The early detection of diseases is crucial for effective treatment, and molecular diagnostics serves as a pivotal tool in this regard. This term encompasses a range of diagnostic techniques that assess an individual’s health at a molecular level. Specifically, molecular diagnostics focuses on the identification and quantification of genetic sequences—often referred to as biomarkers—found in deoxyribonucleic acid (DNA), ribonucleic acid (RNA), or the proteins they produce.
One of the key functions of molecular diagnostics is its ability to determine a person’s predisposition to specific diseases or confirm the presence of an existing condition. Moreover, it aids in evaluating the effectiveness of particular treatment options tailored to individual genetic profiles. This personalized approach marks a significant advancement over traditional methods of disease identification, which primarily relied on microscopy and histopathology.
Historically, clinicians diagnosed conditions like cancer through the observation of cellular morphology under a microscope. This approach, while foundational, is fraught with limitations. Factors such as human error in tissue processing and challenges in accurately identifying histological sections can lead to misdiagnoses. In contrast, molecular diagnostics leverages genetic information to provide a more reliable basis for disease identification, thus enhancing the accuracy of diagnoses.
Furthermore, molecular diagnostics can facilitate the early detection of genetic disorders, enabling timely intervention. Techniques such as polymerase chain reaction (PCR) and next-generation sequencing (NGS) allow for the rapid and precise analysis of genetic material. These methodologies have revolutionized the field by enabling healthcare providers to detect mutations and variations that may contribute to disease susceptibility.
In addition to genetic testing, molecular diagnostics also encompasses the analysis of RNA and proteins, offering insights into gene expression and protein function. This multifaceted approach allows clinicians to understand the pathophysiology of diseases at a deeper level, ultimately leading to improved treatment strategies.
Therefore, the integration of molecular diagnostics into clinical practice has transformed the landscape of disease management. By providing a clearer picture of genetic predispositions and treatment responses, this field not only enhances diagnostic accuracy but also promotes the development of personalized medicine. In conclusion, molecular diagnostics represents a vital advancement in the ongoing quest for precise and effective healthcare solutions, emphasizing the critical role of genetic analysis in the diagnosis and treatment of genetic diseases.
Molecular Diagnostic Techniques
Below are several prominent techniques utilized in molecular diagnostics:
- DNA Immobilization: Techniques such as Southern blotting, dot blotting, in situ hybridization (ISH), and Fluorescent in situ hybridization (FISH) involve the use of labeled DNA or RNA strands. These strands hybridize with complementary target sequences, enabling the identification and quantification of specific genetic material in various sample types, including blood, tissue, and saliva.
- Polymerase Chain Reaction (PCR): This method is a cornerstone of molecular diagnostics. PCR exponentially amplifies specific DNA or RNA sequences present in a sample. By producing a substantial number of copies, PCR makes it possible to detect and quantify minute amounts of genetic material, facilitating the diagnosis of diseases at an early stage.
- Restriction Endonuclease Digestion: This technique involves the use of restriction enzymes to cut DNA at specific sequences. It is instrumental in identifying restriction fragment length polymorphisms (RFLPs), which are variations linked to particular disease genes. This can help in understanding genetic predispositions and variations associated with certain conditions.
- Microarrays: Microarray technology allows for the simultaneous measurement of the expression levels of thousands of genes. Additionally, it can detect single nucleotide polymorphisms (SNPs) and other genomic regions. This broad-spectrum analysis provides insights into gene expression profiles and genetic variations, offering a comprehensive view of a patient’s genetic landscape.
- DNA Sequencing: This technique is crucial for mapping the precise sequence of nucleotides in a DNA strand. Advanced methods, such as next-generation sequencing (NGS), enable the rapid sequencing of entire genomes. This high-throughput capability allows for the identification of genetic mutations and variations that may be implicated in specific diseases.
- Next-Generation Sequencing (NGS): NGS enhances traditional sequencing methods by allowing for the simultaneous sequencing of millions of fragments. This technique is particularly valuable for whole-exome and whole-genome sequencing, providing extensive data for research and clinical diagnostics.
- Quantitative PCR (qPCR): Unlike conventional PCR, qPCR quantifies the amount of DNA in real-time during the amplification process. This technique is especially useful for monitoring viral loads in infectious diseases and assessing gene expression levels in various conditions.
- Droplet Digital PCR (ddPCR): This highly sensitive technique partitions a sample into thousands of droplets, allowing for absolute quantification of nucleic acids. ddPCR is particularly useful for detecting rare mutations and analyzing genetic variants in heterogeneous samples.
Cystic Fibrosis or mucoviscidosis
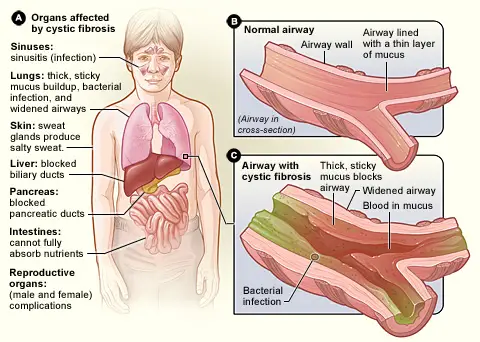
Symptoms of Cystic Fibrosis or mucoviscidosis
Below are the key symptoms associated with cystic fibrosis:
- Respiratory Symptoms:
- Chronic Cough: Patients often experience a persistent cough that may produce thick mucus, leading to difficulties in breathing.
