pressure. This can cause pain, as experienced during descent in an airplane if the Eustachian tube is not unblocked. The middle ear cavity itself is relatively small, and the mastoid air cells serve as an air reservoir, cushioning the effects of pressure changes. Prolonged negative pressure can lead to the secretion of fluid in the middle ear, resulting in conductive hearing loss.
- The outer and middle ears work together to amplify the sound signal. The pinna, with its larger surface area, funnels sound to the smaller tympanic membrane. The surface area of the tympanic membrane is larger than that of the stapes footplate, resulting in hydraulic amplification. This means that a small movement over a large area is converted into a larger movement over a smaller area. Furthermore, the ossicular chain, which consists of the malleus, incus, and stapes, acts as a system of levers, further amplifying the sound. Collectively, the outer and middle ears amplify sound by approximately 30 dB as it travels from the external environment to the inner ear.
The Inner Ear
The inner ear plays a crucial role in the physiology of hearing by transducing vibrations into nervous impulses and analyzing the frequency (pitch) and intensity (loudness) of sound. Nerve fibers in the inner ear can fire at a rate of nearly 200 times per second. The rate of nerve firing conveys information about sound level to the brain, with groups of nerves firing at rates below 200 pulses per second. These nerve fibers can also fire in synchronization with acoustic signals up to around 5 kHz. Below 5 kHz, groups of nerve fibers firing in phase with an acoustic signal provide frequency information to the brain. However, above 5 kHz, frequency information is based on the place of stimulation along the basilar membrane. It is worth noting that music translated into the frequency range above 5 kHz may not sound musical.
The basilar membrane in the inner ear responds differently to various frequencies. Each point along the length of the basilar membrane has its characteristic frequency, with the highest frequency response at the basal end and the lowest at the apical end. When sound is introduced at the oval window through the motion of the stapes, it travels along the basilar membrane as a traveling wave. Each frequency component of the sound wave reaches its corresponding place of resonance on the basilar membrane and stops traveling further. For example, a 1 kHz tone induces resonance around the middle of the basilar membrane. Frequencies lower than 1 kHz must travel more than half the length of the basilar membrane, while frequencies above 1 kHz travel less than half. As the traveling wave passes through regions of high-frequency resonant response, the brain suppresses high-frequency information in favor of low-frequency information. This explains why low-frequency sounds, such as traffic noise, can effectively mask high-frequency sounds like the fricatives of speech, making it challenging to use telephones near busy streets.
Regarding intensity, the physiological range of intensity in the normal ear is vast, similar to the range of the eye when considering the combined responses of cones and rods. It spans from perceiving the flickering of a candle at a distance of 100 meters on a dark night to looking indirectly at a bright sun. This wide range is best encompassed by the logarithmic response characteristic of variable rate processes, which is favored by anatomical systems. The normal range of human hearing is from 0 to 100 dB(A) before sound becomes uncomfortably loud.
In the inner ear, mounted on the basilar membrane near its central core, are a single row of inner hair cells followed by three rows of outer hair cells, separated by the tunnel of Corti. Any natural displacement of the cochlear partition causes the tunnel of Corti to rock, resulting in lateral displacement of the inner hair cells. Only the inner hair cells initiate nervous impulses that are perceived as sound. While they are not particularly sensitive, they are located at the inner edge of the relatively immobile basilar membrane. The point of most vigorous vibration along the basilar membrane is around its middle, minimizing the intense vibration experienced by the inner hair cells. The question arises: How do the inner hair cells respond to slight or moderate stimulation? This is where the outer hair cells play a major role. When stimulated by the traveling wave, the outer hair cells actively and physically contract. With muscle proteins in their walls, they shorten, producing an additional shear movement of the membranous labyrinth due to their attachment to both Reissner’s membrane and the basilar membrane. This amplifies the traveling wave at the point of maximal stimulation, and the amplified movement is transmitted to the inner hair cells, prompting their response.
If the movement of the basilar membrane is slight, the contracture of the outer hair cells significantly adds to the movement of the basilar cells. However, if the movement is already large, the contracture adds nothing to the substantial displacement of the membranous labyrinth. If the outer hair cells are damaged, they no longer contract in response to slight sounds, and the inner hair cells are not stimulated. This leads to a hearing loss for low-intensity sounds. However, for more intense sounds, the inner hair cells are stimulated directly and respond normally, preserving the ability to hear louder sounds.
