The chemical energy stored in molecules can be released as heat in chemical reactions that occur when the fuel methane, coal or cooking gas burns in the air. Chemical energy could also be utilized to carry out mechanical work when fuel is burned in an engine or to generate electric energy via an electrolytic cell similar to a dry cells. So, the various kinds of energy are interconnected and, under certain conditions, they may transform into a different form. The study of these transformations is the focus of thermodynamics. The thermodynamic laws cover energy changes in macroscopic systems with a vast amount of molecules instead of microscopic systems that contain a handful of molecules. Thermodynamics doesn’t care the manner in which and how fast the energy transformations performed, but rather is based on the initial and final conditions of the system that is undergoing a changes. The laws of thermodynamics only apply for systems that are at equilibrium or is moving between equilibrium and a different equilibrium. Properties of the macroscopic kind like temperature and pressure don’t change over time when an equilibrium state system.
Thermodynamics is concerned with the notions of temperature and heat, as well as the inter-conversion of heat as well as other types of energy. The laws that govern thermodynamics regulate the behavior of these variables and give a detailed description. William Thomson, in 1749 was the first to coin the term “thermodynamics”.
What is Thermodynamics?
- Thermodynamics is a branch of physics that encompasses the study of heat, work, temperature, and their relationship with energy, radiation, and the physical properties of matter. Coined by William Thomson in 1749, the term “thermodynamics” focuses on understanding how thermal energy can be converted to or from other forms of energy and how matter is influenced by these processes.
- At its core, thermodynamics explains the interconversion of heat and other forms of energy. Heat is generated by the movement of tiny particles within an object, with faster particle movement resulting in greater heat production. The field of thermodynamics does not concern itself with the specific mechanisms or rates at which these energy transformations occur, but rather focuses on the initial and final states of a system undergoing change.
- It is important to note that thermodynamics is a macroscopic science, meaning it deals with the overall behavior of a system rather than the molecular constitution of matter. By examining the bulk properties of substances, thermodynamics provides a quantitative description of energy-related phenomena.
- The laws of thermodynamics are a set of scientific principles that govern the behavior of thermodynamic systems in equilibrium. They establish relationships between various parameters such as temperature, energy, and entropy, and help define concepts like thermodynamic work and heat. These laws are empirical facts and are not only fundamental to thermodynamics but also have broader applications in physics and other natural sciences.
- Traditionally, three fundamental laws of thermodynamics were recognized and named the first law, the second law, and the third law. However, a more fundamental statement called the zeroth law was later established, completing the set of laws.
- The zeroth law of thermodynamics defines thermal equilibrium and serves as the basis for the concept of temperature. It states that if two systems are each in thermal equilibrium with a third system, then they are in thermal equilibrium with each other.
- The first law of thermodynamics, also known as the law of conservation of energy, states that when energy enters or leaves a system as work, heat, or matter, the internal energy of the system changes accordingly.
- The second law of thermodynamics states that in a natural thermodynamic process, the sum of the entropies of the interacting systems involved never decreases. A common implication of this law is that heat does not spontaneously flow from a colder body to a warmer body.
- The third law of thermodynamics asserts that as the temperature of a system approaches absolute zero, its entropy approaches a constant value. Although exceptions exist for non-crystalline solids, the entropy of a system at absolute zero is typically close to zero.
- These laws prevent the possibility of perpetual motion machines, with the first law prohibiting the production of work without energy input, and the second law forbidding the spontaneous conversion of thermal energy into mechanical work.
- The history of thermodynamics is closely intertwined with the development of physics and chemistry, dating back to ancient theories of heat. The laws of thermodynamics were established through progress made in the 19th and early 20th centuries. Sadi Carnot’s work in the 19th century laid the foundation for the second law, while scientists like Rudolf Clausius and William Thomson formalized the first and second laws by 1860. Walther Nernst later formulated what is now known as the third law between 1906 and 1912. Although there have been suggestions for additional laws, the four accepted laws of thermodynamics remain the most widely recognized and discussed in standard textbooks.
Definition of Thermodynamics
Thermodynamics is the branch of physics that studies the interconversion of heat and energy and the behavior of systems in relation to temperature, work, and entropy.
Definitions of Gibb’s Free Energy
Gibb’s free energy, often denoted as G, is a thermodynamic potential that measures the maximum reversible work that can be performed by a system at constant temperature and pressure. It is named after Josiah Willard Gibbs, an American physicist and mathematician who made significant contributions to thermodynamics.
Gibb’s free energy is defined in terms of other thermodynamic quantities, such as enthalpy (H), entropy (S), and temperature (T). The relationship between these quantities can be expressed by the equation:
G = H – TS
where:
- G is the Gibb’s free energy of the system,
- H is the enthalpy of the system,
- T is the absolute temperature,
- S is the entropy of the system.
The Gibb’s free energy accounts for both the energy content of the system (enthalpy) and the dispersal of energy (entropy) due to thermal fluctuations. It provides insight into the spontaneity and equilibrium of a chemical or physical process.
The sign of Gibb’s free energy change (ΔG) determines the spontaneity of a process:
- If ΔG < 0, the process is thermodynamically favorable and tends to occur spontaneously. The system releases energy, and the process is exergonic.
- If ΔG > 0, the process is thermodynamically unfavorable and does not tend to occur spontaneously. The system requires an input of energy, and the process is endergonic.
- If ΔG = 0, the process is at equilibrium. The system is in a state of maximum stability, and there is no net change in the system.
Gibb’s free energy is a valuable tool in analyzing and predicting the direction and feasibility of chemical reactions, phase transitions, and other thermodynamic processes. It provides insights into the energy and entropy balance of a system, allowing for the optimization and understanding of various thermodynamic phenomena.
Branches of Thermodynamics
Thermodynamics, as a scientific discipline, encompasses several branches that focus on different aspects and applications. The main branches of thermodynamics are as follows:
- Classical Thermodynamics: This branch of thermodynamics analyzes the behavior of matter using a macroscopic approach. It considers properties such as temperature and pressure to calculate other thermodynamic properties and predict the characteristics of the matter undergoing a process. Classical thermodynamics provides a framework for understanding energy transfer, work, and heat in various systems.
- Statistical Thermodynamics: Statistical thermodynamics takes a microscopic perspective by studying the properties and interactions of individual molecules. It aims to describe the behavior of a large group of molecules and derive macroscopic properties from their statistical distribution. This branch employs statistical mechanics to understand the relationships between the microscopic structure and macroscopic behavior of systems.
- Chemical Thermodynamics: Chemical thermodynamics focuses on the study of energy changes and heat transfer in chemical reactions and phase changes of substances. It examines the thermodynamic properties of chemical compounds, their reactions, and the associated energy changes. Chemical thermodynamics plays a crucial role in understanding and predicting the feasibility and spontaneity of chemical reactions.
- Equilibrium Thermodynamics: Equilibrium thermodynamics deals with the transformations of energy and matter as they approach a state of equilibrium. It provides a framework to analyze systems in a stable, balanced state, where there are no net changes occurring. Equilibrium thermodynamics allows for the determination of thermodynamic properties and the establishment of relationships between different variables under equilibrium conditions.
These different branches of thermodynamics provide distinct perspectives and approaches to understanding the behavior of energy, matter, and systems. They collectively contribute to the comprehensive study and application of thermodynamics in various scientific and engineering fields.
Basic Concepts of Thermodynamics – Thermodynamic Terms
System
A thermodynamic system refers to a specific portion of matter that is the focus of attention in thermodynamics. It is characterized by a definite boundary, which can be real or imaginary, fixed or deformable. There are three main types of thermodynamic systems: isolated systems, closed systems, and open systems.
- Isolated System: An isolated system is one that cannot exchange energy or mass with its surroundings. The universe itself is often considered an isolated system, as it is not influenced by external factors.
