Gene therapy is a groundbreaking field of medicine that focuses on the modification of cells to treat or prevent genetic disorders by repairing or replacing defective genetic material. It holds the potential to cure a wide range of genetic diseases by directly addressing the underlying genetic abnormalities. In this series of articles, we aim to delve into the intricacies of gene therapy and explore how it works.
At its core, gene therapy involves the introduction of genetic material into the cells of an individual to produce a therapeutic effect. The concept of gene therapy emerged in the 1980s, and since then, significant progress has been made in this field. The first successful attempt at modifying human DNA occurred in 1989, and the first therapeutic use of gene transfer was performed shortly after. Over the years, numerous clinical trials have been conducted to evaluate the safety and efficacy of gene therapy approaches.
Gene therapy has the potential to cure or effectively treat a wide range of genetic disorders. It offers a promising avenue for addressing conditions caused by mutations in genes. These mutations can take various forms, such as single base changes, deletions, duplications, translocations, and copy number variations. The impact of these mutations can range from mild to severe, depending on the specific gene affected and the nature of the mutation. Gene therapy aims to replace or repair the faulty genes responsible for genetic disorders, offering hope for patients and their families.
In this series of articles, we will explore the principles and techniques underlying gene therapy. We will delve into the various strategies employed, such as the use of viral vectors like adeno-associated viruses (AAVs) and lentiviruses for gene delivery. Additionally, we will discuss the challenges and advancements in the field, including the development of non-viral delivery systems and alternative mechanisms for targeted gene therapy.
It is important to note that not all procedures involving genetic modifications can be classified as gene therapy. Certain medical interventions, such as bone marrow or organ transplants, can introduce foreign DNA into a patient’s cells. Gene therapy specifically focuses on the deliberate modification of genetic material to address genetic disorders.
In this introductory article, we have aimed to provide a basic understanding of gene therapy and its potential as a revolutionary approach to treat genetic diseases. In the upcoming articles, we will delve deeper into the mechanisms, applications, and ethical considerations of gene therapy, shedding light on this fascinating and rapidly advancing field of medical science.
Commonly used abbreviations:
Abbreviation | Full name |
GOI | Gene of Interest |
NIH | National Institute of Health |
FDA | Food and Drug Administration |
DMD | Duchenne Muscular Dystrophy |
ITR | Inverted Terminal Repeats |
CF | Cystic Fibrosis |
AAV | Adeno-associated Virus |
HIV | Human Immuno Virus |
SB | Sleeping Beauty Transposon System |
siRNA | Smaller Interfering RNA |
HSV | Herpes Simplex Virus |
CE | Control elements |
Contents
What Is Gene Therapy? – Definition of Gene Therapy
Gene therapy is a revolutionary technique used to treat and potentially cure genetic defects or disorders by either removing the faulty gene or inserting a functional gene into the genome. The ultimate goal of gene therapy is to restore normal gene function and alleviate the symptoms associated with genetic conditions. Importantly, gene therapy should be performed in a manner that ensures the safety and well-being of the patient.
The process of gene therapy involves several key steps. Initially, a specific gene of interest is selected, which can be either a wild type gene or a modified version designed to address the specific genetic defect. This gene is then inserted into a delivery vehicle called a vector. The vector can be a plasmid or any other carrier that can effectively transport the desired gene into the target cells.
Once the gene of interest is incorporated into the vector, the next step is to introduce it into the target cells. This can be achieved through various methods, such as direct injection, viral vectors, or ex vivo manipulation. In ex vivo gene therapy, the transfer of the gene is conducted in a laboratory setting outside the patient’s body, whereas in in vivo gene therapy, the gene is directly inserted into the cells within the patient’s body.
Gene therapy can be used for different purposes depending on the specific objectives of the treatment. These objectives include replacing a fully mutated gene with a functional one, introducing new genes or DNA sequences that are missing, inactivating a mutant gene (gene knockout), or inserting an entirely new gene. These goals vary depending on the genetic disorder being targeted and the desired outcome of the therapy. By using an appropriate vector, the faulty gene can be treated effectively.
There are two main types of gene therapy: in vivo gene therapy and ex vivo gene therapy. In ex vivo gene therapy, the entire process of gene transfer is carried out in a laboratory setting outside the patient’s body. This involves extracting cells from the patient, introducing the desired gene into these cells, and then re-implanting the genetically modified cells back into the patient. In contrast, in in vivo gene therapy, the gene is directly inserted into the cells within the patient’s body without the need for cell extraction and manipulation.
Furthermore, gene therapy can be classified based on the type of cells targeted. Somatic cell gene therapy focuses on treating non-reproductive cells, while germline gene therapy targets reproductive cells. Somatic cell gene therapy aims to alleviate symptoms and provide therapeutic benefits to the individual receiving treatment. Germline gene therapy, on the other hand, involves modifying the genetic material in reproductive cells, which can potentially be passed down to future generations.
Gene therapy holds great promise for the treatment of genetic disorders and has the potential to revolutionize medicine. It offers hope for individuals and families affected by genetic conditions by providing a means to address the root cause of the disease. Ongoing research and advancements in gene therapy techniques continue to expand our understanding of this field, bringing us closer to realizing the full potential of gene therapy in clinical practice.
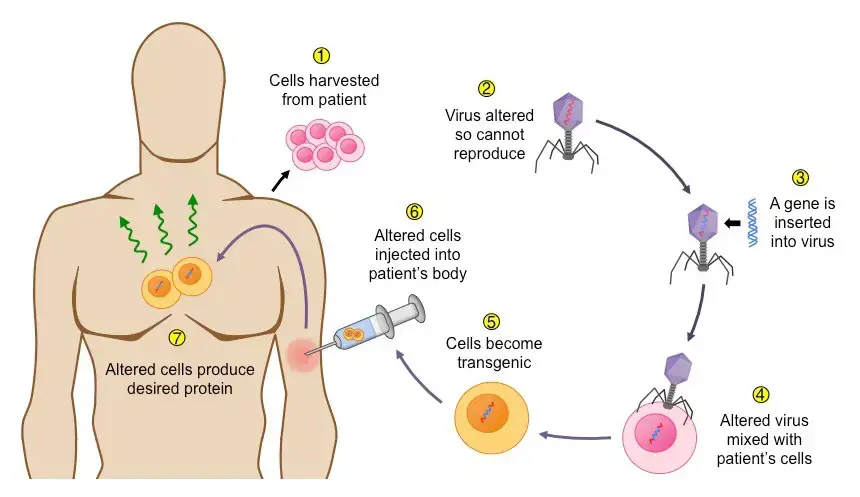
Definition of Gene Therapy
Gene therapy is a technique used to treat genetic disorders by introducing or removing a gene using vectors for gene transfer.
or
Gene therapy is a laboratory genetic technique used to treat genetic disorders by substituting a mutated gene with a healthy one.
History of gene therapy
The history of gene therapy dates back several decades and has undergone significant advancements and milestones. Here is a brief overview of the history of gene therapy:
- Conceptualization (1970s-1980s): The idea of gene therapy first emerged in the 1970s when scientists began to explore the potential of manipulating genes to treat genetic disorders. In 1972, Paul Berg and colleagues demonstrated the ability to insert foreign DNA into the genome of a bacterial virus, paving the way for genetic engineering techniques.
- First attempts (1980s-1990s): The 1980s saw the first attempts at gene therapy in animal models. In 1980, Martin Cline conducted the first human gene therapy trial, aiming to correct a genetic blood disorder. However, the results were not successful. In the late 1980s and early 1990s, the focus shifted to ex vivo gene therapy, where cells are genetically modified outside the body and then reintroduced.
- First successful clinical trial (1990s): The first successful gene therapy clinical trial occurred in 1990, when Ashanti DeSilva, a four-year-old girl with severe combined immunodeficiency (SCID), was treated using ex vivo gene therapy. This breakthrough demonstrated the potential of gene therapy in treating genetic disorders.
- Setbacks and regulatory challenges (1990s-2000s): In the late 1990s and early 2000s, setbacks and safety concerns emerged in gene therapy trials. The death of Jesse Gelsinger in a clinical trial in 1999 raised ethical and safety issues. Regulatory bodies, such as the FDA, tightened the guidelines for gene therapy research to ensure safety and efficacy.
- Advancements in viral vectors (2000s-2010s): During this period, advancements were made in viral vectors used for gene delivery. Adeno-associated viruses (AAV) gained attention as a safer and more efficient vector, overcoming some of the limitations associated with earlier viral vectors. AAV-based gene therapies started showing promise in treating inherited retinal disorders and spinal muscular atrophy.
