What is Polysaccharide?
- Polysaccharides, also known as polycarbohydrates, are the most abundant type of carbohydrates found in food. They are large, complex molecules composed of long chains of monosaccharide units that are linked together by glycosidic linkages. These carbohydrates can undergo hydrolysis, a reaction with water, using amylase enzymes as catalysts, which breaks them down into constituent sugars such as monosaccharides or oligosaccharides. Polysaccharides can have varying structures, ranging from linear to highly branched configurations.
- Examples of polysaccharides include storage polysaccharides like starch, glycogen, and galactogen, as well as structural polysaccharides such as cellulose and chitin. Polysaccharides are often heterogeneous, meaning they may contain slight modifications of the repeating monosaccharide unit. The structure of these macromolecules gives them distinct properties that differ from their individual monosaccharide components. They can be amorphous or even insoluble in water.
- When a polysaccharide consists of only one type of monosaccharide, it is called a homopolysaccharide or homoglycan. On the other hand, if it contains more than one type of monosaccharide, it is referred to as a heteropolysaccharide or heteroglycan.
- Natural saccharides, including polysaccharides, are typically composed of simple carbohydrates called monosaccharides, with a general formula of (CH2O)n, where “n” represents three or more. Common examples of monosaccharides are glucose, fructose, and glyceraldehyde.
- In contrast, polysaccharides have a general formula of Cx(H2O)y, where “x” is usually a large number between 200 and 2500. When the repeating units in the polymer backbone consist of six-carbon monosaccharides, the general formula simplifies to (C6H10O5)n, with the value of “n” typically ranging from 40 to 3000.
- As a general guideline, polysaccharides contain more than ten monosaccharide units, while oligosaccharides consist of three to ten monosaccharide units. However, the precise cutoff can vary depending on the convention used. Polysaccharides play important roles in living organisms, primarily related to structure or storage.
- For example, starch, a glucose polymer, serves as a storage polysaccharide in plants, existing in the forms of amylose and the branched amylopectin. Animals store a similar glucose polymer called glycogen, often referred to as “animal starch,” which is more densely branched and metabolized quickly to suit their active lifestyles. Bacterial polysaccharides contribute to bacterial multicellularity.
- Structural polysaccharides include cellulose and chitin. Cellulose is abundant in the cell walls of plants and other organisms, making it one of the most prevalent organic molecules on Earth. It finds numerous applications in industries such as paper, textiles, and the production of rayon, cellulose acetate, celluloid, and nitrocellulose.
- Chitin has a similar structure to cellulose but possesses nitrogen-containing side branches that enhance its strength. It is found in arthropod exoskeletons and certain fungal cell walls. Chitin also has various applications, including its use in surgical threads. Other examples of polysaccharides include callose, laminarin, chrysolaminarin, xylan, arabinoxylan, mannan, fucoidan, and galactomannan.
- In summary, polysaccharides are large molecules made up of multiple monosaccharide units. They can be either homopolysaccharides, consisting of a single type of monosaccharide, or heteropolysaccharides, containing different types of monosaccharides.
- Depending on the arrangement of monosaccharides and their linkages, polysaccharides can have linear or branched structures. They are essential biomolecules, serving as a major source of energy in animal cells and playing a structural role in plant cells. The diversity of polysaccharides contributes to their wide range of functions and applications in biological systems.
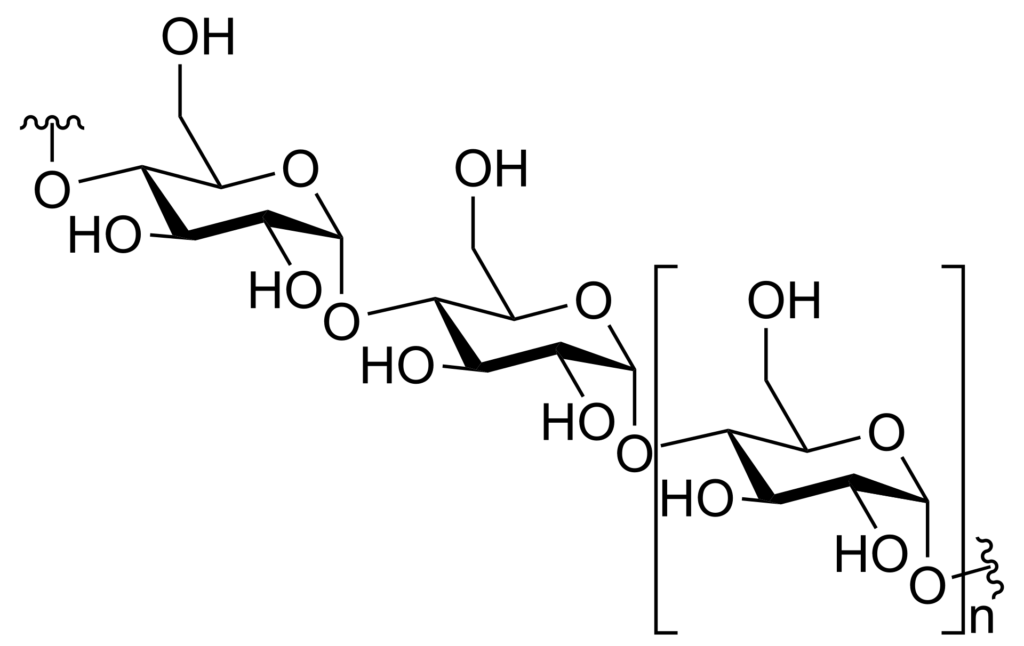
Characteristics of Polysaccharides
Polysaccharides possess several characteristic properties:
- Taste: Polysaccharides are not sweet in taste, unlike monosaccharides or disaccharides, which often have a sweet flavor.