- Closed System: A closed system allows for the transfer of energy across its boundary, but does not permit the transfer of mass. In other words, no material enters or leaves the system. Examples of closed systems include a refrigerator or the compression of gas in a piston-cylinder assembly.
- Open System: An open system is one in which both mass and energy can be exchanged between the system and its surroundings. Material can enter or exit the system, and energy can be transferred in the form of work or heat. A steam turbine is an example of an open system, as steam enters the turbine, performs work, and exits the system.
The interactions of thermodynamic systems differ based on their type:
- Isolated System: In an isolated system, there is no exchange of mass, work, or heat with the surroundings. The system is self-contained and does not interact with external entities.
- Open System: An open system allows for the flow of mass into and out of the system. Additionally, work can be done on or by the system, and heat transfer can occur between the system and its surroundings.
- Closed System: A closed system does not allow for the transfer of mass, but work and heat transfer can take place across the system’s boundary. Work can be done on or by the system, and heat can be exchanged with the surroundings.
Understanding the type of thermodynamic system is essential in analyzing and predicting the behavior of energy and matter within a given system and its interactions with the surroundings.
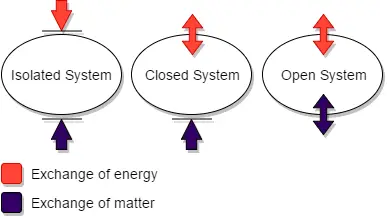
Type of system | Mass flow | Work | Heat |
Isolated System | ☓ | ☓ | ☓ |
Open System | ✓ | ✓ | ✓ |
Closed System | ☓ | ✓ | ✓ |
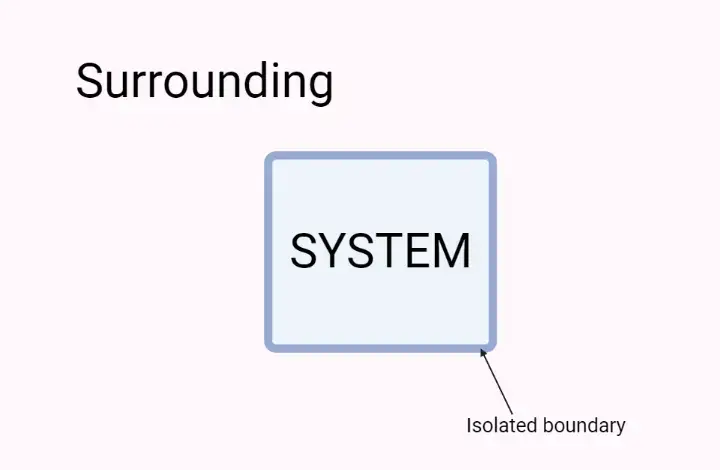
Surrounding
- In thermodynamics, the term “surroundings” refers to everything outside of the system that has a direct influence on the behavior and actions of the system. It encompasses all the components and factors external to the system that interact with it.
- The system and its surrounding together form the universe. The surroundings can be considered as the environment in which the system exists. While the entire universe, excluding the system, is not directly affected by changes within the system, the surroundings have a significant impact on the system’s behavior.
- For example, let’s consider a reaction taking place in a glass beaker containing substances A and B. In this scenario, the beaker and its contents constitute the system, while the space in which the beaker is placed is the surrounding. The surrounding plays a role in influencing the reaction by providing factors such as temperature, pressure, and potential interactions with other substances.
- It is important to establish a boundary between the system and its surroundings, whether it is a physical boundary like the walls of a container or an imaginary boundary defined by coordinates in space. This boundary allows us to monitor and analyze the exchange of energy and matter between the system and its surroundings.
- By considering the system and its surrounding as distinct entities, thermodynamics enables the study and understanding of how energy and matter flow into, out of, and interact within a system in response to changes in the surrounding conditions.
Process of Thermodynamic
The process of thermodynamics involves the energetic changes that occur within a system, specifically associated with variations in pressure, volume, and internal energy. Different types of thermodynamic processes exist, each with its unique characteristics:
- Adiabatic Process: This process occurs when there is no heat transfer into or out of the system. In an adiabatic process, the system undergoes changes in pressure, volume, and internal energy without any heat exchange with the surroundings.
- Isochoric Process: An isochoric process, also known as an isovolumetric process, is characterized by no change in volume. In this process, the system remains at a constant volume while energy can be transferred as heat or work. No work is done by the system in terms of volume change.
- Isobaric Process: An isobaric process is one in which the pressure remains constant. The system can undergo changes in volume and internal energy, while the pressure remains unchanged. This process may involve heat transfer or work done on or by the system.
- Isothermal Process: An isothermal process occurs when the temperature of the system remains constant. In this process, the system experiences changes in volume and pressure while maintaining a constant temperature. Heat can enter or leave the system to maintain thermal equilibrium.
Additionally, a thermodynamic cycle refers to a series of processes or a combination of processes in which the initial and final states of the system are the same. It is a closed loop of operations that can be repeated. Thermodynamic cycles, also known as cyclic operations or cyclic processes, play a vital role in various applications, such as heat engines and refrigeration systems.
What is Thermodynamic Equilibrium?
Thermodynamic equilibrium refers to the state of a system in which all its properties have fixed values, and no changes occur when the system is isolated from its surroundings. It is characterized by the absence of any spontaneous macroscopic changes in the system.
There are several types of equilibrium that can be achieved within a thermodynamic system:
- Thermal Equilibrium: When the temperature is the same throughout the entire system, it is considered to be in thermal equilibrium. In this state, there is no net heat flow within the system, and all parts of the system are at the same temperature.
- Mechanical Equilibrium: Mechanical equilibrium is achieved when there is no change in pressure at any point within the system. In other words, the forces acting within the system are balanced, and there is no net force or pressure gradient.
- Chemical Equilibrium: Chemical equilibrium is attained when the chemical composition of the system does not vary with time. It occurs when the rates of forward and backward reactions in a chemical system are equal, resulting in a stable composition.
- Phase Equilibrium: Phase equilibrium refers to the equilibrium between different phases of matter within a system. In a two-phase system, phase equilibrium is reached when the mass of each phase reaches a stable and constant level.
For a thermodynamic system to be in thermodynamic equilibrium, it must simultaneously satisfy thermal equilibrium, mechanical equilibrium, and chemical equilibrium. In this state, all relevant parameters, such as temperature, pressure, composition, and phase distribution, remain constant over time.
Thermodynamic equilibrium is a state of balance and stability, where no spontaneous changes occur within the system. It is a fundamental concept in thermodynamics that allows for the analysis and prediction of system behavior under specific conditions.
Properties of Thermodynamic
Thermodynamic properties are defined as the most significant characteristics of a system which are capable of describing the state of the system. These thermodynamic characteristics of a substance can be classified in a variety of ways.
- Measured properties: The properties of the system that are directly accessible from the laboratory are called measured properties. Examples are temperature, volume and pressure.
- Fundamental properties: The properties of the system directly linked to the thermodynamic laws that are fundamental to the system are called fundamental properties. Examples include internal energy and Entropy
- Derived properties: Property of the system that exhibit particular relations and consist of the combination with measured as well as derived properties are referred to as derived properties. Examples include: Gibbs, Enthalpy Free energy.
System’s thermodynamic properties can be classified into two classes:
- Extensive property: The value of an extensive property’s value is determined by the amount or size of the matter within the system. However, the large variables can help define the system under study. Examples: volume, mass in-built energy and enthalpy heat capacity and entropy, the Gibbs Free Energy.
- Intensive property: The ones which do not depend on the size or quantity of matter are referred to as properties that are intensive. The intensity property can vary between different locations in the entire system at any time. Examples of this are: Density, Pressure temperature, specific volume specific entropy, thermal Conductivity Thermal Expansion, Compressibility and numerous other.