- FDA approvals and breakthroughs (2010s-present): The 2010s marked significant milestones for gene therapy. In 2012, the FDA approved the first gene therapy product, Glybera, for the treatment of a rare genetic disorder. Since then, several gene therapies have been approved for various conditions, including spinal muscular atrophy, certain types of cancer, and inherited retinal diseases. Advancements in gene editing technologies, such as CRISPR-Cas9, have also expanded the possibilities for gene therapy.
The history of gene therapy reflects a progression from early conceptualization to successful clinical trials and FDA approvals. While challenges and setbacks have occurred along the way, ongoing research and advancements in gene therapy hold promise for the future treatment of genetic disorders.
Types of gene therapy
Gene therapy can be classified into four main types based on the target cells and the method of application:
- Somatic Cell Gene Therapy: Somatic cell gene therapy focuses on treating disorders by targeting somatic cells, which are non-reproductive cells in the body. This type of gene therapy aims to correct or replace the mutated genes within specific tissues or organs. The therapeutic genes are introduced into the targeted somatic cells, and the effects are limited to the individual being treated. Somatic cell gene therapy does not affect future generations as the genetic modifications are not passed on to offspring.
- Germline Gene Therapy: Germline gene therapy involves making genetic modifications to the germ cells, which include sperm or egg cells. The goal of germline gene therapy is to introduce therapeutic genes into the germ cells, which can then be inherited by future generations. This approach aims to prevent the transmission of genetic disorders to offspring. However, germline gene therapy raises ethical concerns and is strictly regulated due to the potential for unintended consequences and the inheritability of the modifications. Currently, germline gene therapy is prohibited in many countries.
- In Vivo Gene Therapy: In vivo gene therapy involves the direct delivery of therapeutic genes into the patient’s body. This is typically done by administering the gene therapy vector, such as a viral vector or nanoparticles, directly into the targeted tissues or organs. The vector carries the therapeutic genes, allowing them to be taken up by the target cells in the body. In vivo gene therapy offers the advantage of directly targeting the affected tissues without the need for cell extraction or manipulation.
- Ex Vivo Gene Therapy: Ex vivo gene therapy involves the removal of cells from the patient’s body, which are then genetically modified outside the body before being reintroduced into the patient. The cells are typically cultured and genetically modified in the laboratory to introduce the therapeutic genes or correct the faulty genes. Once the modifications are made, the cells are returned to the patient’s body, where they can replace the diseased or malfunctioning cells. Ex vivo gene therapy is commonly used when targeting specific cell types or when a high level of control over the genetic modifications is required.
These different types of gene therapy offer diverse approaches to treating genetic disorders and have their own advantages and limitations. The choice of gene therapy type depends on the specific disorder, the target cells involved, and the desired outcomes of the treatment.
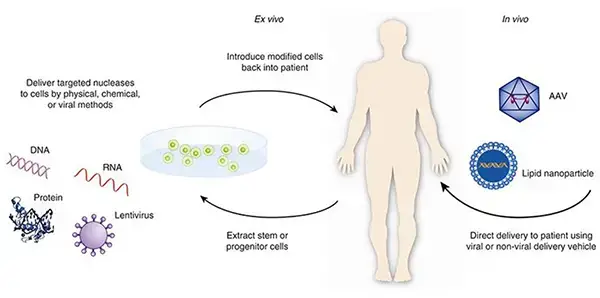
Somatic cell gene therapy
- Somatic cell gene therapy involves replacing or correcting a mutant gene within somatic cells, which are non-reproductive cells in the body. Somatic cells are responsible for the development of various tissues and organs through cell division.
- Unlike germline cells, which are involved in reproduction and can pass on genetic modifications to future generations, somatic cells cannot be inherited. Therefore, the changes made through somatic cell gene therapy are limited to the individual being treated and do not affect future generations.
- The success rate of somatic cell gene therapy is notable, although there are ethical considerations associated with it. The two main methods used in somatic cell gene therapy are virus-mediated gene transfer and liposome-mediated gene transfer.
- While somatic cell gene therapy can be applied to various types of somatic cells, the common target is often bone marrow cells. Bone marrow cells have the ability to divide throughout a person’s lifetime and produce different types of blood cells. Therefore, somatic cell gene therapy has shown greater success in treating blood-related disorders such as thalassemia, sickle cell anemia, and hemophilia.
- In the process of somatic cell gene therapy, a viral vector like adeno-associated virus (AAV) is commonly used to infect isolated bone marrow cells. The vector carries the therapeutic gene, which inserts itself into the target location within the cells’ DNA. The transformed cells are then grown in the laboratory before being reintroduced into the patient’s bone marrow.
- However, somatic cell gene therapy also has its limitations. There is a risk of viral escape, where the virus used as a vector combines with the host genome and infects the cell. Additionally, if the gene does not insert at the specific location, it may disrupt the function of normal genes. The chances of activating oncogenes and proto-oncogenes, which can lead to cancer development, are also a concern.
- It’s important to note that adeno-associated viruses (AAV) used in somatic cell gene therapy can only alter dividing cells, as they directly integrate DNA into the nuclear chromosome.
- Overall, somatic cell gene therapy offers a promising approach for treating genetic disorders, particularly blood-related conditions. Ongoing research and advancements in this field aim to improve the success rate and overcome the limitations associated with somatic cell gene therapy.
Germline gene therapy
- Germline gene therapy involves introducing the gene of interest (GOI) into the germ cells, which include sperm or egg cells, fertilized egg cells, or embryos. Unlike somatic cell gene therapy, germline gene therapy aims to make genetic modifications that can be passed on to future generations.
- The DNA modifications made in the germ cells or embryos can be inherited by the offspring, resulting in a permanent change in the genetic makeup. This raises ethical concerns and has led to the prohibition of germline gene therapy worldwide. Manipulating live embryos is considered unethical by many scientists and society at large.
- The process of germline gene therapy involves infecting the reproductive tissues’ cells or unsplit embryos with viral vectors. These viral vectors deliver the therapeutic genes into the targeted cells. It is crucial to ensure that the modified cells do not become infected with unmodified viral cells during the process. Care must be taken to maintain a sterile laboratory environment.
- The modified germ cells can be used for in vitro fertilization (IVF) and other artificial reproductive techniques. Alternatively, direct injection of the transgene into the embryo is another approach commonly used in germline gene therapy.
- While germline gene therapy holds the potential for preventing the transmission of genetic disorders to future generations, its ethical implications and the potential for unintended consequences have led to its prohibition. The focus of gene therapy research and application has primarily been on somatic cell gene therapy, which targets non-reproductive cells and does not affect future generations.
In vivo gene therapy
- In in vivo gene therapy, the therapeutic gene is directly inserted into the target cells within the body. This approach involves introducing the gene of interest (GOI) into the affected area through aerosols or injections.
- The impact of in vivo gene therapy is limited to specific areas of the body rather than affecting all bodily tissues. This targeted approach is exemplified in conditions such as cystic fibrosis and Duchenne muscular dystrophy (DMD).
- In the case of cystic fibrosis, the exogenous gene or transgene is delivered to the respiratory system using a nasal spray or aerosol. The aim is to introduce the functional gene into the lung cells affected by the disease. Similarly, in DMD, the GOI, which is the dystrophin gene, is injected directly into the muscle cells to provide them with the missing protein.
- However, one challenge of in vivo gene therapy is that some surrounding cells may also be inadvertently infected during the process, leading to potential adverse effects. Achieving precise targeting and minimizing off-target effects remains an ongoing area of research.
- Consequently, in vivo gene therapy is currently not considered a viable option for the treatment of inherited diseases. There are limitations to its effectiveness and safety, and further advancements are necessary before it can become a reliable approach for the treatment of genetic conditions.
- Nevertheless, research continues to explore and refine the techniques and technologies involved in in vivo gene therapy, aiming to improve its specificity, efficiency, and safety for potential future applications.
Ex vivo gene therapy
Ex vivo gene therapy involves collecting cells from the patient’s body, modifying them in a laboratory setting outside of the body, and then reintroducing the modified cells back into the patient’s body. Unlike in vivo gene therapy, where the gene modification is performed directly within the body, ex vivo gene therapy allows for more control over the process and is considered a safer approach.
This technique is applicable to cells that have the capacity to divide. Therefore, it is mainly used for certain types of tissues or cells that possess this ability. One of the commonly targeted cell types is the bone marrow or blood cells, as they have the ability to undergo division throughout a person’s life.