- Frequent Lung Infections: The inability to clear mucus increases the risk of bacterial infections, resulting in recurrent pneumonia or bronchitis.
- Wheezing: Airway obstruction due to thick mucus can cause wheezing, characterized by a whistling sound during breathing.
- Shortness of Breath: As lung function declines, patients may struggle with shortness of breath during routine activities.
- Digestive Symptoms:
- Poor Growth and Weight Gain: Despite normal or increased appetite, malabsorption of nutrients due to pancreatic insufficiency can lead to poor growth in children.
- Frequent, Greasy Stools: Malabsorption results in steatorrhea, which manifests as bulky, oily stools that may float.
- Gastrointestinal Pseudo-obstruction: Thickened mucus can block the intestines, causing abdominal pain and distension, resembling a bowel obstruction.
- Salty Skin:
- Individuals with cystic fibrosis often have sweat that is saltier than normal. This occurs due to impaired chloride ion transport, which can be detected during a sweat test.
- Reproductive Symptoms:
- Male Infertility: The mucus can obstruct the vas deferens, which can lead to infertility in males.
- Menstrual Irregularities: Females may experience irregular menstrual cycles, which can complicate reproductive health.
- Pancreatic Dysfunction:
- The thick mucus can block pancreatic ducts, leading to insufficient enzyme secretion necessary for digestion. This results in digestive problems and nutrient deficiencies.
- Liver Involvement:
- In some patients, the bile ducts may become obstructed due to mucus buildup, potentially leading to liver disease over time.
- Increased Risk of Diabetes:
- Cystic fibrosis-related diabetes is a common complication resulting from damage to the pancreas, affecting insulin production.
- Sinus Issues:
- Patients may suffer from chronic sinusitis due to mucus accumulation in the sinuses, leading to inflammation and infection.
Pathogenesis of Cystic Fibrosis or mucoviscidosis
Cystic fibrosis (CF) is a complex genetic disorder primarily caused by mutations in the CFTR (cystic fibrosis transmembrane conductance regulator) gene, located on chromosome 7. The pathogenesis of cystic fibrosis involves a series of molecular and cellular mechanisms that disrupt normal physiological functions across various organ systems. The following points elucidate the underlying processes contributing to the disease:
- Genetic Mutations:
- CFTR gene mutations can affect protein structure and function. Over 1,900 mutations have been identified, with the most common being ΔF508, which leads to improper protein folding and degradation.
- The CFTR gene spans approximately 250,000 base pairs and contains 27 exons, producing a 6,129 base pair mRNA transcript that encodes a 1,480 amino acid protein.
- Defective CFTR Protein:
- The CFTR protein functions as a chloride ion channel and is critical for maintaining the balance of salt and water in epithelial tissues. Mutations disrupt its ability to transport chloride ions across cell membranes.
- This impairment results in reduced hydration of airway surface liquid, leading to the production of thick, viscous mucus.
- Altered Ion Transport:
- In healthy cells, CFTR regulates the transport of chloride ions and influences sodium absorption. In CF, the defective CFTR leads to excessive sodium absorption and insufficient chloride transport.
- Consequently, the osmotic balance is disrupted, leading to dehydration of mucus, which becomes thick and sticky.
- Mucus Accumulation:
- The impaired clearance of mucus from the airways results in obstruction and chronic inflammation. This environment fosters bacterial colonization, contributing to recurrent respiratory infections.
- The buildup of thick mucus can also block pancreatic ducts, leading to malabsorption of nutrients.
- Multisystem Involvement:
- CF is characterized by its effects on multiple organ systems, primarily the lungs, pancreas, liver, intestines, and reproductive tract. The widespread expression of CFTR in these tissues accounts for the diverse symptoms associated with the disorder.
- For instance, in the reproductive system, mucus obstruction can lead to congenital bilateral absence of the vas deferens in males, resulting in infertility.
- Pathological Consequences:
- Chronic lung infections and inflammation can result in progressive lung damage, leading to respiratory failure, which is a common cause of mortality in CF patients.
- Long-term complications include pancreatic insufficiency, diabetes, liver disease, and gastrointestinal issues due to obstructed bile ducts.
- Potential for Treatment:
- Understanding the molecular pathogenesis of cystic fibrosis has led to targeted therapies, such as CFTR modulators that aim to correct the function of the defective protein.
- These advancements highlight the importance of molecular diagnostics in developing personalized treatment approaches.
Structure of CFTR
The following points outline the key structural components of CFTR:
- Gene Characteristics:
- The CFTR gene spans approximately 189 kilobases and consists of 27 exons.
- The protein product is a glycoprotein composed of 1,480 amino acids, highlighting its complexity.
