Confocal microscopy offers some pretty cool perks compared to regular optical microscopes. For starters, it gives you a razor-thin focus depth, cuts out that annoying blurry background glow, and lets you snap crisp, detailed “slices” of thick samples—one after another. This makes it a go-to tool in biomedicine, especially for studying cells and tissues, whether they’re preserved or alive, that have been tagged with fluorescent markers. Think of it like highlighting parts of a complex structure to see exactly where things are happening.
Now, if you’re new to this, the laser scanning confocal microscope (LSCM) is probably the one you’ll bump into first. It’s the workhorse of most biomedical labs today. But here’s the thing: while the LSCM is the default for many researchers, there are niche areas in bioimaging where other designs steal the spotlight. The good news? Most sample prep steps are pretty universal. Whether you’re tweaking settings for a different confocal model, using deconvolution software to sharpen images, or even working with fancy setups like multi-photon imaging, the basics stay the same—just a few adjustments here and there.
What is a Confocal Microscope?
So, what exactly is a confocal microscope? Imagine a microscope that uses laser beams like a precision spotlight, letting scientists peek into different layers of a sample—almost like slicing a cake to see each layer without actually cutting it. That’s the magic of confocal microscopy. It works by scanning samples with a super-focused laser, then picking up the fluorescent glow emitted at specific depths. This tech, called confocal laser scanning microscopy (CLSM), is basically the VIP pass to high-res 3D imaging.
Why does this matter? Well, it’s a superstar in labs worldwide. Biologists use it to spy on cells, doctors study tissues for clues about diseases, and materials scientists even use it to inspect tiny structures in new materials. The big sell? It lets researchers build crystal-clear 3D images by snapping “slices” of a sample layer by layer, then stitching them together with smart software. Oh, and you can tag different parts of a sample with colorful fluorescent markers—like using neon highlighters to track multiple things at once.
A quick history lesson:
- 1955: Marvin Minsky (yep, the AI pioneer!) first dreamed up the idea. His brainwave? Use a laser to scan samples and piece together images from different depths.
- 1970s: Minsky’s team at MIT built the first working version. Their prototype used a clunky helium-neon laser and a photomultiplier tube—basically the Stone Age compared to today’s gear.
- 1980s: Tech caught up. Solid-state lasers and CCD cameras (think early digital cameras) turned confocal scopes from lab curiosities into everyday tools.
- 1990s: Things got wilder with multi-photon imaging—like upgrading from a flip phone to a smartphone for microscopy.
Today, confocal scopes are everywhere in science. They’ve helped crack mysteries about how cells work, how diseases spread, and even how materials behave at the tiniest scales. And they’re still evolving—researchers keep finding new ways to push what these machines can do.
Principle of Confocal Microscope
In wide-field microscopy, light illuminates the entire specimen, capturing signals from all planes (focused and unfocused). This creates cluttered, low-contrast images due to overlapping light from multiple depths.
The confocal microscope solves this by using laser-based point illumination. A specimen stained with fluorochromes is scanned with a tightly focused laser beam (0.25–0.8 µm diameter). Emitted fluorescence passes through a confocal pinhole above the objective lens, which blocks stray light from outside the focal plane.
Two galvanometer mirrors control the laser’s movement: one scans horizontally (X-axis), the other vertically (Y-axis). After each scan, the beam resets rapidly (flyback), skipping data collection during this phase. By capturing sequential optical slices (0.5–1.5 µm thick) along the specimen’s depth (Z-axis), the system assembles a 3D image.
Result: Only in-focus regions appear bright; out-of-focus areas remain dark. This eliminates background noise, enhancing resolution and contrast even in thick samples like tissues or biofilms.
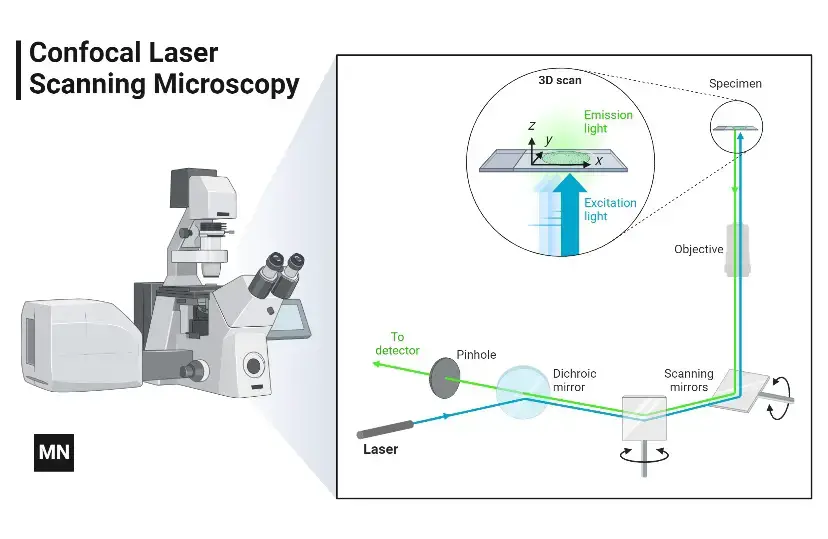
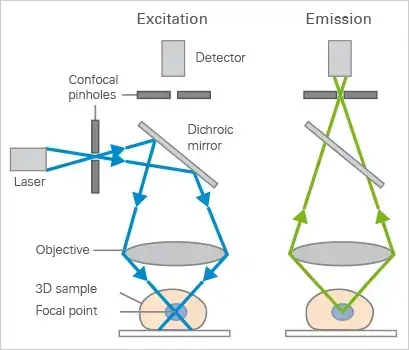
Mechanism of Confocal Microscope – How does a confocal microscope work?