The choice of actively dividing cells is crucial because it allows for faster spread of the introduced transgene to other cells. As a result, ex vivo gene therapy is primarily limited to blood-related disorders. Conditions such as thalassemia, sickle cell anemia, thrombosis, and hemophilia can be treated using this approach.
The steps involved in ex vivo gene therapy are as follows:
- Isolation: The defective or mutant cells are isolated from the patient’s body.
- Gene Insertion: The exogenous gene is inserted into the defective cell lines using viral vectors. Retroviruses and adeno-associated viruses (AAV) are commonly used vectors for ex vivo gene therapy. In the case of retroviruses, the viral sequences are modified to prevent their own DNA from integrating into the host genome.
- Cell Culturing: The modified cells are grown under strict sterile conditions in the laboratory. Care is taken to ensure that the altered cells are not contaminated with other viruses.
- Cell Selection: The transformed cells are carefully selected and grown to obtain a population of cells with the desired genetic modification.
- Cell Reintroduction: The modified cells are then injected back into the patient’s body, where they can replace the defective cells. The success of ex vivo gene therapy depends on the rate of incorporation and expression of the transgene in the patient’s cells.
While ex vivo gene therapy has shown promise for treating certain disorders, it is not suitable for all conditions. For example, it may not be effective for conditions like cystic fibrosis and Duchenne muscular dystrophy. The success rate of ex vivo gene therapy is influenced by various factors, including the efficiency of transgene incorporation and expression in the targeted cells. Ongoing research aims to improve the techniques and technologies involved in ex vivo gene therapy for wider application and enhanced efficacy.
Different techniques of gene therapy
Gene therapy encompasses various techniques aimed at addressing genetic disorders caused by loss of function mutations or abnormal gene expression. In addition to the four types mentioned earlier (somatic cell gene therapy, germline gene therapy, in vivo gene therapy, and ex vivo gene therapy), there are three additional techniques commonly used in gene therapy: gene augmentation therapy, gene inhibition therapy, and suicidal gene therapy.
- Gene Augmentation Therapy: Gene augmentation therapy involves introducing functional copies of a gene into the patient’s cells to supplement or replace the defective or non-functioning gene. This approach aims to increase the expression of the desired gene to restore normal function. The introduced gene may be delivered using viral vectors or other delivery systems, such as liposomes or nanoparticles. This technique is particularly relevant for genetic disorders caused by a loss of function mutation, where the gene is either absent or not functioning properly.
- Gene Inhibition Therapy: Gene inhibition therapy aims to suppress or downregulate the expression of specific genes that contribute to the development or progression of a genetic disorder. This technique involves using various approaches to interfere with the expression or activity of the targeted gene. Examples of gene inhibition strategies include the use of small interfering RNAs (siRNAs) or antisense oligonucleotides (ASOs) to specifically bind to the target gene’s messenger RNA (mRNA) and inhibit its translation or degrade the mRNA itself. By reducing the expression of disease-causing genes, gene inhibition therapy aims to mitigate the effects of the genetic disorder.
- Suicidal Gene Therapy: Suicidal gene therapy, also known as gene-directed enzyme prodrug therapy (GDEPT), involves introducing a gene into the patient’s cells that codes for an enzyme capable of activating a prodrug (an inactive form of a therapeutic agent). The introduced enzyme converts the prodrug into its active form, which selectively kills the target cells. This technique is particularly useful for targeted cancer therapy, where the activated drug can specifically destroy cancer cells while sparing healthy cells. Suicidal gene therapy exploits the differential expression of certain genes between normal and diseased cells to achieve targeted therapeutic effects.
These different techniques of gene therapy provide a range of approaches to address genetic disorders at the molecular level. Depending on the specific nature of the genetic disorder and the therapeutic goals, the most appropriate technique or combination of techniques can be selected to modulate gene expression, restore function, or selectively eliminate diseased cells. Continued research and advancements in gene therapy techniques hold promise for the development of effective treatments for a wide range of genetic disorders in the future.
Gene augmentation therapy
- Gene augmentation therapy is a technique used in gene therapy to address genetic disorders caused by loss of function mutations. Loss of function mutations refers to mutations in a gene that result in the loss or reduction of its natural function, leading to the inability of the affected cells to produce a functional protein.
- In gene augmentation therapy, the defective or non-functioning gene is replaced with a fully functional wild-type gene. This wild-type gene encodes the normal, functional protein that is required for proper cellular function. By introducing the wild-type gene into the patient’s cells, gene augmentation therapy aims to restore the production of the functional protein and alleviate the symptoms associated with the genetic disorder.
- This technique is particularly effective for monogenic disorders, which are caused by mutations in a single gene. Cystic fibrosis is an example of a genetic disorder that can be treated using gene augmentation therapy. In cystic fibrosis, a mutation in the CFTR gene leads to the production of a faulty protein that affects the function of ion channels in various organs, particularly the lungs and digestive system. Gene augmentation therapy in this case involves introducing a functional CFTR gene into the patient’s cells to restore the production of the normal CFTR protein and improve the function of the affected organs.
- The delivery of the wild-type gene can be achieved using various methods, including viral vectors such as adenoviruses, adeno-associated viruses (AAVs), or lentiviruses. These vectors serve as vehicles to deliver the desired gene into the target cells and facilitate its integration into the cellular genome. The choice of vector depends on factors such as efficiency, safety, and the specific requirements of the targeted disorder.
- Gene augmentation therapy holds promise for the treatment of a wide range of monogenic disorders where loss of function mutations result in the loss of normal protein function. By replacing the defective gene with a functional copy, this approach aims to restore the cellular and physiological processes affected by the genetic disorder. Continued research and advancements in gene augmentation therapy offer hope for improved treatments and outcomes for individuals with monogenic disorders in the future.
Gene inhibition therapy
- Gene inhibition therapy, also known as gene silencing therapy, is a technique used in gene therapy to address diseases that are caused by the overexpression of certain genes, such as cancer.
- The overexpression of a gene can lead to the development of life-threatening conditions, including various types of cancer. This overexpression can be a result of changes in the methylation pattern or epigenetic profile of the gene, which affects its regulation and leads to abnormal levels of gene expression.
- In gene inhibition therapy, the goal is to inhibit or reduce the expression of the overactive gene. This can be achieved through various approaches. One approach involves using another gene or DNA sequence to interfere with the expression of the target gene. This can be done by introducing a gene or DNA sequence that produces RNA molecules that can bind to the target gene’s mRNA, preventing its translation into protein or leading to its degradation. This interference effectively reduces the levels of the target gene’s product and restores a more normal gene expression pattern.
- Another approach in gene inhibition therapy involves directly interfering with the activity of the product of the overexpressed gene. This can be achieved through the use of small molecules, such as small interfering RNA (siRNA) or antisense oligonucleotides (ASOs), that specifically target the mRNA of the overexpressed gene and inhibit its translation or promote its degradation.
- Gene inhibition therapy has shown promise in the treatment of various diseases, including inherited disorders, infectious diseases, and cancer. In the context of cancer, this approach can be particularly valuable for reducing the expression of oncogenes, which are genes that promote the development and progression of cancer. By inhibiting the overexpression of oncogenes, gene inhibition therapy aims to slow down or halt the growth of cancer cells and improve patient outcomes.
- The development of effective gene inhibition therapies relies on understanding the underlying molecular mechanisms of gene regulation and identifying specific targets for intervention. Advances in gene editing technologies, such as CRISPR-Cas9, have provided powerful tools for precisely modulating gene expression and have expanded the possibilities for gene inhibition therapy.
- Further research and ongoing clinical trials are aimed at refining and optimizing gene inhibition therapies, paving the way for improved treatments for a wide range of diseases in the future.
Suicidal gene therapy
- Suicidal gene therapy is a specialized gene therapy technique used to target and eliminate specific cells, especially in the context of cancer treatment.
- Certain diseases, such as cancer, necessitate the elimination of specific cells that contribute to the progression of the disease. Suicidal gene therapy entails the introduction of suicide genes into targeted cells. These genes are designed to produce a toxin or protein capable of causing cell demise.
- These suicide genes are engineered to be expressed selectively in the target cells. Once the suicide genes are expressed, the cells produce a toxic substance or protein. This toxin induces a robust immune response against the cells containing the suicidal gene, resulting in their annihilation. The immune response may involve the activation of T cells or natural killer cells, which recognize and attack the targeted cells.
- Suicidal gene therapy is particularly effective at treating cancer. This therapy can specifically target and destroy cancer cells by introducing suicide genes into them, potentially reducing tumor size and slowing disease progression. The immune response generated against cells containing suicide genes can contribute to the elimination of cancer cells and improve the efficacy of the treatment.