- Domain Organization:
- CFTR is organized into five distinct functional domains, each playing a critical role in its overall function:
- Membrane-Spanning Domains (MSD1 and MSD2):
- These two domains are responsible for forming the chloride ion channel, crucial for the transport of chloride ions across cell membranes.
- Each membrane-spanning domain comprises six alpha-helical segments, creating a channel that allows ion passage.
- Nucleotide-Binding Domains (NBD1 and NBD2):
- NBD1 and NBD2 bind and hydrolyze ATP, providing the energy necessary for channel activation and regulation.
- NBD1 is particularly significant, as mutations in this domain, such as ΔF508, are commonly associated with CF.
- Regulatory Domain (R Domain):
- The R domain is essential for the proper regulation of CFTR’s activity.
- It connects NBD1 to MSD2 and contains phosphorylation sites that modulate channel opening in response to cellular signals.
- Membrane-Spanning Domains (MSD1 and MSD2):
- CFTR is organized into five distinct functional domains, each playing a critical role in its overall function:
- Transmembrane Domains:
- CFTR includes twelve transmembrane domains (M1 to M12), which are crucial for its structural integrity and function.
- These domains consist of typical alpha-helical structures that line the ion channel, influencing its permeability and regulation.
- Functional Significance of Structure:
- The organization of CFTR allows for precise regulation of chloride and bicarbonate ion transport, which is vital for maintaining epithelial hydration and mucus clearance.
- Mutations affecting the structural integrity of CFTR, particularly in the NBDs and MSDs, lead to the dysfunctional ion transport characteristic of cystic fibrosis.
- Pathological Implications:
- The ΔF508 mutation exemplifies how alterations in CFTR structure can prevent proper folding and function, resulting in the degradation of the protein before it reaches the cell membrane.
- This disruption contributes to the clinical manifestations of CF, including thickened mucus and impaired organ function.
Function of CFTR
The cystic fibrosis transmembrane conductance regulator (CFTR) plays a crucial role in maintaining ion and fluid balance in various epithelial tissues throughout the body. Understanding its functions is essential for comprehending the pathophysiology of cystic fibrosis (CF). The following points summarize the primary functions of CFTR:
- Ion Channel Activity:
- CFTR operates primarily as a chloride ion channel located in the membranes of epithelial cells.
- It facilitates the movement of negatively charged chloride ions in and out of cells, which is critical for maintaining the electrochemical gradient.
- Cyclic-AMP Dependency:
- The activity of CFTR is regulated by cyclic adenosine monophosphate (cAMP), a signaling molecule.
- Increased levels of cAMP activate protein kinase A (PKA), which then phosphorylates the regulatory (R) domain of the CFTR protein.
- This phosphorylation is essential for channel activation, allowing CFTR to open and function properly.
- Regulation of Water Movement:
- The transport of chloride ions by CFTR influences the movement of water via osmosis.
- When chloride ions are transported into the airway lumen, water follows, leading to the production of thin, hydrated mucus essential for respiratory health.
- Mucus Clearance:
- The proper functioning of CFTR ensures that mucus remains fluid and can be easily cleared from the airways by ciliated epithelial cells.
- This process is vital for trapping and removing pathogens and debris from the respiratory tract, thus maintaining lung health.
- Sodium Ion Balance:
- CFTR also plays a role in regulating sodium ion balance. By facilitating chloride transport, it indirectly influences sodium absorption, which is crucial for maintaining osmotic balance within epithelial tissues.
- Impact of CF Mutations:
- In cystic fibrosis patients, mutations in the CFTR gene disrupt its function, leading to impaired chloride and water transport.
- This dysfunction results in the production of thick, sticky mucus that obstructs airways and can lead to recurrent lung infections and respiratory complications.
- Sweat Gland Function:
- CFTR’s role extends to sweat glands, where it regulates chloride and sodium absorption.
- Mutations in CFTR impair this function, leading to excessively salty sweat, a hallmark of cystic fibrosis.
Common Mutations leading to Cystic Fibrosis
Cystic fibrosis (CF) arises from mutations in the CFTR gene, which encodes the cystic fibrosis transmembrane conductance regulator protein. Various mutations lead to distinct phenotypes and disease severity. Understanding these mutations is critical for comprehending the disease mechanism and exploring potential treatments. Below are the key points regarding common mutations associated with cystic fibrosis:
- ΔF508 Mutation:
- This deletion involves three nucleotides in the DNA sequence, leading to the loss of the amino acid phenylalanine at position 508 of the CFTR protein.
- It is the most prevalent mutation, found in approximately 90% of CF patients.
- Due to this mutation, the first nucleotide-binding domain (NBD1) of CFTR fails to form correctly, resulting in a misfolded protein that is targeted for degradation by cellular quality-control mechanisms. This absence of functional CFTR prevents proper ion and water transport.
- Class I Mutations:
- These mutations disrupt the biosynthesis of CFTR by introducing premature stop codons or causing abnormal mRNA splicing.
- An example is the W1282X mutation, where the sequence is truncated at position 128, leading to the absence of the tryptophan amino acid.