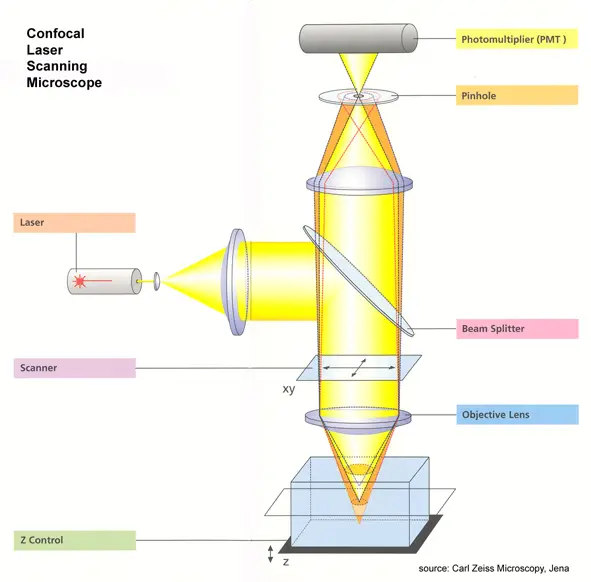
- Laser Light Source
A laser beam (instead of regular light) is used to illuminate the sample. Its intensity is controlled using filters to avoid damaging the sample. - Scanning the Beam
Two fast-moving mirrors tilt the laser beam side-to-side (X-axis) and up-down (Y-axis), creating a precise grid pattern to scan the sample. - Focusing the Light
An objective lens focuses the laser beam into a tiny spot on the fluorescently labeled sample. This excites the dyes, making them glow. - Fluorescent Light Emission
The excited dyes emit light (fluorescence) at a longer wavelength than the laser. This emitted light travels back through the same lens and mirrors. - Removing Unwanted Light
A special mirror directs the fluorescent light away from the laser path. The light then passes through a confocal pinhole, which blocks out-of-focus or scattered light, ensuring only sharp signals reach the detector. - Amplifying Weak Signals
A photomultiplier tube (PMT) detects the faint fluorescent light. It amplifies the signal millions of times without adding noise. - Creating the Image
The PMT converts light into electrical signals. A computer translates these signals into pixels, building a sharp 2D image of the scanned area. - 3D Imaging
By scanning the sample layer-by-layer at different depths, the microscope compiles multiple 2D images to reconstruct a 3D model.
Parts of Confocal Microscope
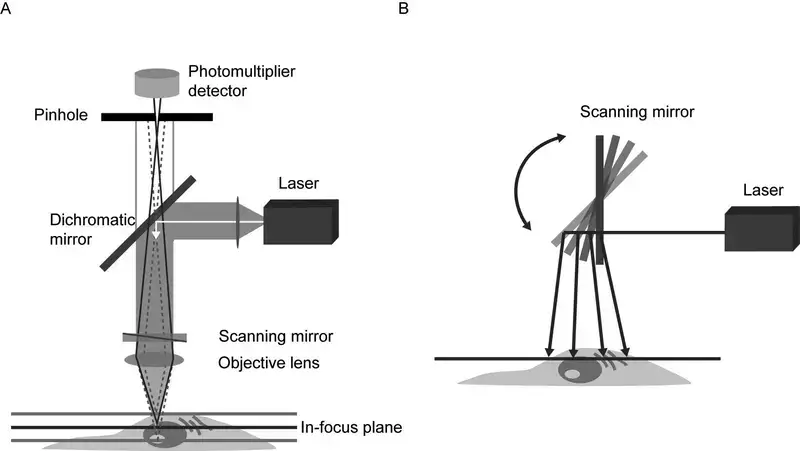
- Objective Lens
Located near the specimen, this lens focuses laser light onto the sample and collects emitted fluorescence. Its high numerical aperture ensures sharp imaging of fine structures. Early designs used basic lenses, but modern versions correct optical aberrations for improved performance. - Laser Light Source
Lasers provide intense, monochromatic light to excite fluorescent markers in the sample. Users select wavelengths via software to match specific dyes or proteins. Multiple lasers are often integrated, enabling imaging of diverse biological targets. - Scanning System
Mirrors or acousto-optic devices move the laser beam across the sample in a grid pattern. Galvanometer-based systems are common, though faster alternatives exist for capturing rapid processes. Earlier models relied on mechanical stages, which limited speed. - Beam Splitter
This optical component separates excitation light from emitted fluorescence. Dichroic mirrors—chosen based on wavelength—direct unwanted light away from detectors, enhancing image contrast. - Detectors
Photomultiplier tubes (PMTs) dominate, converting light particles into electrical signals. Advanced systems use hybrid detectors for greater sensitivity, particularly in low-light conditions. - Control and Data Acquisition System
A computer synchronises components, adjusts settings like pinhole size or laser intensity, and processes image data. Modern software simplifies operation, even for complex experiments like time-lapse studies. - Stage
Motorised stages allow exact movement in three dimensions, crucial for imaging multiple regions or thick samples. Manual adjustment is rare outside basic training setups. - Z-Control
A motorised focus mechanism shifts the objective lens vertically with nanometre-level precision. This enables detailed 3D reconstructions by capturing sequential optical sections. - Eyepieces
Though digital displays now dominate, traditional eyepieces remain useful for initial setup checks. Their role has diminished as real-time imaging shifts to monitors. - Pinhole
This adjustable aperture blocks out-of-focus light, ensuring only signals from the focal plane reach the detector. Smaller apertures improve resolution but require brighter samples. - Photomultiplier Tube (PMT)
PMTs amplify faint signals, with sensitivity settings adjustable to prevent overexposure. Newer models reduce noise, improving image quality in dim conditions.