- Solubility: Many polysaccharides are insoluble in water. This hydrophobic nature is due to the presence of numerous hydrogen bonds within the polysaccharide molecules, preventing water molecules from infiltrating and dissolving them.
- Crystallization: Polysaccharides do not form crystals when they are dried out or undergo desiccation. Instead, they tend to become amorphous, forming powdery or gel-like substances.
- Molecular Weight: Polysaccharides are high molecular weight carbohydrates. They consist of long chains of monosaccharide units, resulting in larger molecular sizes compared to monosaccharides or disaccharides.
- Composition: Polysaccharides are composed of hydrogen, carbon, and oxygen atoms. The hydrogen-to-oxygen ratio is generally 2:1, reflecting the typical composition of carbohydrates.
- Osmotic Activity: Inside cells, polysaccharides are compact and osmotically inactive. This means they do not significantly contribute to osmotic pressure or water movement across cell membranes.
- Extraction: Polysaccharides can be extracted and isolated from natural sources, such as plants or microorganisms, to obtain a white powder or purified form.
Chemically, polysaccharides are characterized by their complex structure, consisting of multiple monosaccharide units linked together by glycosidic bonds. The general chemical formula of polysaccharides is (C6H10O5)n, where “n” represents the number of monosaccharide units in the polysaccharide chain.
Polysaccharides exhibit great structural diversity, ranging from simple linear structures to highly branched architectures. They can be heterogeneous, meaning they may contain slight modifications or variations in their repeating units. Depending on their specific composition and structure, polysaccharides may exhibit amorphous properties or have limited solubility in water.
Overall, polysaccharides play crucial roles in biological systems, serving as storage molecules, structural components, and energy sources. Their diverse properties and functionalities make them essential biomolecules with a wide range of applications.
Structure of Polysaccharide
- The structure of polysaccharides is determined by the arrangement of monosaccharide units and the type of glycosidic bonds between them. Polysaccharides are formed through a dehydration reaction, where a hydroxyl group is removed from one monosaccharide and a hydrogen atom is removed from the hydroxyl group of another monosaccharide. The resulting bond, called a glycosidic bond, is formed when an oxygen molecule bridges two carbon rings.
- The specific arrangement of monosaccharide units and the type of glycosidic bond formed between them contribute to the overall structure and properties of the polysaccharide. For example, a polysaccharide used for energy storage will have a structure that allows for easy access to the monosaccharide units when they need to be broken down for energy. On the other hand, a polysaccharide used for structural support will typically consist of long chains of monosaccharides that form fibrous structures.
- The interactions between hydroxyl groups, side groups, the configuration of the molecules, and the enzymes involved also play a role in determining the structure of polysaccharides. These factors can influence the branching patterns, the degree of polymerization, and the overall conformation of the polysaccharide.
- One common example of a structural polysaccharide is cellulose, which is composed of long chains of glucose monosaccharides. The glycosidic bonds between the glucose units in cellulose result in a linear structure. Multiple cellulose strands can interact through hydrogen bonding, forming a network that provides strength and rigidity.
- In summary, the structure of polysaccharides is determined by the arrangement of monosaccharide units, the type of glycosidic bonds between them, and the interactions between different functional groups. These structural characteristics contribute to the diverse functions and properties exhibited by polysaccharides in biological systems.
Solubility of polysaccharides
The solubility of polysaccharides can vary widely depending on their chemical structure and interactions with water molecules. Some polysaccharides are insoluble in water, such as cellulose, while others are soluble only in hot water, like starch. On the other hand, certain polysaccharides readily dissolve in cold water, including pullulan and gum arabic.
The dissolution process of polysaccharides differs from that of small crystalline molecules. While small molecules typically disintegrate their crystalline structure to release separate atoms or ions, the dissolution of polysaccharides involves a continuous hydration process. It entails the conversion of inter-polysaccharide binding to polysaccharide-water binding, especially since most non-starch polysaccharides exist in an amorphous state. During dissolution, the molecules assume lower-energy conformations, and entropy plays a role in assisting the process.
Polysaccharides possess a strong affinity for water due to the presence of multiple hydroxyl (OH) groups. However, this also leads to significant interactions among polysaccharide molecules through hydrogen bonding. The solubility of polysaccharides depends on the balance between molecule-molecule interactions and molecule-water interactions. For soluble polysaccharides, the interactions with water are energetically favorable, and the solvent forms a solvating envelope around the polymer chain, keeping the polysaccharide molecules separated. In contrast, polysaccharides with poor water solubility experience dominant intramolecular interactions between polymer segments, resulting in aggregation, precipitation, or gelation when an ordered molecular structure, such as a junction zone, forms. Under specific conditions, the polymer-polymer interaction can precisely compensate for the polymer-water interaction, leading to a state known as the theta condition.