- Class II Mutations:
- This category includes mutations that affect the maturation and folding of the CFTR protein.
- The ΔF508 mutation also falls under this class as it leads to improper folding and subsequent degradation of the protein.
- Class III Mutations:
- These mutations alter the regulation of the CFTR channel, affecting its activation.
- An example is the G551D missense mutation, where glycine is replaced by aspartate at position 551, disrupting ATP binding and hydrolysis necessary for channel activation.
- Class IV Mutations:
- Mutations in this class impact the conduction of chloride ions through the CFTR channel.
- The R117H missense mutation serves as an example, where histidine replaces arginine at position 117, resulting in reduced channel activity but not complete loss.
- Class V Mutations:
- These mutations reduce the overall quantity of functional CFTR protein at the cell membrane, rather than altering its function.
- An example is the splice-site mutation 3120+1G>A in intron 16, where the normal splice process is disrupted, affecting the production of the CFTR protein.
- Class VI Mutations:
- This class of mutations influences the regulation of other ion channels, such as sodium channels, indirectly affecting ion transport and fluid secretion.
Possible Methods used in CFTR testing
Identifying mutations in the CFTR gene is essential for diagnosing cystic fibrosis (CF). Various methods are employed, broadly categorized into direct or targeted methods and indirect or scanning methods. Each method plays a significant role in detecting specific genetic alterations associated with the disease. Below are the primary techniques utilized in CFTR testing:
- Targeted Methods:
- These techniques focus on detecting specific known mutations, such as the ΔF508 deletion.
- This method employs sequence-specific oligonucleotide (SSO) probes designed to bind to either the normal or mutated CFTR gene sequences.
- SSO1 is complementary to the normal sequence, while SSO2 targets the mutated sequence.
- After amplifying CFTR DNA using PCR, samples are applied to a membrane as dot blots.
- Each blot is hybridized with SSO probes, allowing for differentiation between genotypes:
- Individuals homozygous for the ΔF508 mutation will hybridize only to SSO2.
- Those homozygous for the normal sequence will hybridize to SSO1.
- Heterozygous individuals will bind to both probes, indicating one normal and one mutated allele.
- Primers are designed to amplify the CFTR gene, specifically distinguishing between normal and mutant alleles.
- The PCR process generates two distinct products: a 154 bp fragment from normal alleles and a 151 bp fragment from mutated alleles.
- Heterozygous carriers will show both band sizes on a gel, indicating the presence of one normal and one mutated gene.
- RFLP utilizes restriction enzymes to cut DNA at specific sequences.
- Mutations like ΔF508 can alter restriction sites, changing the pattern of DNA fragments produced.
- This method allows for the identification of genetic variations by comparing the digestion patterns of normal and mutated sequences.
- Indirect or Scanning Methods:
- These techniques screen for any deviations from the standard CFTR gene sequence rather than targeting specific mutations.
- While the detailed descriptions of these methods are outside the current scope, they generally involve broader genomic analysis to identify potential mutations that may not be well characterized.
Huntington’s Disease
Huntington’s disease, also known as Huntington’s chorea, is a progressive and fatal neurodegenerative disorder characterized by autosomal dominant inheritance. This means that a single copy of the mutated gene is sufficient to cause the disease, making both males and females equally susceptible. The presence of the Huntington gene on an autosome ensures that the disease is not sex-linked, allowing for a 50% chance of inheritance for each child of an affected individual. Below is a detailed exploration of Huntington’s disease, its inheritance patterns, symptoms, and forms.
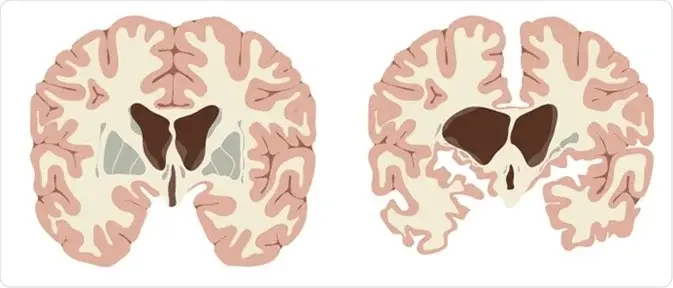
- Genetic Basis:
- Huntington’s disease is caused by a mutation in the HTT gene located on chromosome 4.
- The mutation involves an expansion of CAG repeats, leading to an abnormal protein that disrupts neuronal function.
- Affected individuals possess one normal allele (non-HD) and one mutant allele (HD), with each child having a 50% chance of inheriting the mutant allele.
- Autosomal Dominant Inheritance:
- Only one copy of the mutated gene is necessary for the expression of the disease phenotype.
- Each pregnancy from an affected individual has a 50% chance of resulting in an offspring with the disease allele.
- Typically, all affected individuals have at least one parent with the disease unless a new mutation has occurred.
- This mode of inheritance is referred to as “vertical inheritance” because it appears in multiple generations.
- Male-to-male transmission is possible, and the incidence of the disease is similar between genders.