This phenomenon is known as loudness recruitment. Inner hair cells are more resilient than outer hair cells and less prone to damage from aging, noise exposure, or most ototoxic drugs. Therefore, hearing loss typically occurs but not deafness in cases of aging, noise exposure, or ototoxic drugs. It is noteworthy that the ear’s sensitivity to sounds is highest between approximately 3000 and 4000 Hz, partly due to the amplification mechanism of the ear canal. Consequently, the most intense stimuli occur at these frequencies, posing the greatest risk of damage to the outer hair cells that respond to them. Prolonged exposure to loud sounds can lead to the impairment of these hair cells, explaining the hearing loss that commonly occurs first at 3 to 4 kHz.
Central Auditory Processing
Central auditory processing refers to the complex neural mechanisms involved in the transmission and interpretation of auditory information within the central nervous system. After the nervous impulses are carried along the 8th cranial nerve, also known as the vestibulocochlear nerve or the statico-acoustic nerve, from the cochlea to the brainstem, they reach nuclei where they relay with other nerve fibers. These nuclei are responsible for various processing tasks, such as extracting different features of sound, analyzing spatial location, and integrating auditory information with other sensory inputs.
The fibers from each auditory nerve split, with some passing to the contralateral (opposite) side of the brain and others remaining on the same side. This bilateral pathway allows for the integration of auditory inputs from both ears, enabling sound localization and the perception of binaural cues. Consequently, unilateral hearing loss cannot be caused solely by a brain lesion since auditory information is processed on both sides of the brain.
As the fibers ascend from the brainstem, they pass through the hindbrain to the midbrain and eventually reach the cerebral cortex. The auditory cortex, located in the temporal lobes of the brain, is responsible for higher-level auditory processing. Here, auditory information undergoes further analysis, interpretation, and integration with other cognitive functions.
Central auditory processing involves numerous functions, many of which extend beyond the scope of a single chapter. However, some key aspects can be explored. These include the perception of pitch, temporal aspects of sound, sound localization, auditory discrimination, speech processing, and the integration of auditory information with other sensory modalities. The central auditory system works in conjunction with other brain regions to create a comprehensive auditory experience and to support communication, learning, and interpretation of the auditory environment.
Disorders or impairments in central auditory processing can result in difficulties with sound localization, auditory discrimination, speech understanding, and auditory memory. Conditions such as auditory processing disorder (APD) may affect the ability to process and interpret auditory information accurately, even when hearing sensitivity is normal. Diagnosis and management of central auditory processing disorders involve comprehensive assessments and targeted interventions to improve auditory processing abilities and facilitate effective communication.
The Ability to Block Out Unwanted Sounds.
- The ability to block out unwanted sounds, often referred to as selective attention or the cocktail party effect, is a remarkable cognitive process that allows individuals to focus on a specific sound source or conversation while filtering out competing background noise. This ability relies on a combination of peripheral hearing, binaural hearing, and central auditory processing mechanisms.
- In a crowded and noisy environment, a person with normal hearing can selectively tune in and out of conversations at will. This feat is made possible by the brain’s automatic adjustment of time of arrival and intensity differences of sound from different sources. The brain processes the auditory inputs received from both ears and employs feedback loops to determine which signals should be passed on to the auditory cortex for further processing, while suppressing or attenuating irrelevant or unwanted sounds that do not meet certain criteria.
- For this process to be effective, several factors come into play. Good high-frequency peripheral hearing is essential as it allows the detection of subtle acoustic cues and the ability to perceive and differentiate speech sounds in noisy environments. Binaural hearing, having two ears, provides important cues for sound localization and helps in distinguishing sounds coming from different directions.
- Additionally, there is an intricate central mechanism involved in the selective attention process. This mechanism involves the integration and analysis of auditory information within the central auditory pathways and cognitive systems. The brain’s ability to process and prioritize certain sound sources relies on neural networks that evaluate temporal and spectral cues, comparing them with stored representations of familiar sounds and linguistic patterns. Through this complex system, the brain can allocate attention to the desired sound source while suppressing or inhibiting competing sounds.