- Depending on the specific gene or protein employed and the type of cancer being treated, different suicidal gene therapy strategies may be employed. Utilizing viral vectors to deliver the suicide genes to the target cells is a prevalent method. The viral vectors can efficiently transport genetic material into cancer cells, enabling the expression of suicide genes and subsequent induction of cell death.
- Suicidal gene therapy has the potential to be an effective cancer treatment method. Nonetheless, it is essential to design and optimize these therapies with care to ensure their efficacy and mitigate potential side effects. The ultimate objective of ongoing research and clinical trials is to improve cancer treatment outcomes through the development of safer and more effective suicide gene therapy approaches, as well as the identification of optimal target genes and delivery mechanisms.
Gene therapy vectors (Viral and non-viral)
Vectors for gene therapy are indispensable for delivering therapeutic genes, also known as transgenes, into the host genome. These vectors can be divided into two categories: viral and non-viral vectors. Each type has advantages and disadvantages, and the selection of the vector is based on a variety of criteria.
Viral vectors are derived from naturally occurring viruses modified to transport therapeutic genes. In gene therapy research and clinical trials, these vectors have been extensively investigated and employed. They provide numerous benefits, such as high transduction efficiency, the capacity to transport large transgenes, and long-term expression of the transgene. However, viral vectors have the potential to induce an immune response in the host, which is a potential disadvantage. This immune response can reduce the efficacy of the treatment and cause adverse effects. Retroviruses, lentiviruses, adenoviruses, and adeno-associated viruses (AAVs) are common viral vectors.
In contrast, non-viral vectors do not rely on viral components for gene delivery. Instead, they employ non-viral vectors such as liposomes, nanoparticles, and bare DNA. Compared to viral vectors, non-viral vectors offer advantages such as reduced immune response, simplicity of production, and greater safety. However, they typically have a lower transduction efficiency and a reduced duration of transgene expression. Non-viral vectors are especially valuable for delivering smaller transgenes and can be administered repeatedly.
The choice of vector for gene therapy is contingent on a number of variables. Initially, the magnitude of the transgene is crucial. For the delivery of larger transgenes, viral vectors are typically preferable, whereas non-viral vectors are suitable for smaller genes. Second, delivery efficiency is crucial for the successful transduction of target cells. Viral vectors are renowned for their high transduction efficiency, whereas non-viral vectors may need further optimization to enhance their delivery efficiency.
The vector’s potential to elicit an immune response in the host is an additional crucial factor to consider. As a result of their viral origin, viral vectors are more likely to provoke immune responses. In contrast, non-viral vectors are typically less immunogenic. This quality makes them advantageous for certain applications, particularly when repeated administrations of the therapy are necessary.
The stability and duration of transgene expression are crucial factors to consider. Due to their capacity to integrate the transgene into the host genome, viral vectors frequently provide long-term expression. However, non-viral vectors typically result in transient transgene expression.
Lastly, the quantity of transgene expression is an important factor in ensuring therapeutic efficacy. Both viral and non-viral vectors are capable of achieving high expression rates, although viral vectors are typically more effective in this regard.
Conclusion: vectors for gene therapy serve a crucial role in the delivery of therapeutic genes to target cells. Viral vectors provide high transduction efficiency, the ability to transport larger transgenes, and long-lasting expression. In contrast, non-viral vectors have benefits such as reduced immune response and simplicity of production. The choice of vector is determined by the specific requirements of the therapy, such as the transgene’s size, delivery efficiency, immune response, stability, and expression level. There is hope for the treatment of a variety of genetic disorders and diseases as vector technology continues to enhance the safety and effectiveness of gene therapy methods.
Viral vectors
In gene therapy, viral vectors are widely employed as efficient vectors for delivering therapeutic genes to target cells. Viruses of various varieties have been engineered and altered for this purpose. Here, we will discuss some of the most frequently used viral vectors in gene therapy:
- Retrovirus: Retroviruses are RNA viruses that have been examined extensively and utilized in gene therapy. These viruses are capable of integrating their genetic material, including the therapeutic gene, into the genome of the host cell. This integration permits long-term transgene expression. However, retroviruses have a limited capacity for packaging and can only accommodate transgenes that are relatively small. In clinical trials, they have been used successfully to treat diseases such as severe combined immunodeficiency (SCID) and certain forms of malignancy.
- Lentivirus: Due to their ability to infect both dividing and non-dividing cells, lentiviruses have acquired popularity as gene therapy vectors. This quality makes them suitable for targeting a variety of cell types within the body. Lentiviral vectors, such as those derived from the human immunodeficiency virus (HIV), have been modified to eliminate disease-causing viral genes while retaining gene delivery capabilities. Compared to retroviruses, lentiviral vectors can transport larger transgenes and are extensively used in preclinical and clinical studies for the treatment of various genetic disorders and neurodegenerative diseases.
- Adenovirus: Due to their high transduction efficiency, adenoviruses are frequently employed as gene therapy vectors. They can infect a wide variety of dividing and non-dividing cells and have a high packaging capacity, enabling them to transport larger transgenes. Adenoviral vectors do not integrate into the host genome; rather, they provide temporary expression of the therapeutic gene. This characteristic makes them suitable for applications in which long-term expression is unnecessary. In clinical trials, adenoviral vectors have been utilized for cancer gene therapy and vaccine delivery.
- Adeno-Associated Virus (AAV): AAVs are small, non-pathogenic viruses that have received considerable attention in gene therapy. AAV vectors are derived from AAV in its natural state, and their genome does not integrate with the host genome. However, they can sustain episomal expression of the transgene over time. AAV vectors are capable of infecting both dividing and non-dividing cells and have a diverse host range. They are recognized for their high safety profile and low immunogenicity. Several successful clinical trials have utilized AAV vectors to treat inherited retinal disorders, hemophilia, and muscular dystrophy.
These viral vectors offer distinct benefits and limitations, making them appropriate for various gene therapy applications. The choice of viral vector is determined by variables such as the target cell type, the quantity of the transgene, the requirement for long-term or transient expression, and safety considerations. Continued research and technological advances in viral vectors seek to improve their efficacy, safety, and targeting capabilities, thereby expanding the therapeutic potential of gene therapy for a variety of genetic diseases and disorders.
1. Retrovirus
Retroviruses are RNA viruses commonly used in gene therapy due to their ability to integrate their genetic material into the host cell’s genome. They are also referred to as retrovirus-mediated gene therapy. Retroviruses have a unique mechanism called reverse transcription, where their RNA genome is converted into DNA, specifically complementary DNA (cDNA), which is then integrated into the host genome.
Structurally, retroviruses contain several essential components. The gag gene encodes for the viral core protein, the pol gene encodes for the reverse transcriptase enzyme responsible for reverse transcription, and the env gene encodes for the envelope protein present on the viral surface, aiding in the recognition of specific receptors on the target cells. The retrovirus genome also includes long terminal repeats (LTRs) on both ends, which are necessary for the integration of the reverse-transcribed DNA into the host genome. Additionally, the Ψ sequences are crucial for viral packaging.
However, when utilizing retroviruses as gene therapy vectors, certain precautions must be taken. The Ψ sequences, responsible for viral packaging, need to be removed to prevent the creation of new viral particles inside the host cell. Moreover, integration of the retroviral DNA upstream of oncogenes in the host genome can activate these oncogenes, potentially leading to cancer. Therefore, it is crucial to ensure that the altered cell lines used for gene therapy experiments are not contaminated with replication-competent viruses, which can replicate in the host cell.
In gene therapy experiments using retroviruses, three types of plasmids are commonly employed. Plasmid 1 contains the 5′ LTR and an env gene but lacks Ψ sequences, rendering it incapable of producing virions. Plasmid 2 includes the 5′ LTR, gag, and pol genes without Ψ sequences, providing essential components for replication. Plasmid 3, sandwiched between both 3′ and 5′ LTRs, contains a gene of interest (GOI) with a promoter and the Ψ sequence, allowing the formation of mature virion particles carrying the GOI.
The pol gene in retroviral vectors encodes the reverse transcriptase enzyme, which facilitates the integration of the target DNA into the host genome. Notably, plasmids lacking virion-forming sequences only possess the 3′ LTR, while plasmid 3, which produces virions carrying the GOI, contains both 3′ and 5′ LTRs. The 5′ LTR includes the promoter necessary for retroviral gene transcription. By using only the 3′ LTR sequences in non-virion plasmids, the risk of viral activity is minimized.
To ensure safety, each altered cell line is carefully screened for the presence of replication-competent viruses before being administered to the patient. This method provides advanced safety measures.