- Clinical Manifestations:
- Symptoms of Huntington’s disease can be classified into three main categories, often termed the “clinical triad”:
- Movement Abnormalities (Chorea):
- Characterized by involuntary, irregular movements that can affect various muscle groups.
- Patients may exhibit difficulty in coordinating movements, leading to problems with walking, speech, and swallowing.
- Emotional Disturbances:
- Individuals may experience mood swings, depression, anxiety, and irritability.
- Emotional disturbances can significantly affect social interactions and quality of life.
- Cognitive Impairment:
- Cognitive decline can manifest as difficulties with planning, organizing, and executing tasks.
- Memory issues and impaired judgment are also common, leading to progressive dementia.
- Movement Abnormalities (Chorea):
- Symptoms of Huntington’s disease can be classified into three main categories, often termed the “clinical triad”:
- Forms of Huntington’s Disease:
- Early-Onset Huntington Disease:
- This rare form begins in childhood or adolescence, presenting with symptoms earlier than the typical adult onset.
- Adult-Onset Huntington Disease:
- The most common form, typically diagnosed in individuals during their 30s or 40s.
- Symptoms gradually worsen, often leading to severe impairment over time.
- Early-Onset Huntington Disease:
Symptoms and Causes of Huntington’s Disease
Huntington’s disease (HD) is a neurodegenerative disorder marked by a progressive decline in cognitive, motor, and psychiatric functions. The underlying pathology of HD primarily involves the selective loss of neurons within the striatum, notably affecting areas such as the caudate nucleus and putamen. Understanding the symptoms and causes of Huntington’s disease provides insight into its debilitating nature and its impact on affected individuals.
- Symptoms of Huntington’s Disease:
- The clinical manifestations can be categorized into three main areas:
- Movement Disorders:
- Chorea: This is characterized by involuntary, irregular movements that can affect the entire body, leading to difficulties in voluntary control.
- Dystonia: Sustained muscle contractions may result in abnormal postures.
- Bradykinesia: Slowness of movement becomes apparent as the disease progresses.
- Cognitive Impairments:
- Declines in executive functions, including planning and organizing tasks.
- Impaired memory and difficulty in concentrating.
- Progression toward dementia, particularly in the later stages of the disease.
- Psychiatric Disturbances:
- Mood swings, depression, and anxiety are common emotional challenges faced by individuals.
- Social withdrawal and changes in personality can also occur.
- Movement Disorders:
- The clinical manifestations can be categorized into three main areas:
- Causes of Huntington’s Disease:
- The primary cause of Huntington’s disease is a genetic mutation in the HTT gene located on chromosome 4. This gene encodes the huntingtin protein, which is crucial for neuronal function.
- The mutation involves an expansion of CAG repeats, leading to the production of an abnormally long huntingtin protein. This abnormal protein disrupts cellular function and promotes neuronal death.
- The disease follows an autosomal dominant inheritance pattern, which means that only one mutated copy of the gene is necessary for the disease to manifest. Each child of an affected individual has a 50% chance of inheriting the mutated allele.
- Neuropathological Characteristics:
- The hallmark of Huntington’s disease pathology is the atrophy of specific brain regions, particularly the striatum. This atrophy results in a significant loss of medium spiny neurons, which are critical for movement control and coordination.
- As the disease advances, the overall brain weight may decrease by 25-30%, with substantial degeneration also observed in the globus pallidus and subthalamic nucleus.
- Widespread neuronal loss in the cerebral cortex contributes to cognitive decline and dementia. This neuronal loss disrupts communication within neural networks, leading to the diverse array of symptoms associated with Huntington’s disease.
Structure of Huntingtin gene
The huntingtin gene (HTT) is pivotal in understanding Huntington’s disease, as it encodes the huntingtin protein, which plays various roles in cellular functions. Identified in 1989, the HTT gene is located on the short arm of chromosome 4 at position 16.3. Its structure and function are critical to the pathophysiology of Huntington’s disease.
- Gene Structure:
- The HTT gene consists of 67 exons, encompassing approximately 180 kilobases (kb) of DNA. This extensive structure contributes to the complex nature of the huntingtin protein.
- The gene features a repeating region of CAG trinucleotide repeats, which can vary in length. This region encodes a sequence of glutamine residues, which are crucial for the protein’s properties. Typically, normal individuals have fewer than 36 repeats, while individuals with Huntington’s disease often have 40 or more, leading to an expanded polyglutamine tract.
- Protein Characteristics:
- Huntingtin is classified as a large protein, weighing about 347 kilodaltons (kDa) and comprising 3,144 amino acids. Its size and composition are significant for its various functions within the cell.
- The N-terminal region of the protein is particularly important due to the presence of the glutamine repeat. The expansion of this region in mutant forms of huntingtin leads to the protein’s misfolding and subsequent neurotoxicity.
- Expression and Localization:
- The huntingtin protein is expressed throughout the human body, with notable concentrations in the male testes and neurons of the central nervous system. This widespread expression underscores its fundamental roles in various biological systems.
- Although the precise cellular functions of huntingtin remain partially elucidated, several studies have indicated that it is involved in key processes such as:
- Protein Trafficking: Facilitating the movement of proteins to their designated locations within cells.