- However, with age, there can be a decline in the central mechanisms that support selective attention. The elderly may find it more challenging to listen in crowded rooms due to a diminished ability to filter out background noise and focus on specific conversations. This decline is often compounded by any underlying hearing loss, which further compromises the perception of speech in noisy environments.
- In conclusion, the ability to block out unwanted sounds and selectively attend to specific auditory stimuli is a remarkable feat accomplished by the human auditory system. It relies on a combination of peripheral hearing, binaural hearing, and complex central auditory processing mechanisms. Understanding the factors that contribute to selective attention can help inform strategies for improving communication in challenging listening situations and addressing difficulties experienced by individuals with hearing loss or age-related changes in auditory processing.
Spatial Localization
- Spatial localization refers to the ability of a normal human to accurately determine the direction or source of a sound. It is a fundamental aspect of auditory perception that allows us to locate where a sound is coming from and orient ourselves accordingly. Whether it’s identifying the direction of a speaker’s voice, pinpointing the location of an approaching car, or detecting the position of a bird in flight, our auditory system plays a crucial role in spatially localizing sounds.
- The process of spatial localization involves specialized neurons in the midbrain, particularly in the superior colliculus and inferior colliculus. These regions of the midbrain receive inputs from both ears and are responsible for integrating auditory information with other sensory inputs, such as visual and proprioceptive cues, to create a spatial map of the environment.
- One of the key mechanisms involved in spatial localization is binaural hearing, which takes advantage of the differences in time of arrival and intensity of sounds between the two ears. These differences, known as interaural time differences (ITDs) and interaural level differences (ILDs), provide important cues for localizing sounds in the horizontal plane. When a sound source is located to one side, it reaches the ear closest to it slightly earlier and with higher intensity compared to the other ear. The brain processes these subtle differences and uses them to determine the direction of the sound source.
- In addition to binaural cues, other cues, such as spectral cues and head-related transfer functions, contribute to spatial localization. Spectral cues arise from the way sounds interact with the shape of the outer ear, or pinna, and the head. These cues provide information about the elevation or vertical position of a sound source. The unique filtering properties of the outer ear help us discern whether a sound is coming from above, below, or at the same level as our ears.
- The neural processing of spatial localization occurs in a hierarchical manner. Information from the ears is first processed in the brainstem, where it undergoes initial computations of ITDs and ILDs. This information is then relayed to the midbrain and further refined in the auditory cortex. The auditory cortex integrates the binaural and spectral cues, as well as other multisensory inputs, to create a coherent representation of sound location.
- Through this complex neural processing, we are able to perceive and interpret the spatial characteristics of sounds in our environment. Spatial localization is an essential ability that allows us to navigate our surroundings, react to potential threats, and engage in effective communication.
- Understanding the mechanisms of spatial localization can have practical implications in fields such as audiology and audio engineering. It can inform the design of hearing aids and assistive listening devices that enhance spatial perception for individuals with hearing impairments. Additionally, in audio engineering, knowledge of spatial localization can contribute to the creation of immersive and realistic sound experiences in various applications, such as virtual reality, gaming, and audio production.
On and Off Sounds
- In the realm of auditory perception, our hearing serves an alerting function, particularly when it comes to detecting and responding to changes in our acoustic environment. The human auditory system contains specialized cells in the brain that are specifically attuned to the onset and offset of sounds. These cells play a crucial role in our ability to notice and adapt to acoustic changes, while also allowing us to become accustomed to steady or constant sounds over time.
- When a sound suddenly begins or “turns on,” dedicated neurons in the brain respond to this change and generate a response. This acute sensitivity to sound onset helps us direct our attention toward new or potentially significant auditory events. For instance, imagine being in a quiet room when a door slams shut. The abrupt onset of the sound immediately captures your attention and triggers a response. Similarly, warning signals, such as sirens or alarms, are designed to exploit our sensitivity to sound onset, effectively alerting us to potential dangers or urgent situations.