Integration of Components
Confocal microscopy merges precision optics, automated controls, and digital processing. Early systems faced challenges like slow imaging speeds and photodamage, but iterative improvements—such as resonant scanners and spectral detection—have expanded applications. Today, these microscopes are indispensable in cell biology, neuroscience, and materials research, offering insights into subcellular dynamics and nanoscale structures. Their evolution reflects broader trends in instrumentation: balancing technical complexity with user accessibility.
Types of Confocal Microscope
Confocal microscopy has evolved significantly, offering distinct systems tailored to diverse research needs. Below are key variants, each leveraging unique mechanisms to enhance imaging precision and adaptability.
- Confocal Laser Scanning Microscope (CLSM)
Employing oscillating mirrors that raster-scan specimens along X and Y axes, this system directs emitted light through a pinhole aperture before detection. The descanning process ensures only in-focus photons reach the detector, enabling optical sectioning. Its high-resolution capability suits fixed samples, though prolonged laser exposure risks photobleaching. - Spinning Disk (Nipkow Disk)
A rotating disk embedded with multiple pinholes illuminates the sample in parallel, reducing excitation energy per unit area. Lower phototoxicity and rapid acquisition make it ideal for live-cell imaging. Extended observation periods are feasible here, unlike CLSM, which demands higher-intensity illumination. - Microlens-Enhanced Dual Spinning Disk
Developed by Yokogawa Electric, this variant incorporates a secondary disk fitted with microlenses positioned anterior to the pinhole array. By focusing broadband light into each aperture, throughput efficiency improves markedly. Enhanced sensitivity permits imaging of dim fluorescent markers, addressing limitations of conventional spinning disks. - Programmable Array Microscope (PAM)
Spatial light modulators (SLMs) replace physical apertures here, dynamically adjusting opacity or reflectivity to control light paths. Coupled with microelectrochemical mirrors, SLMs enable programmable patterning of excitation light. Captured via CCD cameras, images benefit from adaptive optical control, facilitating customisable resolution and depth profiling.
Comparative Applications
- CLSM: Dominates fixed-tissue studies requiring high axial resolution.
- Spinning Disk: Preferred for live-cell dynamics due to reduced photodamage.
- Microlens Systems: Utilised in low-light scenarios, such as quantum dot imaging.
- PAM: Adapts to complex 3D structures via software-driven aperture modulation.
Technical Evolution
Early confocal systems relied on mechanical scanning, limiting speed and flexibility. Innovations like microlens integration and SLMs reflect shifts towards modularity and computational imaging. Modern iterations balance photon efficiency with spatial fidelity, underscoring microscopy’s progression from static observation to dynamic, quantitative analysis.
Operational Considerations
While CLSM remains widespread, spinning disks dominate developmental biology. Dual-disk systems, though costly, mitigate trade-offs between speed and sensitivity. PAM’s programmability suits exploratory research, yet demands advanced computational resources. Selecting an appropriate type hinges on balancing resolution, phototoxicity, and specimen viability—a decision shaped by both historical precedent and contemporary experimental demands.