Thermodynamic state
The thermodynamic condition of a system is determined by defining the values of a set specific properties that can be measured to establish all other aspects. Fluid systems the most common properties are volume, pressure and temperature. Complex systems might necessitate the specification of unique characteristics. For instance the condition that an electrical battery is in demands to be specified in terms of quantity of charge it has.
Properties can be extensive or intense. The properties that are extensive are also additive. Therefore, if the system is broken down into several sub-systems, then the worth of the property across the whole system is the value of all the components. Volume is an expansive property. Intense properties don’t depend on the amount of matter that is present. Pressure and temperature are both intensive properties.
Specific properties are properties that are complex per unit mass . They are indicated by lower cases letters. Examples:
specific volume = V/m = v
Specific properties are in-depth because they don’t depend on the size of the system.
The basic properties of a system are the same across the entire system. However, in general the characteristics of a system could differ from one point to the next. It is common to study a system’s general properties by subdividing the entire system (either conceptually or actually) into several simple systems, in each of which the properties are believed to be the same.
It is crucial to remember that properties are only used to describe states at the point of equilibrium.
Laws of Thermodynamics
Thermodynamics laws describe the essential physical quantities like energy temperature, and entropy that define thermodynamic systems in equilibrium temperature. The thermodynamics laws define the way these quantities work in various conditions.
There are four thermodynamic laws and are listed in the following:
- Zeroth law of thermodynamics
- First law of thermodynamics
- Second law of thermodynamics
- Third law of thermodynamics
1. Zeroth law of thermodynamics
The Zeroth law of thermodynamics states that if two bodies are individually in equilibrium with a separate third body, then the first two bodies are also in thermal equilibrium with each other.
This means that if A is in equilibrium with C, as well as system B in equilibrium with C, then systems A as well as B are in equilibrium with thermal energy.
An example of Zeroth Law
Think about 2 cups B and A filled with boiling water. If a thermometer is put inside cup A will be heated through the water to read 100°C. If it reads 100 degrees Celsius then we can say that thermometer was in equilibrium cup A. If we move the thermometer into cup B in order to measure the temperature it will continue to read 100 degrees Celsius. It is in equilibrium also with the cup. If we keep in mind the law that is zero in thermodynamics, one can say that cups A and B both are both in equilibrium with one another.
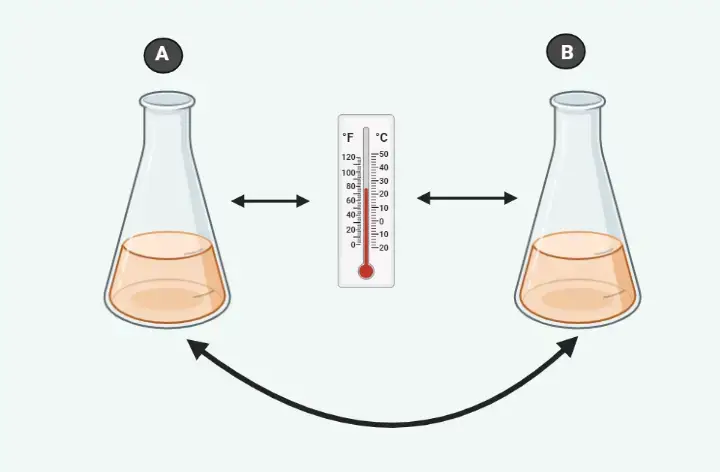
A thermodynamic law that is zeroth in its application permits us to make use of thermometers to determine the temperature of two objects we would like.
2. First law of thermodynamics
The First Law of Thermodynamics, also known as the law of conservation of energy, is a fundamental principle in thermodynamics. It states that energy within a closed system is conserved and cannot be created or destroyed. However, energy can be transferred from one form to another or converted between different forms.
To understand the First Law of Thermodynamics, let’s consider a few examples:
- Photosynthesis: Plants convert radiant energy from sunlight into chemical energy through the process of photosynthesis. They store this energy in the form of carbohydrates. When we consume plants, the chemical energy is transferred to our bodies. We then convert this chemical energy into kinetic energy as we engage in activities like swimming, walking, breathing, or even scrolling through a page.
- Electrical Energy: When we switch on a light, it may appear as if energy is being created. However, the First Law of Thermodynamics tells us that this is not the case. The electrical energy provided to the light bulb is converted into other forms of energy, such as light energy and heat energy. The total amount of energy remains constant, but its form changes.
These examples demonstrate the principle that energy can be transformed or transferred between different forms, but the total energy within a closed system remains constant. This law of conservation of energy is a fundamental concept in thermodynamics and has wide-ranging applications in various fields of science and engineering.
The First Law of Thermodynamics provides a basis for understanding energy flow, heat transfer, and work in thermodynamic systems. It forms the foundation for the study of energy conversion, efficiency, and the relationships between different forms of energy. By applying this law, scientists and engineers can analyze and predict the behavior of systems, ensuring the proper utilization and management of energy resources.
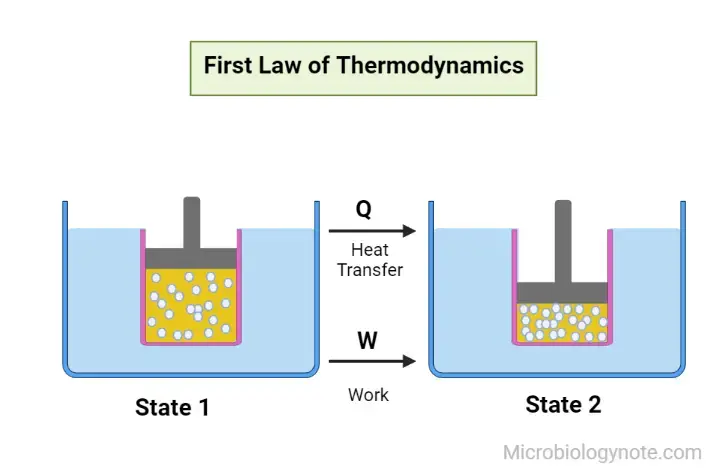
As per this law, some of the heat that is supplied to the system is utilized to alter the energy within the system, while the remainder is utilized in performing the work of the system.
It is mathematically represented as
Where,
- ΔQ is the heat given or lost
- ΔU is the change in internal energy
- W is the work done
We can also represent the above equation as follows,
Therefore, we can deduce from the equation above that the amount (ΔQ – W) is not dependent on the route taken to alter the state. Furthermore, we can conclude that the internal energy will increase when heat is transferred to the system, and the reverse is true.
Sign Conventions
The table below illustrates the sign conventions that are appropriate for the three quantities in different circumstances:
ΔU (change in internal energy) | Q (heat) | W (work done on the gas) |
is “+” if temperature increases | is “+” if heat enters gas | is “+” if gas is compressed |
is “-” if temperature decreases | is “-” if heat exist gas | is “-” if gas expands |
is “0” if temperature is constant | is “0” if no heat is exchanged | is “0” if volume is constant |
Examples of First Law Of Thermodynamics
- Plants convert the energy from sunlight into chemical energy by photosynthesis. We eat plants , and transform this chemical energy to kinetic energy when we walk, swim in the air, breathe, and browse this page.
- Turning on the lights may appear to generate energy, but it’s the electrical energy that is converted.
2. Second Law of Thermodynamics
Second law of thermodynamics states that the entropy in an isolated system always increases. Any isolated system spontaneously evolves towards thermal equilibrium—the state of maximum entropy of the system.
Second law of thermodynamics stipulates that any event that happens spontaneously is always accompanied by an increase in the amount of entropy (S) in the world. Simply put this law states that the entropy of a system isolated is never going to decrease as time passes by.