- Vesicle Transport: Aiding in the transport of vesicles that carry neurotransmitters and other molecules.
- Cytoskeletal Anchoring: Contributing to the stability and structure of the cytoskeleton.
- Clathrin-Mediated Endocytosis: Playing a role in the internalization of cellular materials.
- Postsynaptic Signaling: Influencing signaling pathways at synapses, thus impacting neuronal communication.
- Transcriptional Regulation: Modulating gene expression by interacting with transcription factors.
- Anti-Apoptotic Functions: Providing protection against programmed cell death in certain cellular contexts.
Mutation that causes Huntingtin’s disease
The mutation that causes Huntington’s disease (HD) is a result of a CAG trinucleotide repeat expansion within the HTT gene. This mutation disrupts the normal function of the huntingtin protein, leading to progressive neurodegeneration. Below is an overview of the specific genetic mutation involved in HD:
- CAG Trinucleotide Repeats:
- In a normal HTT gene, the number of CAG repeats ranges from 10 to 29. However, some individuals may have up to 30-35 repeats, and this is still considered within the normal range.
- CAG repeats are polymorphic, meaning that the number of repeats can vary between individuals without necessarily leading to disease. This variation contributes to the genetic diversity of the HTT gene.
- When the number of CAG repeats exceeds 35, Huntington’s disease is likely to develop. In most cases, individuals with HD have more than 40 CAG repeats, and the severity and age of onset of the disease often correlate with the number of repeats.
- CAG Expansion and Pathogenesis:
- The expansion of the CAG sequence leads to the production of an abnormally long chain of glutamine residues in the huntingtin protein. This expanded polyglutamine tract disrupts the protein’s normal function and triggers toxic effects in neurons, particularly in the striatum and cortex of the brain.
- The mutated huntingtin protein forms aggregates in the brain, which interfere with neuronal function, contributing to the motor, cognitive, and psychiatric symptoms of HD.
- CCG Polymorphism:
- Upstream from the CAG repeat, there is another polymorphic region consisting of CCG repeats, which encode polyproline. The size of the CCG tract can vary from 7 to 12 repeats, but unlike the CAG region, there is no direct correlation between the number of CCG repeats and the manifestation of Huntington’s disease.
- However, research has suggested an interesting population-specific relationship between CAG and CCG repeat numbers. For example, in Caucasian populations, HD cases with expanded CAG repeats are often associated with (CCG)7, while in Mongoloid populations, the association tends to be with (CCG)10. This indicates potential genetic variation linked to ethnic background.
- Threshold for Disease Onset:
- Individuals with 36 to 39 CAG repeats may have a reduced penetrance of Huntington’s disease, meaning that some may develop symptoms later in life, while others may not experience significant symptoms.
- In contrast, individuals with 40 or more CAG repeats will almost certainly develop the disease, with symptoms typically emerging in mid-adulthood. The more repeats present, the earlier the onset of the disease.
- Implications for Heredity:
- The CAG repeat expansion can increase in size when passed from one generation to the next, a phenomenon known as anticipation. This often results in earlier onset and more severe disease in successive generations, especially when inherited from the father.
Molecular Methods for testing Huntington’s Disease
Molecular methods for testing Huntington’s disease (HD) focus on accurately quantifying the number of CAG repeats within the HTT gene. Given the clinical significance of detecting these repeats, several diagnostic techniques have been developed. Below is a detailed overview of the primary molecular methods employed in the diagnosis of Huntington’s disease:
- Polymerase Chain Reaction (PCR) for CAG Repeat Detection:
- The most straightforward approach involves using simple PCR to amplify the CAG repeat region of the HTT gene. This is accomplished by designing two primers that flank the CAG repeats.
- The resulting PCR products are analyzed using polyacrylamide gel electrophoresis, allowing for size estimation of the amplified fragments.
- A secondary primer set is often utilized to amplify adjacent regions, providing confirmation of results.
- Limitations include:
- Large CAG Repeats: If a CAG repeat exceeds approximately 150 repeats, it may be undetectable using standard PCR methods, potentially leading to misinterpretation of results.
- Allele Amplification Issues: Mutations in primer-binding regions can prevent amplification of one allele, resulting in a misleading diagnosis suggesting two identical alleles.
- High GC Content: Loci with high cytosine-guanine (C+G) content can be challenging for conventional PCR techniques, limiting detection efficiency.
- Shadow Bands: Radioactive or silver-stained gels may display additional bands, complicating the identification of the primary allele, especially when bands have similar intensities.
- Inconsistent Sizing: Variability in migration rates can affect size determination, particularly with low yield products.
- Fluorescent PCR Method:
- An enhancement over conventional PCR is the fluorescent PCR technique, which utilizes fluorescently labeled primers to amplify the CAG repeats.
- Detection and analysis are conducted with a fluorescent DNA sequencer (e.g., GeneScanner).
- Advantages of this method include:
- Increased Sensitivity: Fluorescent detection allows for the identification of lower quantities of DNA.
- Quantitative Measurement: This method enables precise quantification of the amplified products.