Another approach involves using a helper virus alongside the retrovirus. The env gene is removed from the retrovirus and used to infect the target cell. The helper virus acts as a vector to insert the GOI. However, this method carries a higher risk of infection as the cell line can be contaminated with both retroviruses and the helper virus.
Despite its promise, retrovirus-mediated gene therapy has limitations. Retroviruses can only infect dividing cells because they insert DNA directly into the chromosomes, making them unsuitable for diseases like Duchenne muscular dystrophy (DMD) and cystic fibrosis (CF) that affect non-dividing cells. The size of DNA that retroviruses can transfer is limited to around 8 kilobases (kb). Retroviruses also lack precise target specificity, potentially leading to the transfer of the GOI to unintended cell types. Integration of the retroviral DNA occurs randomly, which can result in integration near cellular oncogenes, leading to the activation of these oncogenes and potential cancer formation. Additionally, there is a risk of the retrovirus itself infecting the host cell if contamination occurs.
To improve target specificity, modifications can be made to the viral envelope protein. Murine leukemia virus (MLV) is a commonly used retrovirus in gene therapy research.
Addressing these limitations and ongoing research in retroviral vector technology aim to enhance their safety, efficiency, and target specificity, making them valuable tools for gene therapy in the future.
2. Adenovirus
Adenovirus is another commonly used viral vector in gene therapy due to several advantageous properties. Unlike retroviruses, adenoviruses have a double-stranded DNA genome with a size of approximately 35 kilobases (kb). The viral genome is enclosed within an icosahedral capsid composed of 12 different proteins.
One of the key advantages of adenovirus as a gene therapy vector is its ability to naturally infect non-dividing cells, particularly cells in the respiratory and gastrointestinal tracts. This characteristic makes it well-suited for diseases such as cystic fibrosis, where the target cells are non-dividing. Additionally, adenoviruses have the capability to evade the host immune response, which is a significant advantage in gene therapy applications.
Adenovirus vectors exhibit high target specificity, meaning they primarily infect and integrate their DNA into their host cells rather than surrounding cells. Among the 50 different serotypes of adenovirus, serotypes 3 and 5 demonstrate a higher tropism for respiratory tract cells.
However, it is crucial to disable the viral replicating mechanism to mitigate the harmful effects of adenovirus. The viral gene expression in adenovirus can be divided into two phases: early and late phases. The virus possesses 100-base-pair terminal inverted repeats that flank the 35 kb DNA within its structure.
Clinical trials involving adenovirus-based gene therapy have revealed that even in an inactive state, adenovirus can stimulate a strong immune response. This immune response needs to be carefully managed to avoid adverse reactions in patients undergoing gene therapy using adenoviral vectors.
Continued research and advancements in adenovirus vector design aim to improve their safety, target specificity, and immune response regulation. These efforts contribute to the ongoing development of adenovirus-based gene therapies for various genetic diseases and disorders.
3. Adeno-associated virus
- Adeno-associated virus (AAV) has a genome composed of single-stranded DNA and contains two genes: “rep” and “cap.”
- Terminal repeats are present on both ends of the AAV genome.
- The rep gene encodes a protein that facilitates the integration of AAV into the host genome, particularly on chromosome 9.
- The cap gene encodes a protein responsible for constructing the AAV capsid.
- AAV is naturally non-replicative and requires a helper virus to perform its functions. Adenovirus is commonly used as a helper virus along with AAV.
- AAV is a suitable alternative to adenovirus as it can infect both dividing and non-dividing cells. It primarily targets cells in the upper respiratory airways and exhibits long-lasting expression for over 6 months.
- In gene therapy applications, the rep and cap genes of AAV are replaced with the transgene of interest. Removing the rep gene eliminates AAV’s specific integration capability on chromosome 9.
- Due to its ability to provide long-lasting expression, AAV has been approved for human trials in gene therapy for conditions such as hemophilia A.
4. Lentivirus
- Lentivirus is a type of retrovirus that can infect both dividing and non-dividing cells. HIV is a well-known example of a lentivirus.
- The genetic composition of a lentivirus is RNA, which carries env, gag, and pol genes. Lentivirus specifically infects T cells.
- However, using HIV for gene therapy is not considered a favorable choice due to its pathogenic nature.
- Lentivirus has a DNA carrying capacity of less than 8kb.
- Positives of lentiviral vectors include the ability to infect proliferating, non-proliferating, and bone marrow cells.
- Lentiviral vectors are self-inactivating, which means they have a reduced risk of causing harm.
- The chance of infection with lentivirus is relatively high.
- Lentiviral vectors can provoke an immune response.
In summary:
- Adenovirus has a DNA carrying capacity of 8kb to 35kb and can infect both dividing and non-dividing cells. It has a lower chance of causing infection but can provoke a strong immune response. Expression is transient.
- AAV has a DNA carrying capacity of up to 4.5kb and provides longer transgene expression. It is non-pathogenic and has broad tropism. However, it requires a helper virus and has a smaller DNA carrying capacity.
- Retrovirus has a DNA carrying capacity of less than 8kb. It allows stable integration, but it cannot infect non-dividing cells and has adverse effects. It can also provoke an immune response.
- Lentivirus has a DNA carrying capacity of 8kb. It can infect proliferating, non-proliferating, and bone marrow cells. It is self-inactive, but the chance of infection and immune response is relatively high.
- HSV has a DNA carrying capacity of 30kb. It is considered safer, has a higher DNA carrying capacity, and is suitable for in vivo gene therapy. However, it is difficult to produce.
Non-viral vector
Non-viral vectors are gaining significant attention in the field of gene therapy due to their promising characteristics and potential advantages over viral vectors. Liposomes, naked DNA, nucleofection, and transposons are among the non-viral vector-mediated methods that have shown promise in gene transfer.
One of the key advantages of non-viral vectors is their non-toxic nature. Unlike viral vectors, which can trigger immune responses and have the potential to cause adverse reactions, non-viral vectors are generally considered safe and do not elicit significant immune reactions. This non-toxicity is a crucial aspect when it comes to therapeutic applications, as it minimizes the risk of harmful side effects.
Non-viral vectors are also non-immunogenic, meaning they do not provoke strong immune responses in the body. This is particularly important in the context of repeated administration of gene therapy, as the immune system’s response to viral vectors can reduce their efficacy over time. By using non-viral vectors, the risk of immune recognition and subsequent neutralization of the therapeutic payload is minimized, allowing for more effective and sustained gene transfer.
Another advantage of non-viral vectors is their tissue-specificity. These vectors can be designed to target specific tissues or cell types, allowing for precise and targeted delivery of therapeutic genes. By incorporating tissue-specific targeting ligands or modifying the vector’s surface properties, non-viral vectors can be engineered to selectively interact with specific cells or tissues of interest. This targeted approach enhances the efficiency and specificity of gene transfer, reducing off-target effects and improving overall therapeutic outcomes.
Moreover, non-viral vectors offer flexibility in design and application. Unlike viral vectors, which are often limited in terms of cargo capacity and integration sites, non-viral vectors can accommodate larger DNA fragments and can be tailored to meet specific therapeutic requirements. They can be easily modified and optimized to enhance stability, cellular uptake, and gene expression, making them versatile tools for gene delivery.
While non-viral vectors hold great promise, it’s important to note that they also have certain limitations. Compared to viral vectors, non-viral vectors generally exhibit lower transfection efficiency, meaning they are less effective at delivering genes into target cells. However, ongoing research and advancements in vector design and formulation techniques are addressing this limitation and improving the efficacy of non-viral vectors.
In conclusion, non-viral vectors offer several advantages that make them attractive options for gene transfer in gene therapy. Their non-toxic, non-immunogenic, and tissue-specific nature, coupled with their design flexibility and ease of application, contribute to their potential as effective tools in the field of gene therapy. Continued research and development in this area are expected to further enhance the capabilities of non-viral vectors and unlock their full potential for safe and efficient gene delivery.
Liposome
- Liposomes have emerged as a promising non-viral vector for gene therapy, conveying genetic material to target cells in a safe and efficient manner. Liposomes are comprised of lipids and are artificially synthesised molecules ranging in size from 0.025 to 2 μm.
- Due to the negative charge present on both the cell surface and the DNA, one of the major obstacles in gene therapy is the inability of DNA to directly penetrate the cell membrane. This electrostatic repulsion hinders the delivery of DNA efficiently. Liposomes are used to surmount this obstacle.