However, in certain situations where the system is at equilibrium in thermodynamics or is going through an irreversible process, the total entropy of the system and its surrounding remains in a constant state. Second law called”the Law of Increased Entropy.
This second rule clearly shows that it is not possible in converting heat into mechanical energy with 100 % effectiveness. As an example, if we take a look at the piston of an engine it is heated in order to increase the pressure and power a piston. But, as the piston is moving there’s always left-over heat which is not used for doing any other task. It is wasted heat and is discarded. In this scenario this is accomplished by moving it to a sink, or in the case of an engine in a car it is removed by releasing the gas and the air mix into the air. In addition, heat produced by friction, which is usually not usable, should be removed off the engine.
Second Law of Thermodynamics Equation
Mathematically, the second law of thermodynamics is represented as;
ΔSuniv > 0
where ΔSuniv is the change in the entropy of the universe.
Entropy is a measurement of the randomness of the system , or it’s the measure the amount of energy, or even chaos in the system. It can be viewed as an index of quantitative value that defines the energy quality.
In addition, there are several causes that result in an increase in the entropy of an enclosed system. In closed systems, although the mass stays constant, there is a exchange of heat between the surrounding. This shift in the temperature content causes disturbances within the system, thereby increasing the energy content of the system.
Additionally, internal changes can be observed in the movement of the molecules within the system. This can cause disturbances that create irreversible effects within the system, leading to the increase in its the entropy.
Different Statements of The Law
Two statements are made regarding the second law of thermodynamics that are
1. Kelvin- Plank Statement
- It is not possible for the heat engine to build the network over a full cycle if it is able to exchange heat only with other bodies with a single temperature.
- Exceptions: If Q2 =0 (i.e., Wnet = Q1, or efficiency=1.00), The heat engine creates work throughout the entire cycle by exchanging heat just one reservoir, thereby in violation of the Kelvin-Planck assertion.
2. Clausius Statement
It is difficult to build devices operating in a circular cycle that could transfer the warmth of a colder object to a warmer one , without needing any effort. Furthermore, energy won’t be transferred from a low temperature object to a high-temperature object. It is crucial to remember that we’re referring to the transfer of energy. The transfer of energy can occur between a cold object and hot objects through the energy particles, and electromagnetic radiation. But the net transfer of energy will happen between the warm and cold one in any process that is spontaneous. Some form of work is required to transfer the heat to the cold object. Also, if the compressor is controlled from an external power source, the refrigerator will not be able operate. The heat pump and the refrigerator are based on Clausius’s assertion.
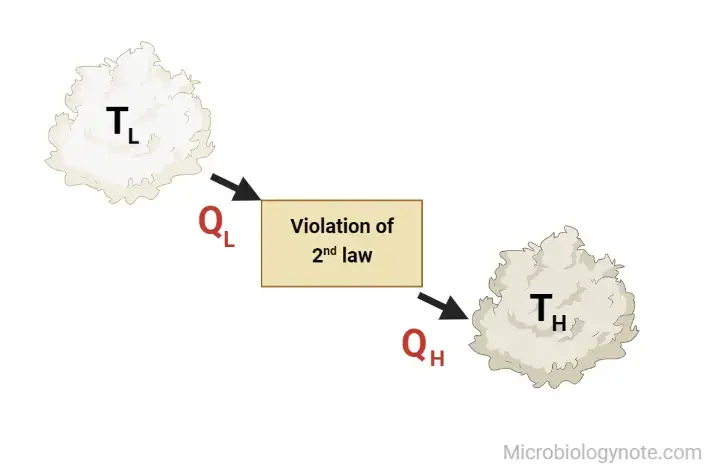
Both statements of Kelvin and Clausius are identical i.e devices that violate Clausius’s assertion will also violate Kelvin’s claim and vice the reverse.
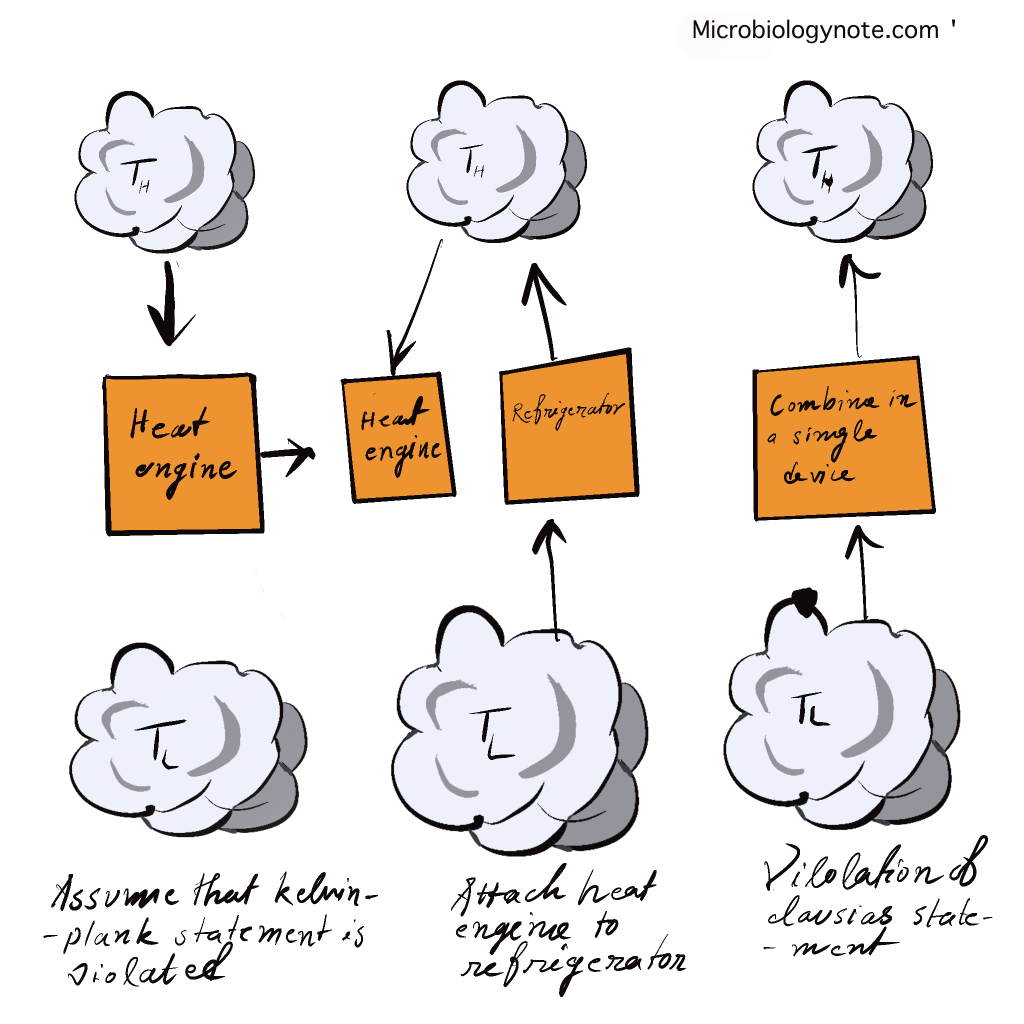
Alongside these claims in addition to these statements, the French scientist by the name of Nicolas Leonard Sadi Carnot also called”the “father of thermodynamics,” was the one who invented his Second Law of Thermodynamics. But, according to his assertion, he stressed the necessity of using caloric theories in the formulation of the law. Caloric (self repellent liquid) refers to heat. Carnot noticed that some caloric is removed during the motion.
Perpetual Motion Machine of the Second Kind (PMM2)
The device that generates work in conjunction with a single reservoir of heat is called one of the perpetual motion machines of second variety (PMM2). Also, a machine that does not conform to two laws of thermodynamics can be described as a permanent movement machine second variety.