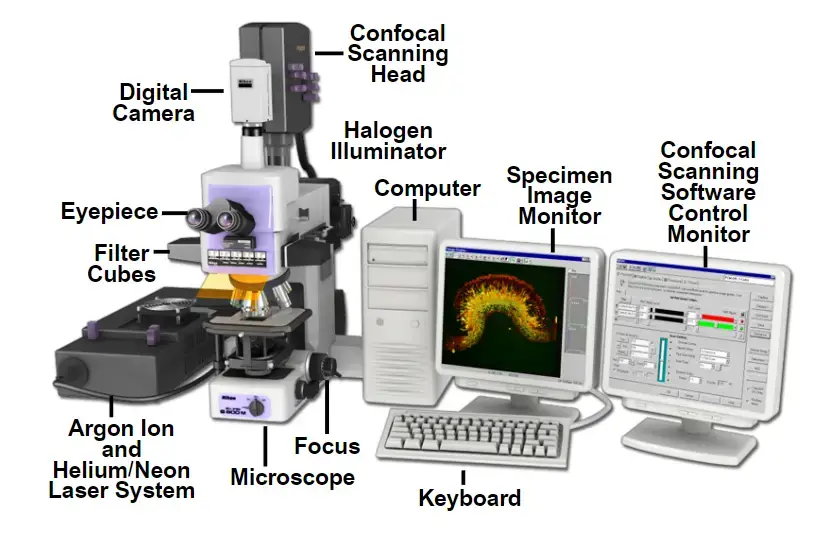
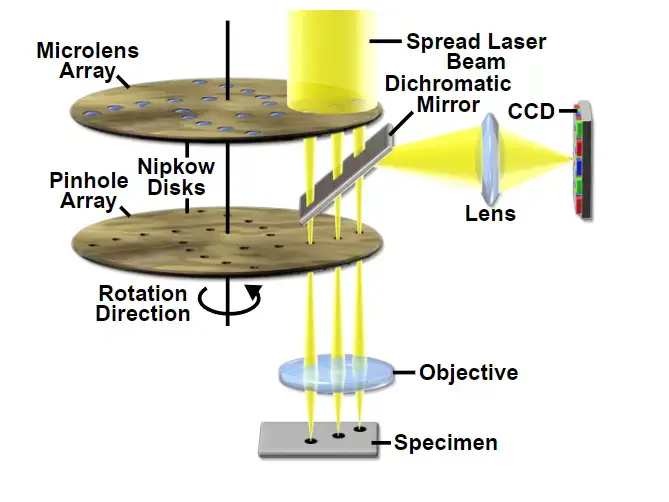
Application of Confocal Microscope
- Studying living cells in 3D
- Lets researchers take razor-sharp “optical slices” of cells or tissues and stack them into 3D models. Perfect for watching mitochondria buzz around or tracking how cells split during mitosis.
- Watching cells move and interact in real time
- Low-power lasers allow hours of filming live cells without frying them. Think calcium waves flashing like tiny signals or vesicles shuttling cargo inside a cell.
- Mapping molecular handshakes
- Tools like FRET (fancy energy transfer between molecules) show when proteins hug each other or how DNA interacts with enzymes.
- Fighting cancer and brain diseases
- Helps spot sneaky tumor cells in biopsies or map tangled neurons in Alzheimer’s studies. Clinicians even use it to scan corneas and retinas for defects.
- Spying on embryo development
- No need to slice delicate embryos! Confocal scopes track how cells migrate or specialize as organisms grow—like watching a tadpole turn into a frog, but at a microscopic level.
- Testing new medicines
- Shows whether drug particles reach their targets in tissues or how fast a cream penetrates skin. Pharma labs rely on this for smarter drug designs.
- Solving material science puzzles
- Reveals cracks in coatings, nanoparticle shapes, or how polymers behave under stress. Engineers use these details to build better phone screens or airplane parts.
- Gardening at the cellular level
- Peek into plant roots or leaves to see how they fight infections or build sturdy cell walls. Handy for creating pest-resistant crops!
- Forensics and quality checks
- From analyzing paint chips in crime scenes to spotting defects in microchips, confocal scopes act like super-powered magnifying glasses for industries.
- Combining with other tech for deeper insights
- Pair it with multiphoton lasers to image thick brain tissue or use time-lapse mode to document slow processes, like crystals forming or wounds healing.
Advantages of Confocal Microscopy
- Elimination of out-of-focus glare via pinhole-based spatial filtering.
- Enhanced lateral (xy) and axial (z) resolution for precise subcellular imaging.
- Compatibility with live-cell imaging protocols and fixed-tissue studies.
- Non-destructive optical sectioning enabling volumetric reconstruction.
- Uniform illumination across specimens, reducing intensity artefacts.
- Dynamic magnification control through electronic zoom adjustments.
- Generation of multidimensional datasets (4D: x, y, z, time) for complex analyses.