- Due to their hydrophilic (water-loving) and hydrophobic (water-repelling) properties, lipid molecules can form a protective lipid bilayer. The hydrophilic head of the lipid is exposed to the aqueous environment, whereas the hydrophobic tail resides within the lipid bilayer. This lipid bilayer encapsulates the DNA solution, thereby facilitating its penetration into the cell.
- Due to their diminutive size, however, liposomes have limitations when it comes to the transfer of larger genes. In addition, they may not possess a strong affinity for DNA and the cell surface. In response, a modified form of liposomes dubbed “lipoplex” has been created.
- Lipoplex is created by imbuing the hydrophilic domain of the lipid with a positive charge. This alteration permits lipoplex to attract both DNA and the cell surface, resulting in a more stable lipid-DNA complex. Lipoplex’s tube-like structure efficiently facilitates DNA transfer into the target cell. In addition, lipoplex is non-immunogenic, making it preferable to liposomes and virus-based vectors because it reduces the risk of immune responses.
- Additionally, lipoplex is simple to prepare and has the capacity to transfer a substantial quantity of DNA. It is essential to observe, however, that lipoplex is not as effective as virus-based gene therapy, which remains the gold standard for gene transfer efficiency.
- In conclusion, liposomes and their modified form, lipoplex, provide an effective method for non-viral gene therapy. They offer a secure and effective method for delivering DNA to target cells. While lipoplex overcomes some of the limitations of liposomes, such as enhanced affinity for DNA and the cell surface, it cannot match the efficiency of virus-based gene therapy. Nevertheless, lipoplex retains promise and continues to be a focus of gene therapy research and development.
Transposon
- Following the development of CRISPR-Cas9 technology, transposon-mediated gene therapy is an emergent therapeutic approach that holds promise in the field of genetic engineering.
- Transposons are mobile genetic elements that can migrate from one location within the genome to another. Similar to viruses, they consist of coding DNA and terminal repeats. However, the majority of transposons are inactive and do not exhibit long-term genomic mobility.
- From a fish fossil, scientists discovered an active transposon known as the Sleeping Beauty transposon. The Sleeping Beauty (SB) system is able to insert DNA into the genome of the host without causing significant adverse effects. It overcomes the limitations of inactive transposons by transferring genes efficiently and effectively.
- In gene therapy, the SB transposon system has several advantages. First, it has the ability to efficiently deliver a gene of interest to its target site within the genome. This targeted delivery improves the accuracy and specificity of gene transfer, reducing off-target effects. In addition, the SB transposon system is designed to evade the host immune system, thereby diminishing the likelihood of immune reactions and extending the duration of gene expression.
- Prior to advancing to pre-clinical trials, however, transposon-mediated gene therapy must overcome a number of obstacles and limitations. These constraints include the optimisation of transposon efficacy, the minimization of potential genotoxicity, and the guaranteeing of the long-term stability of transferred genes. Ongoing research and development focuses on enhancing the safety and effectiveness of transposon-based gene therapy.
- Despite these obstacles, the SB transposon system and the revolutionary CRISPR-Cas9 technology hold tremendous promise for future gene therapy advancements. As scientists continue to refine and optimise these systems, it is anticipated that they will become more efficient and trustworthy instruments in the field of genetic engineering.
- It is important to note that the previous article referenced in the text contains detailed information on the Sleeping Beauty transposon, including its discovery and mechanism of action. This article provides comprehensive knowledge of the SB transposon system.
- The direct injection of DNA into cells without a carrier or delivery vehicle is referred to as naked DNA or plasmid DNA. Experiments on rodents have demonstrated that naked DNA can be injected directly into muscle cells via lesions on the cell surface.
- Given that both DNA and the cell surface contain negatively charged molecules, which would normally repel one another, the notion that bare DNA can penetrate cells is somewhat counterintuitive. Despite this theoretical barrier, scientists have observed that cells can absorb bare DNA and express the desired transgene.
- Potential exists for the production of therapeutic proteins from naked DNA. It is possible to stimulate the production of specific proteins, including insulin and thrombotic factors, by inserting a transgene into muscle cells using exposed DNA. This strategy has implications for the treatment of diseases that require the body’s production of specific proteins.
- It is important to emphasise, however, that the evidence currently supporting naked DNA-mediated gene therapy is limited. Although promising results have been observed in animal models, additional research is required to determine the safety, efficacy, and long-term effects of this approach in humans. Extensive research data and clinical trials are required to comprehend and validate the viability of bare DNA as a gene therapy method.
- In conclusion, bare DNA is a novel and direct method for gene therapy, eliminating the need for carriers or delivery vehicles. Despite indications that it can penetrate cells and produce therapeutic proteins, additional research is necessary to determine its maximum potential and evaluate its safety and efficacy for human applications. Continued research and clinical trials will shed additional light on the viability and efficacy of bare DNA-mediated gene therapy.
Non-viral vectors mediated gene therapy
Some of the other non-viral vectors mediated gene therapy methods are discussed here.
The non-viral vectors are further divided into three broader categories.
- Physical methods
- Chemical methods
- Synthetic molecules
a. Physical methods
In gene transfer, various physical forces or procedures, such as needles, gene cannons, electroporation, ultra-sonication, and laser photoporation, can be utilised.
- Electroporation
- In vitro and in vivo electroporation
- Application of high electrical current to create pores on the cell surface
- Duration and amplitude of pulses determine membrane permeability
- Delivery of transgene intramuscularly or intradermally
- Higher specificity and success rate for in vivo electroporation
- Limited accessibility to internal organs
- Also known as electro-gene injection or electrical mediated gene therapy
- Sonoporation
- Creation of temporary cell membrane permeability using ultrasonic waves
- Sonoporation-mediated cavitation disrupts cell membrane and creates holes
- Use of artificial microbubbles enhances efficiency
- Microbubbles can be loaded with transgenes
- Site-specific, non-invasive, and applicable to internal organs
- Suitable for various tissue types with increased specificity using ligands or antibodies
- Gene Gun
- Introduction in 1987
- Utilizes highly pressurized gas and metal ions
- Microparticles deliver transgenes at high speed
- Efficiency depends on gas pressure, particle size, and transgene dose
- Commonly used for intradermal, intramuscular, or intratumor gene transfer
- High success rate with 1μm metal particles
- Photoporation
- Laser-induced pores in the cell membrane for transgene introduction
- Success depends on laser frequency and focal length
- Less frequently used due to lack of documented evidence
- Comparable effectiveness to electroporation
- Hydroporation
- Direct injection of DNA solution via hydrodynamic pressure
- Commonly used for gene transfer in hepatic cells
- Needle
- Direct injection of gene of interest through a needle
- Suitable for gene insertion in skin, muscle, liver, and cardiac cells
- Simple method but lower efficiency compared to other vectors
- Magnetofection
- Magnetically charged particles coupled with the gene of interest
- Transgene insertion under a higher magnetic field
- More suitable for ex vivo applications
b. Chemical procedure:
We do not discuss all the compounds used in gene therapy in this article. Gene delivery chemical agents include gold particles, silver particles, silica, and calcium phosphate.
By complexing with the gene or shielding it from nucleases and other enzymes, these particles efficiently convey the gene into the cytosol.
c. Synthetic nanoparticles:
Synthetic nanoparticles are an alternative, safer, and more user-friendly source for gene delivery.
Cyclodextrins, like cyclic natural nanomaterials, are capable of interacting with DNA with low immunogenicity. Therefore, it is one of the finest nanomaterials naturally available for gene therapy.
A foreign particle must evade endocytosis, the process that kills it, in order to survive.
Polyethyleneimine (PEI) is a gene delivery vector that aids in the escape of the gene of interest (GOI) from the endosome. However, because of the vector’s high positive charges, it is less effective.
Polyethyleneglycol (PEG) is one of the most effective gene therapy nanoparticles. It can efficiently deliver DNA to its intended destination.
It is primarily used for siRNA delivery.
In addition to all of these, some peptides and proteins are used for gene transfer.
As gene delivery vehicles, other nanoparticles such as dendrimers, polymethacrylate, chitosan, and cationic synthetic lipids are also utilised.
How does gene therapy work?
Gene therapy is a complex process that aims to treat genetic disorders by introducing a healthy gene into target cells. Here is a step-by-step explanation of how gene therapy works:
- Gene of interest: The gene therapy process begins with identifying the specific gene that needs to be replaced or corrected. This gene of interest (GOI) is the healthy gene that will be introduced into the patient’s cells.
- Vector selection: A vehicle or vector is needed to carry the GOI into the target cells. Various vectors can be used, including viruses (such as retroviruses, adenoviruses, and adeno-associated viruses), transposons, or even naked DNA. Viruses are commonly used as vectors, but they are modified to ensure that they cannot cause any infection in the target cells.