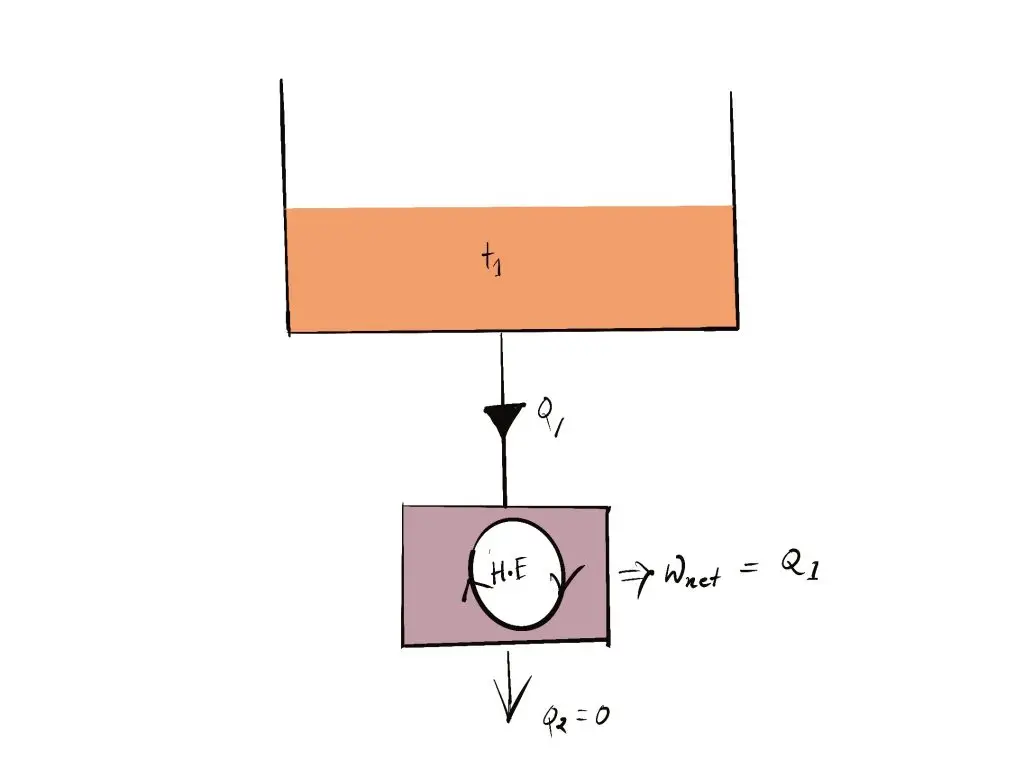
So, a heating engine must interact with at minimum two thermal reservoirs of different temperatures to generate work during the course of a cycle. In the event of an inconsistency in temperature, motivation power (i.e. work) is produced. If the bodies that exchange heat have finite capacities for heat it will produce work by the heat engine up to the temperature of both bodies is equalized.
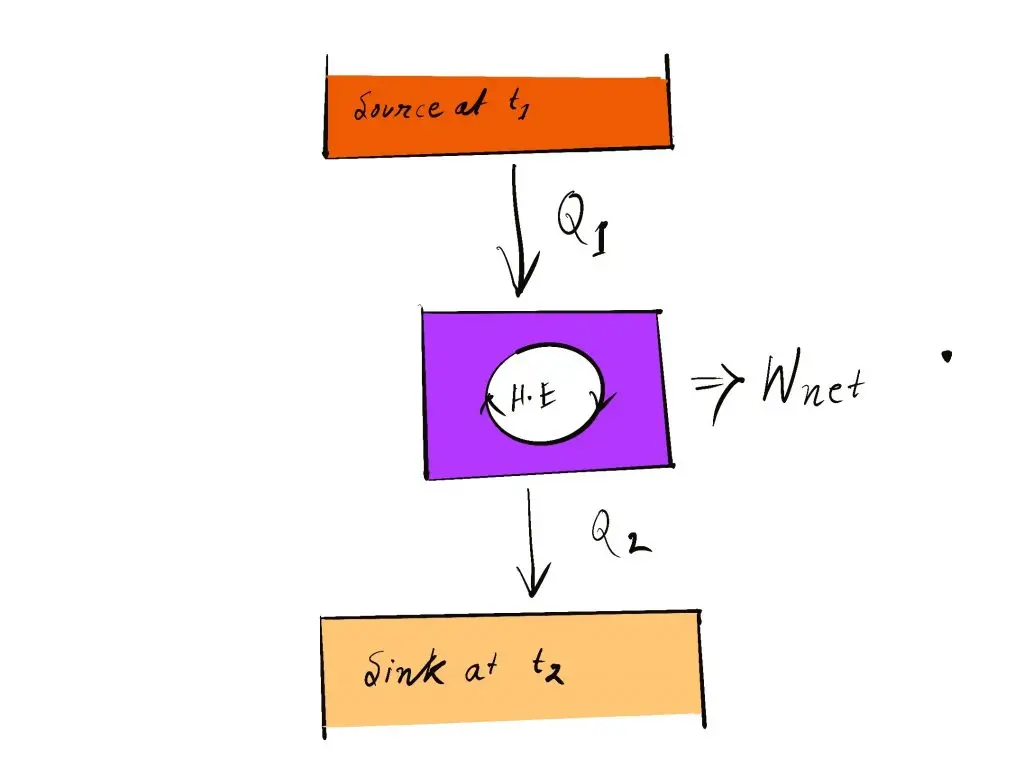
Examples of the second law of thermodynamics
If a space isn’t cleaned or tidied then it will become messy and chaotic with time. If the room is clean the entropy of the room decreases however, the effort required to clean has led to an increase in the entropy of the space, which is greater than the amount of entropy lost.
3. Third Law Of Thermodynamics
Third law of thermodynamics states that the entropy of a system approaches a constant value as the temperature approaches absolute zero.
Entropy, as indicated by the letter ‘S’, is a measure for disorder or randomness of an enclosed system. It is directly proportional to the number of microstates (a permanent microscopic state that is utilized by an entire system) that the system is able to access, i.e. the more microstates a closed system can be able to occupy, the higher its energy consumption. The microstate at which the system’s energy is at its lowest is called the ground state the system.
At temperatures of zero Kelvin the following phenomena are observed in a closed system
- The system doesn’t contain any heating.
- All the molecules and atoms within the entire system remain at their lowest energie levels.
Thus, a system that is that is at zero absolute has one microstate that is accessible – its the ground state. In accordance with the thermodynamic third law the entropy of the system is absolutely zero. The law was developed by the German scientist Walther Nernst between the years 1906 between 1912 and 1906.
Alternate Statements of the 3rd Law of Thermodynamics
The Nernst statement of the third law of thermodynamics implies that it is not possible for a process to bring the entropy of a given system to zero in a finite number of operations.
The American physical chemical chemists Merle Randall as well as Gilbert Lewis stated this law differently according to the following formula: when the entropy value of every element (in their perfectly crystallized states) is taken to be 0 when the temperature is absolute and the entropy for every substance has to be positive and finite value. However, the entropy of a substance at absolute zero could be zero, which happens when a perfectly formed crystal is taken into consideration.
The Nernst-Simon formulation in the third law of thermodynamics could be written in the form the following: for a condensed system undergoing an isothermal process that is reversible in nature, the associated entropy change approaches zero as the associated temperature approaches zero.
A further implication that the law 3 has the fact that The exchange of energy among two thermodynamics systems (whose combination forms one isolated unit) is restricted.
Mathematical Explanation of the Third Law
According to statistics the entropy in an entire system can be calculated using the following equation:
S – S0 = 𝑘B ln𝛀
Where,
- S is the entropy of the system.
- S0 is the initial entropy.
- 𝑘B denotes the Boltzmann constant.
- 𝛀 refers to the total number of microstates that are consistent with the system’s macroscopic configuration.