Disadvantages of Confocal Microscopy
- Steep initial and ongoing costs
Confocal systems aren’t cheap. The lasers, specialized optics, and detectors drive up the price, and maintaining them (e.g., replacing aging lasers or recalibrating optics) adds long-term expenses. - Requires serious training and expertise
Optimizing settings like pinhole alignment, laser power, and detector gain isn’t intuitive. New users often struggle with balancing image quality and avoiding artifacts. - Harsh on delicate samples
The intense laser light fries fluorescent dyes (photobleaching) and stresses live cells (phototoxicity). This makes long-term live-cell imaging tricky—your cells might not survive the experiment! - Not the fastest option for dynamic events
Point-scanning systems build images pixel by pixel, which is slow. If you’re watching rapid processes (e.g., calcium sparks), you might miss key details. - Struggles with thick specimens
Light scatters in dense or opaque tissues, limiting how deep you can image. For brain slices or embryos, two-photon microscopy often works better. - Weak signals get weaker
The pinhole blocks out-of-focus light but also throws away useful signal. Dim samples force you to crank up the laser, worsening photobleaching—a lose-lose situation. - Fussy alignment needs
Even minor misalignments in the pinhole or optics create artifacts (e.g., streaks, shadows). Keeping the system perfectly tuned is a never-ending task. - Limited viewing area