- Conversely, there are brain cells that are specifically attuned to sound offsets or the cessation of sound. These cells detect changes in the auditory environment when a sound abruptly ends or “switches off.” This ability to detect the absence of sound is equally important, as it helps us perceive changes in our surroundings. Returning to the example of an air-conditioned room, when the air conditioner turns off, the sudden absence of its noise is noticeable, at least for a brief period. Our brains register this change in the auditory stimulus, allowing us to adapt and become aware of the new acoustic state.
- The phenomenon of adjusting to constant sounds while remaining sensitive to changes is known as auditory adaptation or habituation. Our auditory system is designed to filter out or ignore continuous, unchanging sounds that are deemed non-threatening or irrelevant to our current focus. This adaptive mechanism enables us to direct our attention to new or more salient auditory stimuli while filtering out background noise or steady sounds that have become familiar or unimportant.
- This ability to discern changes in our auditory environment extends beyond everyday situations and applies to various contexts. In industrial settings, for example, trained workers develop a keen ear for detecting changes in machinery sounds. They can identify irregularities or malfunctions by noticing alterations in the usual patterns of sounds produced by the equipment. The brain’s sensitivity to acoustic changes helps individuals remain vigilant and respond promptly to potential issues or safety concerns.
- Overall, the existence of neurons that respond specifically to sound onsets and offsets allows our auditory system to be finely tuned to changes in our acoustic environment. By promptly detecting and adapting to these changes, we can effectively navigate our surroundings, respond to warning signals, and remain attuned to potential auditory cues that may impact our well-being or require our attention.
Interaction of Sound Stimuli with Other Parts of the Brain
- When sound stimuli reach our ears, they initiate a complex interaction with various parts of the brain, resulting in a range of appropriate responses and emotional experiences. This integration of auditory information with other brain regions allows us to interpret and react to sounds in ways that are specific to the context and our individual experiences.
- For instance, a warning signal or a sudden loud noise triggers an immediate and instinctive reaction throughout the body. The brain rapidly processes the sound, triggering a general response aimed at ensuring our safety. This can include an increased heart rate, heightened muscle tension, and a readiness to move or take evasive action. This automatic response helps us prepare for potential threats and facilitates our survival.
- The power of sound to evoke specific responses is exemplified in the unique bond between a mother and her baby. A mother’s brain is attuned to her baby’s cry in a distinct way, triggering an immediate and strong alertness. The sound of her baby’s cry activates regions of the brain associated with maternal instincts, nurturing behaviors, and emotional attachment. This response is specialized and may not be replicated in the same way for others who hear the baby’s cry.
- Similarly, the influence of sound on our emotional state and behavior is evident in various contexts. The sound of martial music, for instance, can evoke a sense of patriotism, camaraderie, or excitement in individuals who identify with it. It may lead to physical responses such as an increase in energy levels or a bracing movement. On the other hand, if played with hostile intent, martial music can instill fear and anxiety in the hearts and minds of those targeted by it. This demonstrates how sound stimuli can elicit different emotional states and shape our reactions based on our personal associations, cultural background, and past experiences.
- The impact of sound on our emotions extends beyond specific examples. Various sounds can evoke anger, pleasure, happiness, sadness, or nostalgia, among other emotional states. These responses are not solely limited to the auditory experience but are intricately intertwined with the body’s overall physiological and psychological milieu. Sound sensations are integrated within the central nervous system, becoming part of our holistic experience and shaping our perception of the world around us.
- Furthermore, the interaction of sound stimuli with other parts of the brain goes beyond emotional and physical responses. Sound can also influence cognitive processes, memory formation, and language comprehension. For example, certain sounds or melodies can evoke memories or trigger associations with specific events or places. Music has been shown to have a profound impact on mood regulation, motivation, and cognitive performance.
- In summary, the interaction between sound stimuli and other regions of the brain is a multifaceted process. Sound not only alerts us to potential dangers but also evokes emotions, triggers memories, and influences our cognitive and behavioral responses. These intricate connections within the central nervous system make sound an integral part of our overall perception, shaping our experiences and interactions with the world.
Mechanism of Hearing (Physiology of hearing) – Summery
The mechanism of hearing is a complex process that involves various structures and physiological events working together to enable us to perceive sound. Let’s break down the steps involved in the mechanism of hearing:
- Sound Reception: The process begins with the pinna, the visible part of the ear, which receives sound waves from the environment. The sound waves travel through the external auditory meatus (ear canal) and reach the tympanic membrane (eardrum).