Now, for a perfect crystal that has exactly one unique ground state, 𝛀 = 1. Therefore, the equation can be rewritten as follows:
S – S0 = 𝑘B ln(1) = 0 [because ln(1) = 0]
When the initial entropy of the system is selected as zero, the following value of ‘S’ can be obtained:
S – 0 = 0 ⇒ S = 0
Thus, the entropy of a perfect crystal at absolute zero is zero.
What is Enthalpy?
- Enthalpy is a fundamental concept in thermodynamics that measures the total energy content of a system. It is denoted by the symbol H and represents the sum of the internal energy (E) of the system and the product of its pressure (P) and volume (V).
- Mathematically, the equation that defines enthalpy is H = E + PV. This equation illustrates that enthalpy takes into account both the internal energy of the system and the work done on or by the system due to changes in pressure and volume.
- Enthalpy is often referred to as the “heat content” of a system because it includes both the internal energy and the energy associated with the system’s volume and pressure. It represents the total amount of energy available within the system to do work or transfer heat.
- One important property of enthalpy is that it is a state function, meaning that its value depends only on the current state of the system and not on the path taken to reach that state. This allows for the convenient use of enthalpy in thermodynamic calculations and analysis.
- Enthalpy is particularly useful in studying processes that involve heat transfer or changes in pressure and volume, such as chemical reactions, phase transitions, and heat transfer in engineering systems. In exothermic reactions, where heat is released, the enthalpy change is negative, indicating a decrease in enthalpy. In endothermic reactions, where heat is absorbed, the enthalpy change is positive, indicating an increase in enthalpy.
- Enthalpy is commonly used in practical applications, such as calculating heat transfer in industrial processes, designing heating and cooling systems, and determining the energy requirements for chemical reactions. It provides a valuable tool for understanding and quantifying energy changes in various thermodynamic systems.
- Overall, enthalpy provides a comprehensive measure of the energy content of a system, incorporating both internal energy and energy associated with pressure and volume. Its application extends to a wide range of fields, allowing for a deeper understanding of energy transformations and enabling the analysis and optimization of various processes and systems.
What is entropy in thermodynamics?
- Entropy is a fundamental concept in thermodynamics that measures the degree of randomness or disorder in a system. It is denoted by the symbol S and is a thermodynamic quantity that depends on the physical state or condition of the system.
- In simple terms, entropy can be understood as a measure of how dispersed or spread out the energy or matter is within a system. It quantifies the level of randomness or unpredictability in the arrangement and distribution of particles or components within the system.
- To illustrate this concept, let’s consider the example of a solid and a gas. In a solid, the particles are tightly packed and have limited freedom of movement. The arrangement of particles is more ordered, and there is less randomness in their positions and motions. Therefore, the entropy of a solid is lower compared to that of a gas.
- In contrast, in a gas, the particles have greater freedom of movement and are able to fill the available space within the container. The particles are more randomly distributed, and their positions and velocities are less predictable. As a result, the entropy of a gas is higher than that of a solid.
- The concept of entropy is closely related to the concept of energy dispersal. In a system with high entropy, the energy or matter is more dispersed or spread out, leading to greater randomness and disorder. Conversely, in a system with low entropy, the energy or matter is more concentrated or ordered.
- Entropy plays a crucial role in understanding various thermodynamic processes, including heat transfer, energy conversion, and the direction of spontaneous changes. The Second Law of Thermodynamics states that the entropy of an isolated system tends to increase over time. This principle is often referred to as the “arrow of time” and explains why certain processes are irreversible and tend to move towards a state of greater disorder.
- Entropy is a concept that has applications in fields beyond thermodynamics, including information theory and statistical mechanics. It provides insights into the behavior and transformations of systems and helps us understand the fundamental principles governing the physical world.
Thermodynamics Examples in Daily Life
No matter if we’re in a cool room or in a vehicle The application of thermodynamics is all around us. Here are some of these applications below:
- The various kinds of vehicles like trucks, planes and ships are based on the 2nd law of thermodynamics.
- The three types of heat transfer operate in accordance with thermodynamics. The theories of heat transfer are used extensively in heaters, radiators, and coolers.
- Thermodynamics plays a role with the research of various types of power plant, including thermal power plants, nuclear power plants and power plants and others.
What is the mathematical relationship Betweeb Gibb’s Free Energy, enthalpy, and Entropy?
The mathematical relationship among Gibbs free energy (G), enthalpy (H), and entropy (S) is given by the Gibbs free energy equation, which is derived from the second law of thermodynamics. The equation relates these three thermodynamic properties at constant temperature (T) and pressure (P).
The Gibbs free energy equation is as follows:
ΔG = ΔH – TΔS
where:
- ΔG represents the change in Gibbs free energy.
- ΔH represents the change in enthalpy.
- ΔS represents the change in entropy.
- T is the absolute temperature.
The equation quantifies the balance between the energy content of a system (enthalpy) and the dispersal of energy (entropy) due to thermal fluctuations.
By analyzing the signs of the ΔG, ΔH, and ΔS values, we can determine the spontaneity and equilibrium of a process:
- If ΔG < 0: The process is thermodynamically favorable and tends to occur spontaneously. The system releases energy, and the process is exergonic.
- If ΔG > 0: The process is thermodynamically unfavorable and does not tend to occur spontaneously. The system requires an input of energy, and the process is endergonic.
- If ΔG = 0: The process is at equilibrium. The system is in a state of maximum stability, and there is no net change in the system.
Additionally, we can express the Gibbs free energy equation in terms of the Gibbs free energy change per mole (ΔG°), enthalpy change per mole (ΔH°), and entropy change per mole (ΔS°) at standard conditions (often denoted by the superscript “°”):
ΔG° = ΔH° – TΔS°
The standard conditions typically refer to a temperature of 298 K (25°C) and a pressure of 1 bar.
The relationship between Gibbs free energy, enthalpy, and entropy is fundamental in thermodynamics, enabling the analysis and prediction of the spontaneity, equilibrium, and energy changes associated with various chemical reactions and processes.
Standard free energy change and equilibrium constant Coupled reactions and additive nature of standard free energy change
Standard free energy change (ΔG°) is a thermodynamic parameter that provides information about the spontaneity and feasibility of a chemical reaction under standard conditions. It is related to the equilibrium constant (K) of a reaction through the equation:
ΔG° = -RT ln(K)
where:
- ΔG° is the standard free energy change,
- R is the gas constant (8.314 J/(mol·K) or 0.008314 kJ/(mol·K)),
- T is the temperature in Kelvin,
- ln denotes the natural logarithm,
- K is the equilibrium constant.
The equation shows that the standard free energy change is directly related to the logarithm of the equilibrium constant. A negative ΔG° value indicates a thermodynamically favorable reaction, and a positive ΔG° value indicates an unfavorable reaction.
Coupled reactions involve the linking of two or more reactions so that the overall process becomes more thermodynamically favorable. The principle behind coupling reactions is that the overall free energy change of the coupled process is the sum of the individual free energy changes. If a reaction has a positive ΔG° (unfavorable), it can be coupled with another reaction that has a negative ΔG° (favorable) to yield a net negative ΔG° for the overall process.
The additive nature of standard free energy change is a consequence of the fact that free energy is a state function. This means that the overall free energy change for a series of reactions or a multi-step process is equal to the sum of the individual free energy changes. For example, if a reaction can be divided into a series of steps, the overall ΔG° of the reaction is the sum of the ΔG° values for each step.
This additive nature of ΔG° allows for the analysis and prediction of complex reaction networks. It enables the determination of the overall thermodynamic feasibility of a pathway by summing the individual free energy changes for each step or reaction.
In summary, the standard free energy change is related to the equilibrium constant through a logarithmic relationship. Coupled reactions can enhance overall thermodynamic favorability, and the additive nature of standard free energy change allows for the determination of the overall free energy change in multi-step processes or reaction networks.