- Fewer PCR Cycles Required: Reduced cycles lead to cleaner results and simpler band interpretation.
- Visualization of Weak Signals: Weak signals can be detected effectively, facilitating the identification of alleles with high CAG repeat counts.
- Southern Blot Hybridization:
- For cases where CAG repeat numbers exceed 40, Southern blot hybridization is a valuable diagnostic method, particularly for presymptomatic testing in high-risk individuals.
- This technique involves a 360 bp PCR-derived labeled probe, specifically targeting the CAG repeat region, which hybridizes to genomic DNA from the individual being tested.
- This method provides:
- Direct Measurement: Southern blotting offers a direct assessment of CAG repeat counts, which is crucial for accurate diagnosis.
- High Sensitivity and Accuracy: The technique is recognized for its reliability in confirming genotypes, including those classified as “homozygous normal.”
Sickle Cell Anaemia
Sickle cell anemia, also referred to as sickle cell disease, stands as a prominent example of a genetic disorder characterized at the molecular level. It represents the most prevalent inherited blood disorder in the United States, particularly affecting individuals of African descent. This condition primarily impacts hemoglobin, the protein responsible for transporting oxygen throughout the body.
The defining feature of sickle cell disease is the alteration of red blood cells into a characteristic sickle shape. This morphological change results from a specific point mutation in the gene encoding hemoglobin. In this mutation, the normal form of hemoglobin, denoted as HbA, is replaced by the abnormal variant known as HbS. This alteration significantly affects the properties of red blood cells, leading to complications associated with the disease.
Sickle cell disease is inherited in an autosomal recessive manner. This means that an individual must inherit two copies of the sickle cell allele—one from each parent—to manifest the disease. When both parents are carriers of the sickle cell trait, there exists a 25% probability that their offspring will inherit the disease.
It is essential to differentiate between sickle cell trait and sickle cell disease. Individuals with sickle cell trait carry one copy of the HbS mutation but typically do not exhibit any symptoms of the disease. Conversely, those with sickle cell disease express the full spectrum of symptoms associated with the condition, which can include pain crises, anemia, and various complications due to impaired blood flow.
The sickling of red blood cells leads to significant clinical manifestations, including episodes of severe pain, increased risk of infections, and organ damage. Understanding the genetic basis and inheritance pattern of sickle cell anemia is crucial for effective diagnosis, management, and genetic counseling for affected families.
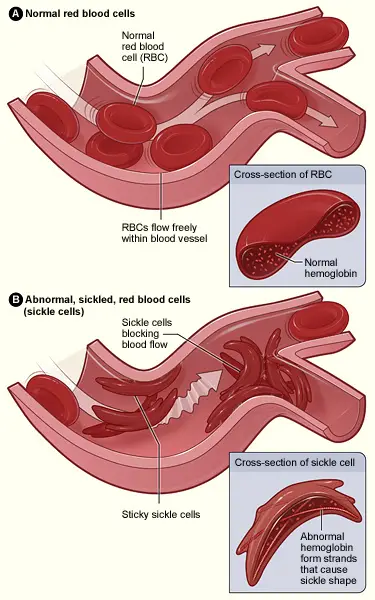
Symptoms and causes of Sickle Cell Disease
Sickle cell disease is characterized by the presence of abnormal hemoglobin, specifically hemoglobin S, which alters the shape of red blood cells. This condition leads to a crescent or sickle-shaped formation of the cells, significantly impacting their function and lifespan. Normal red blood cells typically have a lifespan of about 120 days, while sickle cells only survive for approximately 10 to 20 days. Consequently, the rapid destruction of these cells hampers the bone marrow’s ability to produce sufficient new red blood cells, resulting in a severe reduction of hemoglobin and subsequent anemia.
- Symptoms of Sickle Cell Disease:
- Individuals may experience severe pain, known as vaso-occlusive crises, caused by sickle cells blocking blood vessels.
- Anemia leads to symptoms such as paleness, shortness of breath, fatigue, and delayed growth in children due to inadequate oxygen supply.
- Jaundice may occur, characterized by yellowing of the eyes and skin, reflecting the breakdown of red blood cells.
- Recurrent infections are common, particularly in children, due to splenic damage, which impairs immune function.
- Other complications include blindness and stroke, arising from reduced blood flow and oxygen to vital organs.
- Pulmonary hypertension is a serious complication where high blood pressure affects the blood vessels in the lungs, potentially leading to heart failure.
- Causes of Sickle Cell Disease:
- The root cause of sickle cell disease is a point mutation in the gene that encodes hemoglobin, leading to the production of hemoglobin S.
- This genetic disorder is inherited in an autosomal recessive pattern, meaning that an individual must inherit two copies of the mutated gene—one from each parent—to exhibit symptoms.
- Carriers of the sickle cell trait possess one normal hemoglobin gene and one sickle cell gene, usually without presenting any symptoms but can pass the trait to offspring.
Mutation which causes Sickle cell anemia
Sickle cell anemia is a genetic disorder resulting from a specific mutation in the beta globin gene (HBB), which plays a critical role in hemoglobin function. Understanding this mutation provides insight into how molecular changes can affect both cellular behavior and overall organism health.