- GOI introduction into the vector: The GOI is inserted into the chosen vector, which will serve as a delivery system for the gene.
- Vector introduction into the target cell: The vector carrying the GOI needs to be introduced into the target cells. This can be achieved through techniques like electroporation, gene gun, particle bombardment, or intravenous injection.
- Integration into the target cell: Once the vector reaches the target cells, it inserts the GOI at a specific location within the cell’s genome. The vector itself is typically destroyed by the host cell.
- Replication and expression: The introduced GOI begins to replicate within the host cell. As the host cell undergoes multiple cycles of division, the mutant cells are gradually replaced by cells that contain the new, healthy gene. The GOI is transcribed into messenger RNA (mRNA), which is then translated into the production of new, functional proteins.
It is important to note that the success and applicability of gene therapy vary depending on the type of genetic disorder and the target cells involved. For somatic tissues (non-reproductive cells), such as specific parts of the brain affected by Parkinson’s disease, gene therapy has shown promise. However, germline gene therapy, which involves altering genes in reproductive cells or embryos, is strictly prohibited due to ethical and safety concerns.
While gene therapy holds great potential, there are still limitations and risks associated with this approach. The success rate of gene therapy remains low, and the potential harmful effects or long-term consequences are not fully understood. Adverse immune responses, off-target effects, potential impact on healthy cells, and the risk of oncogenic activity are some of the challenges that need to be addressed.
Newer techniques, such as the use of transposons (like the sleeping beauty transposon system) and CRISPR-Cas9, offer more targeted and potentially safer approaches to gene therapy. These methods are being extensively studied and tested to determine their efficacy and safety in clinical settings.
Additionally, non-viral vectors like liposomes, naked DNA, nanoparticles, and nucleofection have emerged as alternatives to viral vectors, reducing the risk of immune responses and enabling more controlled gene transfer.
While monogenic disorders caused by single-gene defects have shown promising results with gene therapy, treating polygenic disorders (caused by multiple genes) remains challenging due to the complexity of targeting and modifying multiple genes simultaneously.
In summary, gene therapy is a multi-step process that involves introducing a healthy gene (GOI) into target cells using a vector. While there are limitations and risks associated with gene therapy, ongoing advancements in techniques and vector systems offer potential solutions and improvements for the future.
The process of gene therapy
Gene therapy is a promising approach in the field of medicine that aims to treat or prevent diseases by modifying a person’s genes. The process of gene therapy involves several important steps, which are crucial for the success of the experiment. These steps can be categorized into five main stages: selection of the gene of interest (GOI), selection of a plasmid, selection of a vector, selection of a method, and selection of a technique.
- Selection of GOI: The first step in gene therapy is to identify and select the appropriate gene of interest. This selection is based on the specific disease being targeted. It is generally more effective to transfer a single gene rather than multiple genes, so selecting a monogenic disease yields better results. The size of the gene also plays a role in the success of the experiment, as smaller DNA fragments have higher transfer efficiency. The selected GOI should have specific characteristics, such as high AT-rich sequences, a GC content of less than 50%, and a minimal number of exons.
- Selection of plasmid: A plasmid is a small, circular DNA molecule that plays a crucial role in delivering the gene of interest into the host genome. The plasmid selected for the experiment should have a high transgene carrying capacity, meaning it can accommodate the desired gene. It should also contain specific sequences required for DNA insertion, such as promoter sequences specific to the gene of interest, inverted terminal repeats (ITR) for target site recognition in the genome, an antibiotic resistance gene, control elements, and genes essential for viral enzymes.
- Selection of vector: The vector selection is another important factor in gene therapy. The choice of vector depends on the type of gene to be inserted. Viral vectors, although they have excellent delivery rates and integration efficiency, may pose safety concerns. The selection of vectors also depends on the size of the gene of interest. For example, if the transgene size exceeds 35 kb, adenovirus vectors may not be recommended due to their lower gene carrying capacity. It is important to exercise caution while using viral vectors, especially retroviruses, due to their high infectivity.
- Selection of method: Once the gene of interest and the vector are selected, the next step is to determine the method of gene transfer. The choice of method depends on whether the target tissue is a somatic cell or other factors related to the specific transgene. For example, in vivo gene therapy methods work well for diseases like cystic fibrosis but may not be suitable for diseases like Duchenne muscular dystrophy (DMD) or AIDS. Ex vivo gene therapy provides greater precision for diseases like hemophilia. The selection of the method is based on the type of disease, type of transgene, type of tissue, and the chance of infection. In general, ex vivo gene therapy for AIDS is not recommended due to the high-risk factor associated with it.
- Selection of technique: The final step in the gene therapy process is the selection of a technique. If a non-viral vector is chosen as the method, techniques such as sonication, electroporation, magnetoception, gene gun, or liposome can be used. Since DNA cannot be directly inserted into host cells, pores need to be created on the cell surface to facilitate gene transfer. Electroporation is considered the best technique for all types of gene transfer experiments using non-viral vectors. It can also be combined with liposomes to enhance efficiency.
Experimental setup: To carry out gene therapy experiments, a highly contaminant-free, sterile, and sophisticated setup is required. State-of-the-art laboratories equipped with all necessary utilities and safety measures are essential for gene therapy research. It is crucial to perform the experiments under the supervision of experts to ensure accuracy and adherence to safety protocols.
Recent trends in gene therapy
Recent trends in gene therapy have shown significant progress and promise. Here are some key developments:
- FDA approval: In 2017, the FDA approved gene therapy for the first time, marking a major milestone in the field. The approval was granted for the treatment of sickle cell anemia, a monogenic blood disorder. This breakthrough demonstrated the potential of gene therapy for treating genetic diseases.
- Advancements in AAV vectors: Adenoviruses were previously used as vectors in gene therapy, but their harmful nature led scientists to explore safer alternatives. Adeno-associated viruses (AAV) have emerged as a preferred choice due to their ability to transfer genes to both dividing and non-dividing cells. AAV vectors are now being used in retinal gene therapy and cancer gene therapy, with promising results.
- Potential for cancer treatment: Ongoing trials on animal models have shown promising results for gene therapy in treating various types of cancer, including cystic fibrosis, Duchenne muscular dystrophy, hemophilia (A and B), thalassemia, severe combined immunodeficiency syndrome, and cancer. These advancements indicate that gene therapy holds potential for improving cancer treatment approaches.
- Future prospects with CRISPR-Cas9 and SB transposon system: The discovery and advancements in CRISPR-Cas9 gene editing technology and the SB transposon system have opened up new possibilities for gene therapy. These systems offer more precise and targeted approaches for gene editing and gene insertion, which can potentially enhance the effectiveness and safety of gene therapies.
Overall, recent trends in gene therapy indicate significant progress and a bright future for the field. While gene therapy may not be fully accessible for routine medical use in the present, ongoing advancements and research suggest that it holds great potential for the future. Continued exploration of innovative techniques and vectors, such as AAV and the use of CRISPR-Cas9 and SB transposon systems, is likely to pave the way for more effective and widely applicable gene therapies.
Some of the approved gene therapy
The FDA has recently approved several gene therapies for specific disorders. Here are some examples:
- Onasemnogene abeparvovec-xioi:
- Manufacturer: AveXis, Inc.
- This gene therapy is used for the treatment of spinal muscular atrophy (SMA) in pediatric patients under 2 years old who have bi-allelic mutations in the survival motor neuron 1 (SMN1) gene.
- For more information, you can refer to the licensing details provided by the manufacturer.
- Autologous Cellular Immunotherapy:
- Manufacturer: Dendreon Corporation.
- This gene therapy is employed for the treatment of prostate cancer in cases of asymptomatic or minimally symptomatic metastatic castrate-resistant prostate cancer.
- For additional information, including licensing details, you can refer to the provided resources.
- Voretigene neparvovec-rzyl:
- Manufacturer: Spark Therapeutics, Inc.
- This gene therapy is designed for the treatment of retinal dystrophy caused by biallelic RPE65 mutation.
- It is an adeno-associated virus (AAV) based gene therapy, requiring viable retinal cells for the therapy to be effective.
- For further details, including licensing information, you can consult the resources provided.
These approved gene therapies represent significant advancements in the field and offer targeted treatment options for specific disorders.
Applications of gene therapy
Gene therapy has the potential to revolutionize the treatment of various diseases and disorders by targeting the underlying genetic causes. Some of the applications of gene therapy include:
- Monogenic Disorders: Gene therapy holds promise for the treatment of monogenic disorders caused by mutations in a single gene. Diseases like cystic fibrosis, hemophilia, muscular dystrophy, and sickle cell anemia can potentially be treated through gene therapy approaches aimed at correcting or replacing the faulty gene.