What is q in thermodynamics?
In thermodynamics, “q” represents heat transfer. Heat transfer, denoted as “q,” is the transfer of thermal energy between two objects or systems due to a temperature difference. It is a form of energy transfer that occurs spontaneously from a higher-temperature region to a lower-temperature region until thermal equilibrium is reached.
The symbol “q” is used to represent the amount of heat transferred during a thermodynamic process. It can have both positive and negative values, depending on the direction of heat transfer. A positive value of q indicates that heat is transferred into the system, increasing its internal energy, while a negative value of q indicates heat transfer out of the system, reducing its internal energy.
The heat transfer, q, can occur through different mechanisms: conduction, convection, and radiation. Conduction is the transfer of heat through direct molecular interactions within a solid or between solids in contact. Convection is the transfer of heat through the motion of a fluid (liquid or gas), where hot particles rise and cool particles sink, creating a circulation pattern. Radiation is the transfer of heat through electromagnetic waves, such as infrared radiation.
The measurement of heat transfer, q, is typically expressed in units of joules (J) in the International System of Units (SI). In some contexts, calories (cal) or British thermal units (BTU) may also be used as units of heat.
In thermodynamic calculations and equations, the symbol “q” is often employed to represent the heat transfer term, which is an essential aspect of analyzing and understanding energy transformations in various thermodynamic processes.
What is cp in thermodynamics?
In thermodynamics, “cp” refers to specific heat capacity at constant pressure. Specific heat capacity is a measure of the amount of heat energy required to raise the temperature of a unit mass of a substance by a certain amount.
The specific heat capacity at constant pressure, cp, is defined as the amount of heat required to raise the temperature of a substance by 1 degree Celsius or 1 Kelvin while keeping the pressure constant. It is expressed in units of energy per unit mass per degree Celsius (or Kelvin), such as joules per kilogram per degree Celsius (J/kg°C) or calories per gram per degree Celsius (cal/g°C).
The specific heat capacity at constant pressure is particularly relevant in processes that occur under constant pressure conditions, such as many practical applications in engineering and physics. It helps in determining the heat transfer and energy changes associated with these processes. The value of cp depends on the substance being considered, as different substances have different abilities to store or release heat energy.
In thermodynamic calculations, cp is often used in conjunction with the mass of a substance and the temperature change to calculate the amount of heat transferred or the change in internal energy. It is also used in the formulation of equations that describe the behavior of substances during various thermodynamic processes, such as the calculation of enthalpy changes.
It’s worth noting that the specific heat capacity at constant pressure, cp, is different from the specific heat capacity at constant volume, cv. The latter refers to the amount of heat required to raise the temperature of a substance by 1 degree Celsius or 1 Kelvin while keeping the volume constant. The relationship between cp and cv is significant in thermodynamics and is often used to analyze and understand the behavior of substances under different conditions.
What is k in thermodynamics?
In thermodynamics, the symbol “k” is often used to represent Boltzmann’s constant. Boltzmann’s constant, denoted as “k” or sometimes as “k_B,” is a fundamental constant in physics that relates the average kinetic energy of particles in a system to its temperature.
Boltzmann’s constant is named after Ludwig Boltzmann, an Austrian physicist who made significant contributions to statistical mechanics. It is defined as:
k = R/N_A
where:
- R is the gas constant, which relates the energy scale of a system to its temperature (approximately 8.314 J/(mol·K) or 1.987 cal/(mol·K)),
- N_A is Avogadro’s number, representing the number of particles (atoms or molecules) in one mole of a substance (approximately 6.022 × 10^23 mol^−1).
The value of Boltzmann’s constant is approximately 1.381 × 10^−23 J/K or 8.617 × 10^−5 eV/K. It is often used in equations and formulas in thermodynamics, statistical mechanics, and quantum mechanics to relate the macroscopic behavior of systems to the microscopic properties of their constituent particles.
What is u in thermodynamics?
In thermodynamics, the symbol “U” typically represents the internal energy of a system. Internal energy refers to the sum of all the microscopic forms of energy in a system, such as the kinetic energy of particles and the potential energy associated with their interactions.
The internal energy, denoted as “U,” is an extensive property, meaning it depends on the amount of substance present in the system. It is also a state function, which implies that its value only depends on the current state of the system and not on how the system reached that state.
The internal energy of a system can change through various processes, including heat transfer (Q) and work done (W) on or by the system. The first law of thermodynamics, often expressed as ΔU = Q – W, relates the change in internal energy (ΔU) to the heat added to or removed from the system (Q) and the work done on or by the system (W).
The internal energy can also be related to other thermodynamic properties, such as temperature (T) and entropy (S), through specific equations and relationships, depending on the specific system and conditions being considered.
What is property of a system in thermodynamics?
In thermodynamics, a property of a system refers to a characteristic or attribute that describes the state or behavior of the system. These properties help us understand and analyze the thermodynamic processes and phenomena occurring in the system. Properties can be classified into two main types: intensive properties and extensive properties.
- Intensive Properties: Intensive properties are independent of the size or extent of the system. They are the same throughout the system regardless of its size. Examples of intensive properties include temperature (T), pressure (P), density (ρ), specific heat capacity (c), and composition.
- Extensive Properties: Extensive properties depend on the size or extent of the system. They are additive and proportional to the amount or quantity of matter in the system. Examples of extensive properties include mass (m), volume (V), total energy (U), enthalpy (H), and entropy (S).
It’s important to note that properties can be further classified into different categories based on their nature. For example, there are thermodynamic properties (such as temperature and pressure) that define the state of a system. There are also transport properties (such as viscosity and thermal conductivity) that describe how a system interacts with its surroundings in terms of the transfer of mass, momentum, or energy.
Properties in thermodynamics can be measured or calculated using various experimental techniques or theoretical models. They play a crucial role in formulating thermodynamic equations, equations of state, and understanding the behavior and transformations of substances and systems under different conditions.
Which physical law underlies the first law of thermodynamics?
The first law of thermodynamics is based on the principle of conservation of energy, which is a fundamental concept in physics. The first law states that energy cannot be created or destroyed, but it can be transferred or converted from one form to another.
The underlying physical law that supports the first law of thermodynamics is the law of conservation of energy, also known as the principle of energy conservation. This principle states that the total amount of energy in an isolated system remains constant over time.
The first law of thermodynamics specifically applies the principle of energy conservation to thermodynamic systems, considering the transfer and conversion of energy as heat (Q) and work (W) between the system and its surroundings. It states that the change in the internal energy (ΔU) of a system is equal to the heat added to the system minus the work done by the system:
ΔU = Q – W
This equation demonstrates how energy is conserved in thermodynamic processes, as any increase or decrease in the internal energy of the system is accounted for by the heat entering or leaving the system and the work done on or by the system.
In summary, the first law of thermodynamics is an application of the broader principle of conservation of energy to thermodynamic systems, providing a framework to analyze and quantify energy transfers and conversions within these systems.
How to interpolate thermodynamics?
Interpolation in thermodynamics involves estimating the value of a thermodynamic property or variable between two known data points. This can be useful when you have a set of discrete data points and need to estimate values at intermediate conditions. Here are a few common methods used for interpolation in thermodynamics:
- Linear Interpolation: Linear interpolation assumes a linear relationship between two known data points. It is the simplest form of interpolation and is appropriate when the data exhibits a relatively smooth and linear behavior. To interpolate between two data points (x1, y1) and (x2, y2) for a given value x, you can use the following formula:y = y1 + (x – x1) * (y2 – y1) / (x2 – x1)
- Polynomial Interpolation: Polynomial interpolation fits a polynomial curve to the given data points. This method provides a more accurate representation of the data but can be susceptible to oscillations and inaccuracies when the data is irregular. Polynomial interpolation can be performed using techniques like Lagrange interpolation or Newton interpolation.