- Nature of the Mutation:
- The disease is caused by a point mutation in the HBB gene, leading to the production of an abnormal hemoglobin variant known as hemoglobin S (HbS).
- Specifically, this mutation results in the substitution of valine, a non-polar amino acid, for glutamic acid, which is electrically charged. This seemingly minor alteration has profound effects on the properties of hemoglobin.
- Effects at the Cellular Level:
- Under low oxygen conditions, red blood cells containing HbS undergo a transformation, changing from their typical disc shape to a crescent or sickle shape.
- This sickling of red blood cells can obstruct blood flow, leading to vaso-occlusive crises, characterized by severe pain and potential organ damage.
- Impacts on Organismal Health:
- Although carriers of the sickle cell allele typically do not exhibit full-blown disease, they may experience symptoms such as fatigue and pain, particularly under conditions of physical exertion or at high altitudes.
- Notably, individuals with one copy of the mutant allele (sickle cell trait) possess some advantages, particularly resistance to malaria. The sickle-shaped cells can effectively destroy the malaria parasites, providing a selective survival benefit in malaria-endemic regions.
- Propagation of Effects:
- The mutation’s impact extends beyond molecular biology; it showcases a clear chain of causation where changes at the DNA level influence cellular function, leading to observable physiological outcomes in the organism.
- This example illustrates the dual nature of mutations, where a single nucleotide change can have both deleterious and advantageous effects, highlighting the complexity of evolutionary processes.
Methods used in testing Sickle Cell Anemia
Testing for sickle cell anemia employs a variety of molecular techniques to detect the presence of the mutation responsible for the disorder. These methods ensure accurate diagnosis and enable genetic counseling. Below are the primary testing methodologies used:
- Restriction Fragment Length Polymorphism (RFLP) Analysis:
- The RFLP method identifies variations in DNA sequences by utilizing restriction enzymes that cut DNA at specific recognition sites.
- In sickle cell anemia, the normal HbA allele contains a specific MstII recognition site (CCTGAGG), while the sickle cell HbS allele has a slight alteration (CCTGTGG), due to a single nucleotide substitution.
- When genomic DNA is digested with MstII, the resultant fragments differ depending on the presence of the HbA or HbS alleles. The patterns can be visualized using Southern blotting.
- Southern Blot Hybridization:
- This technique involves digesting total DNA from the individual with MstII, followed by separation using agarose gel electrophoresis.
- The gel is then transferred to a filter, where it is hybridized with a labeled probe specific to the beta-globin gene.
- The resulting band patterns reveal the genotype: two bands for AA homozygotes, one band for SS homozygotes, and three bands for AS heterozygotes. This method allows for the detection of the sickle cell allele as a genetic marker.
- Polymerase Chain Reaction-Oligonucleotide Ligation Assay (PCR-OLA):
- PCR-OLA is a sensitive technique that amplifies the target DNA and utilizes specific oligonucleotide probes designed to hybridize adjacent to the mutation site.
- The ligation of these probes is contingent upon their perfect alignment with the target sequence. If there is a mismatch, the ligation does not occur, allowing differentiation between normal and mutant sequences.
- To visualize successful ligation, a colorimetric reaction is employed. Probes are labeled with distinct markers, and the development of color indicates successful ligation, confirming the presence of the sickle cell mutation.
- Automation of Assays:
- The PCR-OLA technique has been automated to enhance efficiency and accuracy, facilitating large-scale screening for sickle cell disease.
- The use of robotic workstations streamlines the various procedural steps, resulting in rapid and sensitive detection of sickle cell anemia.
- Bienvenu T, Lopez M, Girodon E. Molecular Diagnosis and Genetic Counseling of Cystic Fibrosis and Related Disorders: New Challenges. Genes (Basel). 2020 Jun 4;11(6):619. doi: 10.3390/genes11060619. PMID: 32512765; PMCID: PMC7349214.
- Maddalena A, Bick DP, Schulman JD. Molecular diagnosis of genetic disease. J Reprod Med. 1992 May;37(5):437-44. PMID: 1507192.
- https://www.slideshare.net/slideshow/molecular-diagnosis-of-genetic-disease-ppt-for-students/122615783
- https://www.fujirebio.com/en/insights/genetic-disorders/cystic-fibrosis-diagnosis-and-molecular-testing
- http://www.med.nu.ac.th/pathology/405313/book56/Genetics-2.pdf
- https://edurev.in/p/62464/Lecture-1-Molecular-diagnosis-of-genetic-diseases
- https://renaissance.stonybrookmedicine.edu/sites/default/files/Sickle%20Cell%20Anemia-Molecular%20Dx%20and%20Prenatal%20Counseling.pdf
- https://www.nationwidechildrens.org/conditions/health-library/autosomal-recessive-cystic-fibrosis-sickle-cell-anemia-tay-sachs-disease
- https://redox-college.s3.ap-south-1.amazonaws.com/kmc/2020/Apr/15/0dd6p67pIgMn5nE3cDer.pdf