The scanning process captures smaller regions compared to wide-field microscopes. Studying large samples? Prepare to stitch multiple images together. - High-maintenance relationship
Lasers degrade, mirrors drift, and alignment slips. You’ll need regular tech support and patience for downtime. - Data-hoarding requirements
High-resolution 3D stacks (z-stacks) eat up storage space. A single experiment can generate gigabytes—better invest in a good hard drive! - Can’t see the ultra-small
While sharper than standard microscopy, confocal can’t beat super-resolution methods like STED or PALM. Need nanoscale details? Time to upgrade. - Frustrating for lengthy live-cell studies
Even with upgrades, phototoxicity and slow imaging make alternatives (e.g., spinning-disk or light-sheet systems) better for hours-long observations.
Recent Advances in Confocal Microscopy
- Artificial Intelligence (AI) Integration:- Since the late 2010s, AI tools began to be incorporated into confocal systems. These algorithms automate reconstruction of images and analysis, identifying structures like mitochondria or lysosomes with high accuracy. However, these systems require powerful computers which are expensive, limiting their use in smaller labs.
- Handheld Confocal Devices– In 2022, portable units were developed using fiber-optic lasers. These devices are used for fieldwork, such as imaging algae in ponds or soil microbes, without needing a full laboratory setup. But their resolution is lower compared to traditional confocal microscopes, making them unsuitable for detailed nuclear studies.
- Multi-Laser Systems – Recent systems now use four lasers at once, allowing scientists to track multiple processes in a cell—like protein movement, calcium levels, and DNA repair—simultaneously. This reduces the time needed for experiments but increases complexity in aligning the lasers.
- Quantum Dots– Starting in 2021, quantum dots replaced older fluorescent dyes in some labs. These tiny crystals emit brighter light and last longer, reducing how often samples get damaged by light. However, they are costly to produce, with prices 10 times higher than traditional dyes.
Challenges
- Cost – Advanced confocal systems remain expensive. For example, AI-integrated microscopes require GPUs that add 15,000–15,000–20,000 to the price.
- Training Needs – Technicians must learn complex software for multi-laser systems, increasing setup time.
- Portability Trade-offs– While handheld units are convenient, they cannot achieve the 0.1 µm resolution of lab-based systems.
Future Developments
- Adaptive Optics– By 2025, mirrors that adjust shape in real time (used in telescopes) may fix blurring in thick tissues like tumors. Trials show a 40% improvement in image clarity.