- Sound Amplification: When the sound waves strike the tympanic membrane, it starts to vibrate. These vibrations are transmitted to the three small bones in the middle ear called ossicles: the malleus (hammer), incus (anvil), and stapes (stirrup). The ossicles amplify the sound waves as they pass from the eardrum to the inner ear.
- Transfer of Vibrations: The stapes, the last of the ossicles, transmits the amplified vibrations to the oval window, a membrane-covered opening in the cochlea, which is part of the inner ear. The vibrations pass from the middle ear into the fluid-filled inner ear.
- Fluid Movement: The vibrations cause pressure waves in the fluid of the inner ear. The pressure waves move through the scala vestibuli, a fluid-filled chamber in the cochlea, and eventually reach the endolymph of the scala media (cochlear duct). This movement of fluid is facilitated by the round window, which allows the dissipation of fluid motion in the scala tympani.
- Stimulation of Hair Cells: Within the scala media, the basilar membrane runs along its length. As the pressure waves travel through the fluid, the basilar membrane vibrates. This causes the hair-like projections called stereocilia, which are located on the hair cells of the organ of Corti (a structure on the basilar membrane), to rub against the tectorial membrane.
- Generation of Neural Signals: The bending of stereocilia opens ion channels in the plasma membrane of hair cells. This allows ions, including calcium ions (Ca++), to enter the hair cells, resulting in the release of neurotransmitters, such as glutamate. The released neurotransmitters bind to receptors on afferent neurons (nerve fibers), which form synapses with the hair cells.
- Transmission of Nerve Impulses: The binding of neurotransmitters to the receptors on afferent neurons leads to the depolarization of these neurons. This depolarization generates electrical signals, or nerve impulses, which are then transmitted through the auditory nerve (cranial nerve VIII) to the auditory cortex in the brain.
- Auditory Perception: The auditory cortex in the brain analyzes the incoming nerve impulses and interprets them as sound. The brain not only recognizes the sound but also processes information about the direction, loudness, and pitch of the sound, providing us with a rich auditory experience.
Functions of Ear
The ear is a remarkable sensory organ responsible for our sense of hearing and plays a crucial role in maintaining our balance. It performs several important functions that contribute to our overall auditory perception and spatial orientation. Here are the primary functions of the ear:
- Sound Reception: The outer ear, consisting of the pinna and the external auditory meatus, captures sound waves from the environment. The pinna helps collect and direct the sound waves into the ear canal, where they travel towards the eardrum.
- Sound Amplification: The middle ear, comprised of the tympanic membrane (eardrum) and the three ossicles (malleus, incus, and stapes), amplifies sound waves. The eardrum converts the incoming sound vibrations into mechanical vibrations that are transmitted to the inner ear via the ossicles, which act as a lever system to amplify the sound signals.
- Sound Transmission: The middle ear also serves as a bridge between the outer ear and the inner ear. It transmits the amplified sound vibrations from the eardrum to the fluid-filled cochlea in the inner ear. The stapes, the last of the ossicles, transfers the vibrations to the oval window, a membrane-covered opening in the cochlea.
- Balance and Equilibrium: In addition to hearing, the inner ear plays a crucial role in our sense of balance and spatial orientation. It contains structures called the vestibular system, which includes the semicircular canals and the otolithic organs (utricle and saccule). These structures detect changes in head position and movement, providing information about balance and equilibrium to the brain.
- Sound Transduction: The cochlea, located within the inner ear, is responsible for converting mechanical vibrations into electrical signals that can be interpreted by the brain. Within the cochlea, the basilar membrane vibrates in response to sound waves, causing hair cells to bend and trigger the release of neurotransmitters. These neurotransmitters stimulate the auditory nerve fibers, leading to the generation of nerve impulses that are sent to the brain for processing.
- Auditory Perception: The auditory nerve carries the electrical signals generated by the hair cells to the auditory cortex in the brain. The brain processes and interprets these signals, allowing us to perceive and understand sounds, including their pitch, loudness, and spatial location. This enables us to recognize and discriminate different sounds, such as speech, music, and environmental noises.