- Cubic Spline Interpolation: Cubic spline interpolation is a commonly used method for smooth interpolation of data. It fits a piecewise cubic polynomial to the data, ensuring continuous first and second derivatives at the data points. This method provides a good balance between accuracy and smoothness. There are various algorithms available for cubic spline interpolation, such as the natural spline, clamped spline, or not-a-knot spline.
When performing interpolation in thermodynamics, it’s essential to ensure that the interpolation method you choose is appropriate for the behavior of the thermodynamic property or variable you are working with. Additionally, it is important to be cautious when extrapolating beyond the range of the given data points, as the accuracy of the interpolation may decrease significantly.
It is often beneficial to use established thermodynamic tables, equations of state, or software tools specifically designed for thermodynamic calculations, as they provide accurate and reliable interpolation methods tailored to thermodynamic systems.
What is combustion in thermodynamics?
Combustion is a chemical reaction that occurs between a fuel and an oxidizer, typically involving the release of heat and the production of combustion products. In the context of thermodynamics, combustion is of great importance as it involves the conversion of chemical energy into thermal energy.
During combustion, the fuel reacts with the oxidizer, which is usually oxygen from the surrounding air. The reaction releases energy in the form of heat and light, and it often manifests as a rapid and visible flame. The combustion process is exothermic, meaning it releases energy to the surroundings.
From a thermodynamic perspective, combustion is analyzed in terms of the First Law of Thermodynamics, which states that energy is conserved in a closed system. In the case of combustion, the energy released from the chemical reaction is transferred to the surroundings as heat (Q) and work (W). The specific details of the energy transfer depend on the specific conditions and constraints of the combustion system.
Combustion processes are characterized by various thermodynamic properties, such as enthalpy, temperature, pressure, and heat release. These properties play a crucial role in the analysis and design of combustion systems, such as engines, furnaces, and power plants.
Thermodynamics helps in understanding the efficiency and performance of combustion systems, as well as in determining parameters like heat transfer, energy conversion, and pollutant formation. It provides a framework to quantify and optimize the energy conversion process involved in combustion, allowing for improved design, control, and efficiency of combustion systems.
When is work positive or negative thermodynamics?
In thermodynamics, the sign convention for work depends on the direction of energy transfer. The sign convention is often defined based on the system and its surroundings.
- Positive Work: Work is considered positive when energy is transferred from the surroundings to the system. This occurs when work is done on the system by an external agent, compressing or pushing the system. For example:
- When a gas is compressed, work is done on the gas, and the work is positive.
- When an external force pushes a piston, resulting in the compression of a gas, the work done on the gas is positive.
- Negative Work: Work is considered negative when energy is transferred from the system to the surroundings. This occurs when the system does work on its surroundings. For example:
- When a gas expands and pushes against the surroundings, the work done by the gas is negative.
- When a gas performs work on a piston, moving it outward, the work done by the gas is negative.
The sign convention for work in thermodynamics is based on the energy flow and the perspective of energy transfer. It’s important to note that the sign convention may vary depending on the context and the specific thermodynamic equation being used. Therefore, it’s crucial to carefully define the system and surroundings, as well as to follow the sign convention consistently to ensure accurate calculations and analysis.
FAQ
How many laws of thermodynamics are there?
There are four laws of thermodynamics, which form the foundation of the field and provide fundamental principles governing the behavior of energy and its interactions with matter. These laws are sequentially numbered and build upon each other:
1. Zeroth Law of Thermodynamics: The zeroth law establishes the concept of thermal equilibrium and the transitive property of temperature. It states that if two systems are separately in thermal equilibrium with a third system, they are also in thermal equilibrium with each other. This law enables the definition and measurement of temperature, which is essential for subsequent laws.
2. First Law of Thermodynamics: The first law, also known as the law of energy conservation, states that energy cannot be created or destroyed in an isolated system. It emphasizes that energy is conserved and can be transferred or converted between different forms, such as heat and work. The first law is often expressed as ΔU = Q – W, where ΔU is the change in internal energy of the system, Q is the heat added to the system, and W is the work done by the system.
3. Second Law of Thermodynamics: The second law introduces the concept of entropy and the direction of natural processes. It states that in a closed system, entropy tends to increase or remain constant over time, but it never decreases spontaneously. The second law leads to various important concepts, such as the Carnot cycle, heat engines, and the definition of absolute temperature scales.
4. Third Law of Thermodynamics: The third law addresses the behavior of systems as they approach absolute zero temperature (0 Kelvin or -273.15 degrees Celsius). It states that as the temperature approaches absolute zero, the entropy of a pure, perfect crystalline substance approaches zero. This law helps define the absolute reference point for entropy calculations and establishes the unattainability of reaching absolute zero temperature.
These four laws collectively provide a comprehensive framework for understanding and analyzing energy, heat, work, and the behavior of systems in thermodynamics. They are essential for studying and applying thermodynamic principles to various fields, including physics, chemistry, engineering, and materials science.
is fermentation thermodynamically favorable?
Fermentation is a metabolic process that involves the conversion of organic substances, such as carbohydrates, into simpler compounds, typically in the absence of oxygen. Examples of fermentation include the conversion of glucose to ethanol in alcoholic fermentation or the conversion of glucose to lactic acid in lactic acid fermentation.
From a thermodynamic perspective, whether fermentation is thermodynamically favorable or not depends on the specific reaction and the conditions under which it occurs. Thermodynamically favorable reactions release energy and proceed spontaneously, whereas thermodynamically unfavorable reactions require an input of energy to proceed.
In the case of fermentation, many reactions involved are thermodynamically favorable under appropriate conditions. These conditions often include the absence of oxygen (anaerobic conditions) and the presence of specific enzymes or microorganisms that facilitate the fermentation process.
During fermentation, the breakdown of organic substances results in the production of simpler compounds, such as ethanol or lactic acid, along with the release of energy in the form of ATP (adenosine triphosphate). The specific thermodynamic favorability of the fermentation process depends on the difference in free energy (ΔG) between the reactants and products.
For example, in alcoholic fermentation where glucose is converted to ethanol and carbon dioxide, the overall reaction can be represented as:
Glucose → Ethanol + Carbon Dioxide
This reaction is thermodynamically favorable, meaning it releases energy, allowing the process to occur spontaneously under suitable conditions.
However, it’s important to note that the efficiency and extent of fermentation can be influenced by factors such as temperature, pH, substrate concentration, and the specific microorganisms involved. In some cases, the conditions may not be favorable for complete fermentation, resulting in the production of byproducts or incomplete conversion of the substrate.
Overall, fermentation can be thermodynamically favorable under the appropriate conditions and provides an energy-yielding pathway for the breakdown of organic substances in the absence of oxygen.
Refrences
- Davidovits, Paul (2013). Physics in Biology and Medicine || Thermodynamics. , (), 127–135. doi:10.1016/B978-0-12-386513-7.00010-2
- https://www.chadsprep.com/chads-general-chemistry-videos/3-laws-of-thermodynamics-definition/
- https://www2.estrellamountain.edu/faculty/farabee/biobk/biobookener1.html#Thermodynamics
- https://web.mit.edu/16.unified/www/FALL/thermodynamics/
- https://www.cliffsnotes.com/study-guides/chemistry/chemistry/thermodynamics/introduction-to-thermodynamics
- https://ncert.nic.in/ncerts/l/kech106.pdf
- https://www.grc.nasa.gov/www/k-12/airplane/thermo.html
- https://www.livescience.com/50776-thermodynamics.html
- https://byjus.com/physics/thermodynamics/
- https://studiousguy.com/laws-of-thermodynamics/
- https://byjus.com/physics/thermodynamics/