- Eco-Friendly Dyes– Researchers are testing dyes made from plant proteins that break down after use. These could replace toxic chemicals by 2030, but their brightness is still 30% weaker.
Confocal microscope image
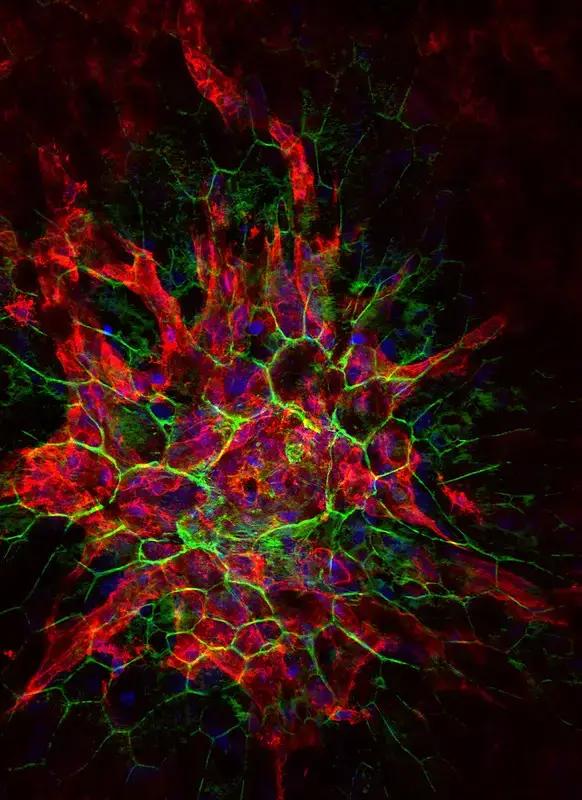
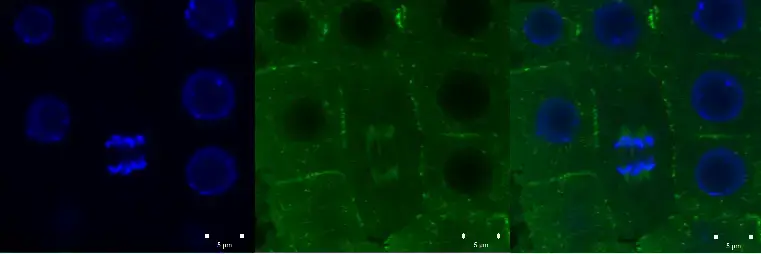
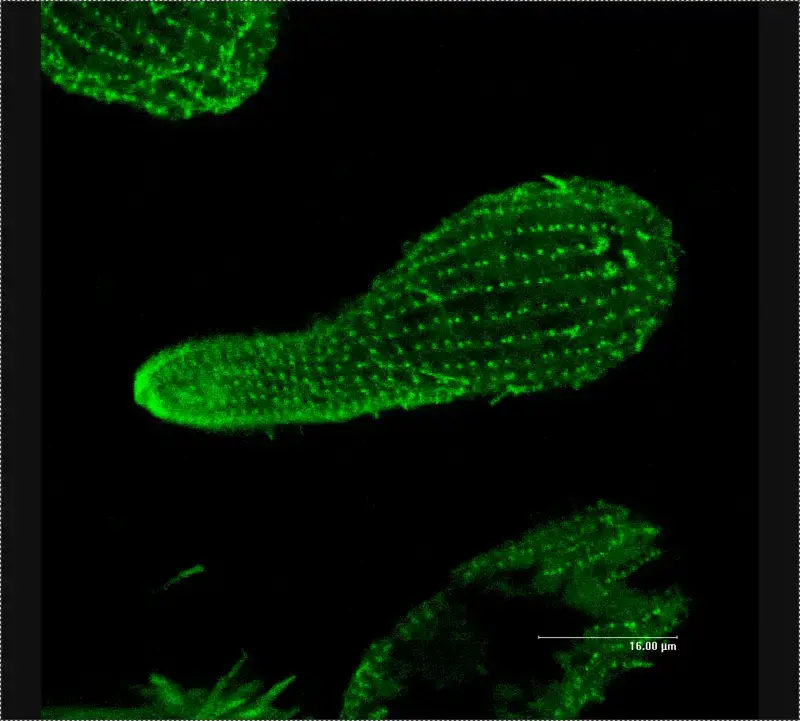
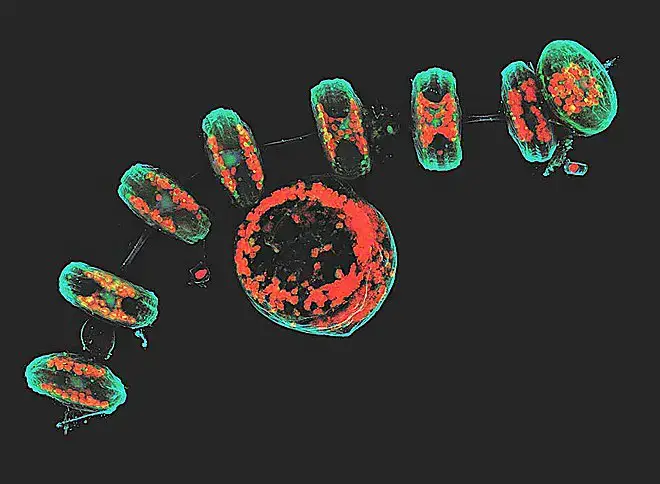
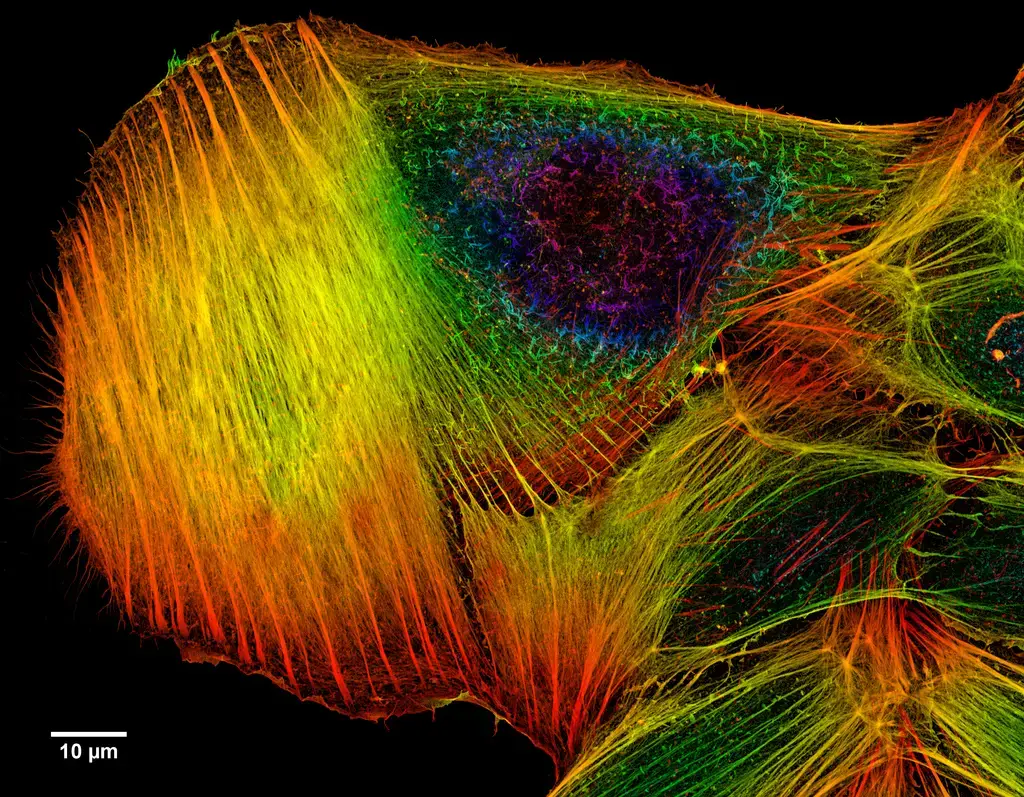
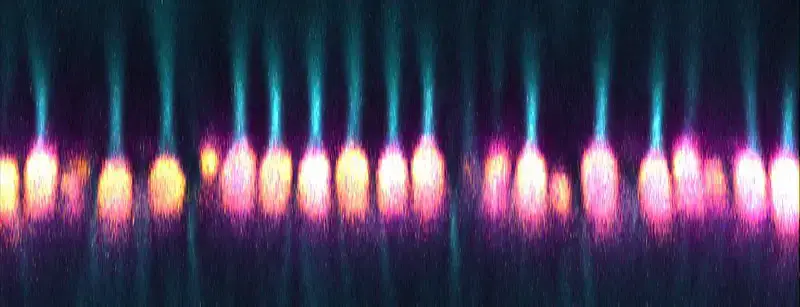
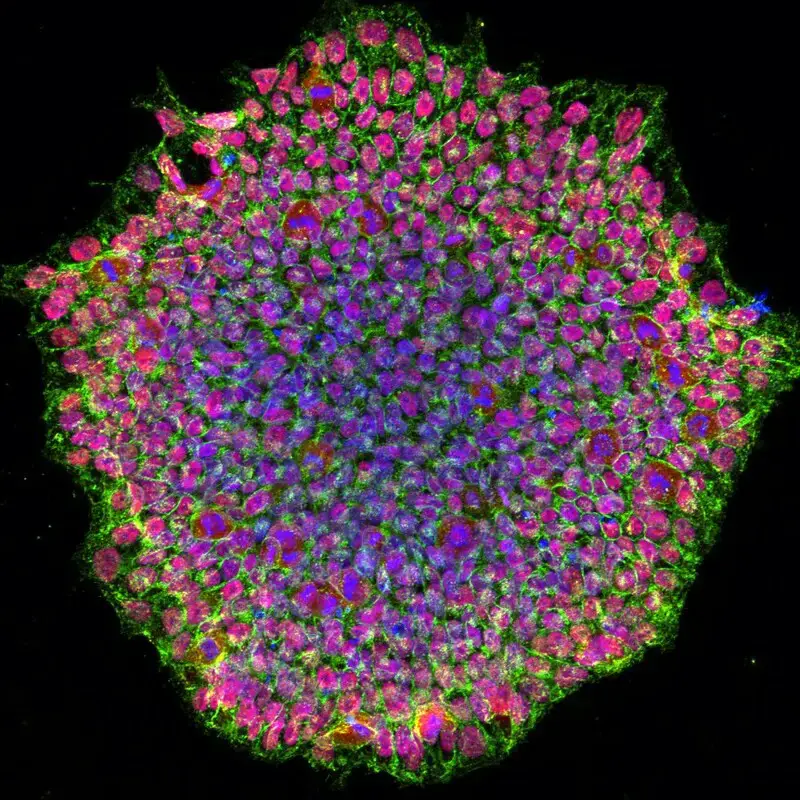
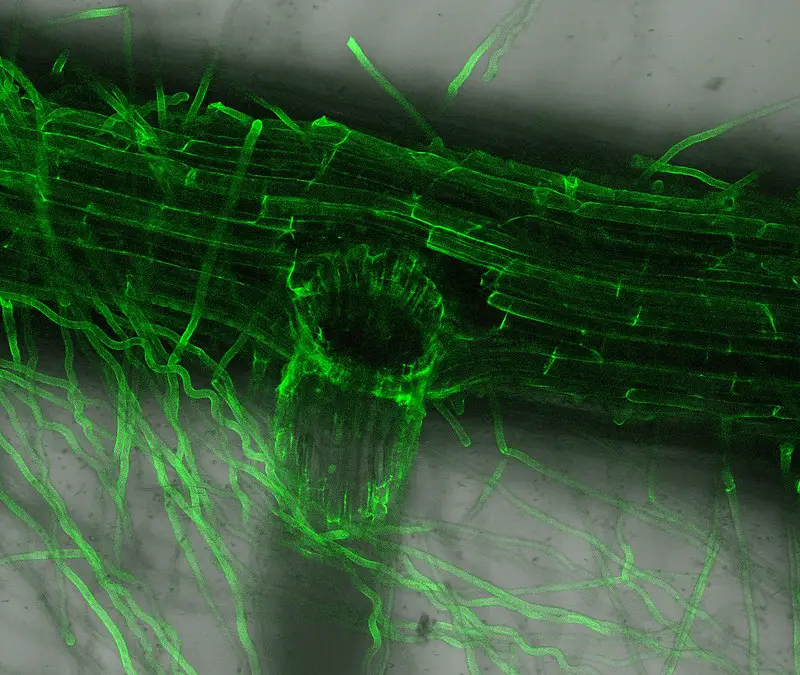
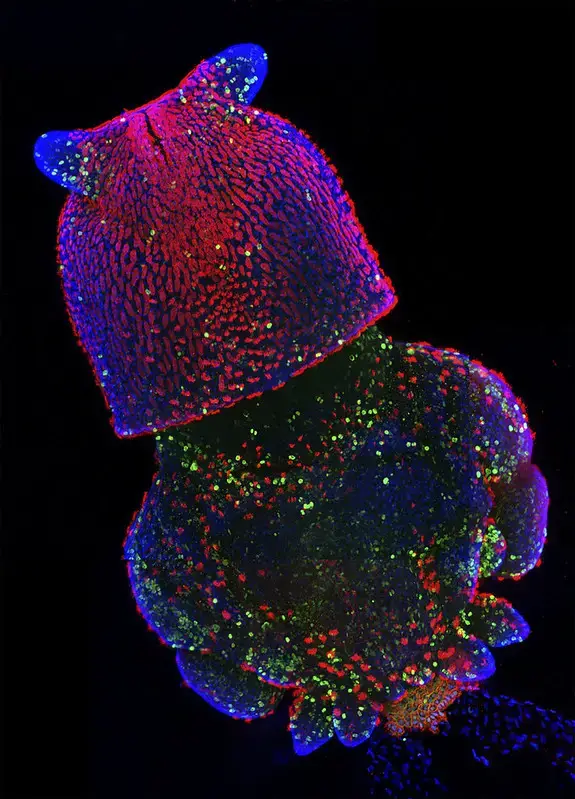
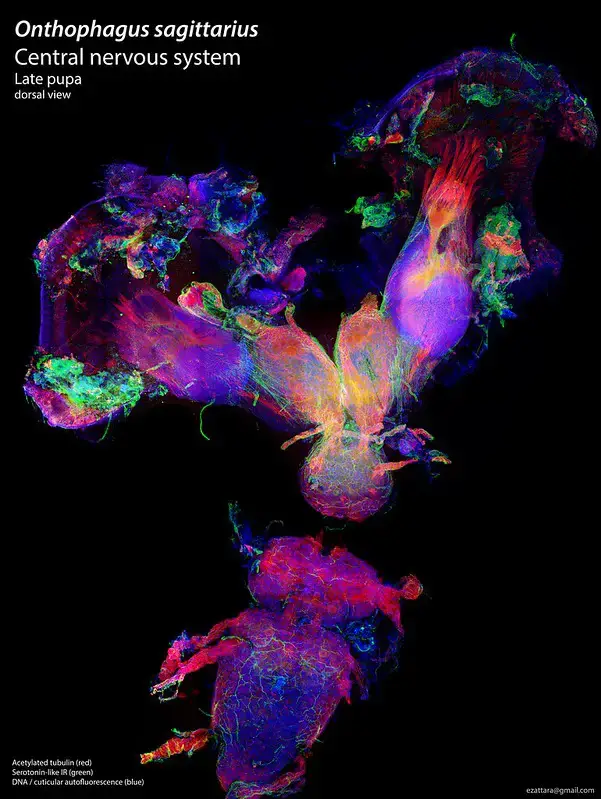
Check more Confocal microscope image – https://www.flickr.com/search/?text=Confocal%20microscope%20image
- Elliott AD. Confocal Microscopy: Principles and Modern Practices. Curr Protoc Cytom. 2020
- Mar;92(1):e68. doi: 10.1002/cpcy.68. PMID: 31876974; PMCID: PMC6961134.
- https://bitesizebio.com/19958/what-is-confocal-laser-scanning-microscopy
- https://www.microscopemaster.com/confocal-microscope.html
- https://ibidi.com/content/216-confocal-microscopy
- http://www.physics.emory.edu/faculty/weeks//confocal/
- https://www.olympus-lifescience.com/en/microscope-resource/primer/techniques/confocal/confocalintro/
- https://www.future-science.com/doi/full/10.2144/000112089
- https://www.microscopyu.com/techniques/confocal/introductory-confocal-concepts