- Protection: The ear also has built-in mechanisms to protect itself. The eardrum acts as a barrier, preventing foreign objects from entering the middle ear. The middle ear muscles, such as the tensor tympani and stapedius muscles, contract reflexively in response to loud sounds, dampening their intensity and protecting the delicate structures of the inner ear from potential damage.
Overall, the functions of the ear encompass sound reception, amplification, transmission, balance, sound transduction, auditory perception, and protection. These functions work together to enable us to hear and maintain our sense of balance in our daily lives.
FAQ
What are the main components of the human ear?
The human ear consists of three main parts: the outer ear (including the pinna and ear canal), the middle ear (including the eardrum and ossicles), and the inner ear (including the cochlea and vestibular system).
How does sound travel through the ear?
Sound waves enter the ear through the pinna, travel down the ear canal, and strike the eardrum. The eardrum vibrates in response to the sound waves, which are then transmitted through the ossicles (malleus, incus, and stapes) in the middle ear. The stapes transmits the vibrations to the fluid-filled cochlea in the inner ear.
What is the function of the cochlea?
The cochlea is responsible for converting mechanical vibrations into electrical signals. Within the cochlea, the basilar membrane vibrates in response to sound waves, stimulating hair cells that generate electrical signals sent to the brain for auditory processing.
How does the inner ear help maintain balance?
The inner ear’s vestibular system, including the semicircular canals and otolithic organs, helps detect changes in head position and movement, providing information about balance and equilibrium to the brain.
How does the brain interpret sound signals?
After the cochlea converts sound vibrations into electrical signals, the auditory nerve carries these signals to the auditory cortex in the brain. The brain then processes and interprets the signals, allowing us to perceive and understand sounds.
What role do hair cells play in hearing?
Hair cells are sensory cells located within the cochlea. When stimulated by sound vibrations, the bending of hair cells initiates the release of neurotransmitters, triggering the generation of nerve impulses that are transmitted to the brain.
How do we differentiate between different pitches of sound?
The pitch of a sound is determined by the frequency of sound waves. Different regions of the basilar membrane within the cochlea vibrate in response to different frequencies, allowing us to perceive different pitches.
What causes hearing loss?
Hearing loss can result from various factors, including aging, exposure to loud noises, genetic factors, certain medications, infections, and ear injuries. Damage to the hair cells in the inner ear or other parts of the auditory pathway can lead to hearing loss.
How does the middle ear protect the inner ear from loud sounds?
The middle ear has two small muscles, the tensor tympani and stapedius muscles, that contract reflexively in response to loud sounds. These contractions help reduce the intensity of sound vibrations transmitted to the inner ear, protecting it from potential damage.
Can hearing loss be treated or restored?
Treatment for hearing loss depends on the underlying cause and can include hearing aids, cochlear implants, assistive listening devices, and certain medical interventions. While some types of hearing loss can be improved or managed, the restoration of normal hearing is currently limited.
References
- Sánchez López de Nava A, Lasrado S. Physiology, Ear. [Updated 2022 Aug 22]. In: StatPearls [Internet]. Treasure Island (FL): StatPearls Publishing; 2023 Jan-. Available from: https://www.ncbi.nlm.nih.gov/books/NBK540992/
- Sheikh, A., Bint-e-Zainab, Shabbir, K., & Imtiaz, A. (2022). Structure and Physiology of Human Ear Involved in Hearing. IntechOpen. doi: 10.5772/intechopen.105466
- https://myhealth.ucsd.edu/Search/90%2CP02025
- https://open.oregonstate.education/aandp/chapter/15-3-hearing/
- https://specialist-ent.com/ear-physiology-of-hearing/
- https://www.ops4ent.com/information/anatomy-and-physiology-of-hearing-disorders/
- https://asram.in/asram/study%20materials/files/14-04-2020/Ear%20Mohanty%20Sir.pdf
- https://courses.lumenlearning.com/suny-ap1/chapter/audition-and-somatosensation/
- https://www.urmc.rochester.edu/encyclopedia/content.aspx?ContentTypeID=90&ContentID=P02025
- https://www.onlinebiologynotes.com/physiology-of-hearing/