- Cancer Treatment: Gene therapy can be used to treat cancer by targeting the genetic alterations that drive tumor growth. This can be achieved through different strategies, such as introducing therapeutic genes to suppress tumor growth, enhancing the immune system’s ability to recognize and destroy cancer cells (immunotherapy), or delivering genes that make cancer cells more susceptible to conventional therapies like chemotherapy or radiation.
- Genetic Eye Disorders: Gene therapy has shown promise in treating inherited retinal disorders, such as Leber congenital amaurosis and retinitis pigmentosa. By delivering functional genes directly to the retinal cells, vision loss can potentially be halted or improved.
- Neurological Disorders: Several neurological disorders, including Parkinson’s disease, Huntington’s disease, and Alzheimer’s disease, have a genetic component. Gene therapy approaches aim to deliver therapeutic genes to the affected areas of the brain to restore normal function, protect neurons, or slow down disease progression.
- Cardiovascular Diseases: Gene therapy can be used to treat cardiovascular diseases like coronary artery disease and heart failure. It involves delivering genes that promote blood vessel growth, enhance heart muscle function, or prevent the formation of arterial plaques, thereby improving blood flow and cardiac function.
- Infectious Diseases: Gene therapy can be utilized to combat infectious diseases by introducing genes that enhance the immune response against specific pathogens. It can also involve modifying a patient’s own cells to resist viral infections, such as HIV.
- Inherited Metabolic Disorders: Gene therapy has shown promise in treating inherited metabolic disorders, such as Gaucher disease and phenylketonuria. By delivering functional genes to the affected cells, the underlying metabolic defects can be addressed.
- Immunodeficiency Disorders: Gene therapy can be used to treat certain types of primary immunodeficiency disorders, such as severe combined immunodeficiency (SCID). By introducing functional genes into the patient’s immune cells, their ability to fight infections can be restored.
Limitations of gene therapy
Despite the potential of gene therapy, there are several limitations and challenges that need to be addressed:
- Immune Response: The use of viral vectors in gene therapy can trigger an immune response in the host, leading to inflammation or rejection of the vector. This immune response can limit the effectiveness of the therapy and potentially cause adverse effects.
- Transgene Expression: The transgene, once delivered to the target cells, may not be expressed consistently or at sufficient levels. Factors such as the choice of promoter sequences, gene silencing mechanisms, or the influence of the cellular environment can impact transgene expression, affecting the therapeutic outcome.
- Transient Effects: The effects of gene therapy can be transient, meaning that the therapeutic benefits may diminish over time. The transgene may not persist in the target cells, requiring repeated treatments or additional strategies to maintain long-term efficacy.
- Limited Target Cells: Not all cells within a specific tissue or organ may be accessible or receptive to the transgene. This can limit the effectiveness of gene therapy, particularly in diseases where a widespread or specific cellular targeting is necessary.
- Ethical Considerations: Gene transfer experiments involving live human embryos raise ethical concerns and are restricted in many countries. The potential risks and unknown long-term effects on the embryo’s development need to be carefully evaluated before considering such applications.
- Integration and Off-target Effects: The integration of the transgene into the host genome can occur randomly, potentially leading to unintended consequences. The transgene may disrupt essential genes or activate oncogenes, increasing the risk of adverse effects or tumorigenesis.
- High Cost: Gene therapy is currently an expensive treatment option, with costs averaging around $100,000 per therapy. This high cost poses challenges for accessibility and affordability, as insurance coverage may not always be available to cover these expenses.
These limitations highlight the need for further research and technological advancements to overcome the challenges associated with gene therapy. Continued efforts are necessary to improve the safety, long-term efficacy, and accessibility of gene therapy approaches for a wider range of diseases.
Challenges & future aspect of gene therapy
Gene therapy holds great promise for the treatment of various diseases, but it also faces several challenges. Here are some challenges and future aspects of gene therapy:
- Safety: Ensuring the safety of gene therapy is a crucial challenge. The use of viral vectors can lead to immune responses or adverse effects. Designing safer and more targeted vectors is important to minimize these risks.
- Targeted Insertion: Inserting the therapeutic gene at a specific location in the genome is challenging. Non-targeted insertions can disrupt normal gene function or lead to unintended consequences. Achieving precise and specific gene insertion is a key focus for future advancements.
- Oncogenic Potential: Gene therapy carries the risk of inadvertently increasing oncogene expression, potentially leading to the development of cancer. Preventing oncogenic activity and carefully monitoring the effects of gene therapy are essential to minimize this risk.
- Cost: The high cost of gene therapy poses a significant challenge in making it accessible and commercially viable. Efforts are needed to reduce the cost of gene therapy and explore strategies for insurance coverage and reimbursement.
- Future Applications: Gene therapy holds great potential for treating monogenic disorders and life-threatening conditions like cancer. Continued advancements in research and technology suggest progress in treating various diseases, such as cystic fibrosis, thalassemia, and Duchenne muscular dystrophy. However, careful consideration and ethical guidelines are necessary to prevent misuse of gene therapy for unnatural enhancements or capabilities.
- Uncertainty and Scattered Results: Predicting the future of gene therapy is challenging due to the uncertainty of outcomes and scattered results. The success of gene therapy depends on multiple factors, including the specific disease, delivery methods, and individual responses. Ongoing research and clinical trials are needed to better understand and refine gene therapy approaches.
In conclusion, while gene therapy has its challenges, it also presents promising opportunities for the treatment of genetic disorders and other diseases. Continued research, technological advancements, and ethical considerations are essential to address the challenges, improve safety, expand applications, and make gene therapy more accessible and effective for patients in the future.
FAQ
What is gene therapy?
Gene therapy is a therapeutic approach that involves introducing genetic material into a person’s cells to treat or prevent a disease. It aims to correct or replace a faulty gene, provide missing genetic material, or introduce new genes to improve cellular function.
How does gene therapy work?
Gene therapy typically involves delivering therapeutic genes into target cells using vectors, such as viruses or non-viral delivery systems. These genes can then produce functional proteins or RNA molecules to compensate for genetic defects, enhance the immune response, or modify cellular processes.
What types of diseases can gene therapy potentially treat?
Gene therapy holds promise for treating a wide range of diseases, including inherited genetic disorders, certain types of cancer, cardiovascular diseases, neurological disorders, and immune deficiencies. It has the potential to address diseases caused by specific gene mutations or abnormalities.
What are the different types of gene therapy vectors?
The commonly used vectors in gene therapy include viral vectors (such as retroviruses, adenoviruses, and lentiviruses) and non-viral vectors (such as liposomes, nanoparticles, and naked DNA). Each vector has its advantages and limitations in terms of efficiency, safety, and target cell specificity.
Is gene therapy safe?
Ensuring the safety of gene therapy is a primary concern. While significant advancements have been made, there can be risks associated with gene therapy, including immune responses, off-target effects, or unintended gene disruptions. Rigorous preclinical testing and clinical trials are conducted to evaluate safety before gene therapies are approved for human use.
Are there any successful gene therapy treatments available?
Yes, there have been successful gene therapy treatments. For example, gene therapy using adeno-associated virus vectors has shown success in treating certain types of inherited retinal disorders, such as Leber congenital amaurosis. Additionally, gene therapies for specific types of cancer, like CAR-T cell therapies, have shown promising results.
Can gene therapy be used to cure genetic diseases?
Gene therapy has the potential to provide a cure for some genetic diseases by addressing the underlying genetic cause. However, the success of gene therapy depends on various factors, including the specific disease, the effectiveness of gene delivery, and the ability to sustain long-term therapeutic effects.
How long do the effects of gene therapy last?
The duration of the therapeutic effects of gene therapy can vary. In some cases, the effects can be long-lasting or even permanent if the therapeutic gene is successfully integrated into the target cells and continues to be expressed. However, in other cases, the effects may be transient, requiring repeat treatments or additional interventions.
Are there any ethical considerations with gene therapy?
Ethical considerations in gene therapy include issues related to the use of human embryos, germline editing, privacy and consent, and the potential for misuse or enhancement beyond medical necessity. Regulations and guidelines are in place to address these ethical concerns and ensure responsible use of gene therapy.
Is gene therapy widely available?
Gene therapy is still an evolving field, and the availability of gene therapy treatments may vary depending on the country and specific disease. While some gene therapies have received regulatory approval, their availability and accessibility can be limited due to factors such as cost, infrastructure, and ongoing clinical research.