The second virial coefficient (A2), which reflects the pair-wise potential contribution to the gas pressure, can provide insights into the polymer-water interaction. A positive A2 indicates a good solvent, a negative A2 indicates a poor solvent, and an A2 value of zero indicates the theta condition. Experimental determination of the second virial coefficient for polysaccharides in aqueous solutions can be achieved through static light scattering using the Zimm plot method. This allows for the characterization of the solvation behavior of polysaccharides.
For instance, the second virial coefficient (A2) of xyloglucans from flaxseed kernel cell wall was reported as positive values in 0.5 M NaOH solution and water, indicating a good solvent condition. However, in 0.1 M NaNO3 solution, A2 was negative, suggesting a poor solvent condition for xyloglucan. Mild alkaline conditions, such as 0.5 M NaOH, have been found to be favorable solvents for several polysaccharides due to their ability to break down intermolecular hydrogen bonding and eliminate aggregates in aqueous solutions.
Overall, the solubility of polysaccharides is influenced by the balance between molecule-molecule interactions and molecule-water interactions, as well as the specific chemical structure of the polysaccharide. Understanding the solubility behavior of polysaccharides is crucial for their applications in various industries, including food, pharmaceuticals, and materials science.
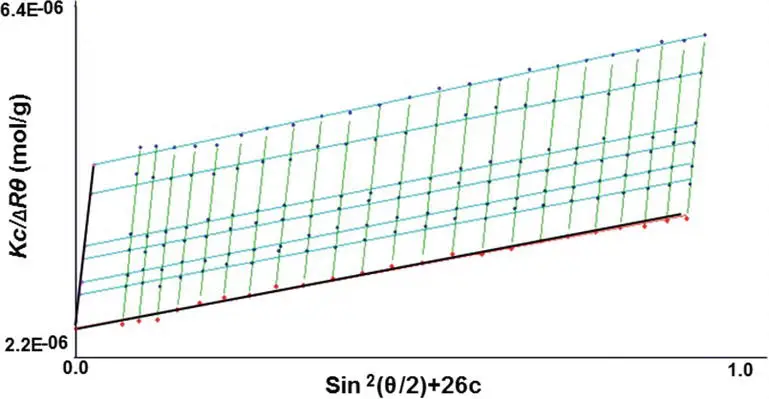
Classification of Polysaccharides
Polysaccharides can be classified into two main types based on their composition:
- Homopolysaccharides: These are polysaccharides composed of the same type of monosaccharide units. Examples of homopolysaccharides include:
- Glycogen: It consists of a large chain of glucose molecules and serves as a storage form of glucose in animals and fungi.
- Cellulose: Cellulose forms the structural component of the cell wall in plants. It is composed of long chains of β-glucose units connected by β-glycosidic bonds.
- Starch: Starch is a storage polysaccharide found in plants, fruits, and seeds. It is made up of two components, amylose and amylopectin, which are both composed of glucose units.
- Inulin: Inulin is composed of fructose units linked together in chains. It is found in tubers of plants such as dahlia and artichoke and serves as a reserve polysaccharide.
- Heteropolysaccharides: These are polysaccharides composed of different types of monosaccharide units. Examples of heteropolysaccharides include:
- Hyaluronic Acid: It consists of repeating units of D-glucuronic acid and N-acetyl-glucosamine. Hyaluronic acid is found in connective tissues and plays a role in lubrication and hydration.
- Heparin: Heparin is composed of D-glucuronic acid, L-iduronic acid, and N-sulfo-D-glucosamine. It is primarily found in mast cells and blood and functions as an anticoagulant.
- Chondroitin-4-sulfate: Chondroitin-4-sulfate is composed of D-glucuronic acid and N-acetyl-D-galactosamine-4-O-sulfate units. It is present in cartilages and contributes to their structural integrity.
- Gamma globulin: This polysaccharide consists of N-acetyl-hexosamine, D-mannose, and D-galactose units. It is found in blood and plays a role in immune response.
The classification of polysaccharides into homopolysaccharides and heteropolysaccharides is based on the similarity or difference in the types of monosaccharide units they contain. These polysaccharides exhibit diverse biological functions and are important components of various biological systems.
Polysaccharides can be classified based on their functional roles:
Polysaccharides can be classified into different categories based on their functional roles in organisms. Here are two major categories:
1. Storage Polysaccharides:
Storage polysaccharides are primarily involved in storing energy as food reserves. They act as a readily available source of energy when needed. Examples of storage polysaccharides include:
- a. Starch: Starch is a storage polysaccharide found in plants. It serves as the main energy storage molecule in plants and is stored in various plant organs such as seeds, tubers, and roots. Starch can be broken down into glucose molecules to provide energy for cellular processes.
- b. Glycogen: Glycogen is the storage polysaccharide found in animals, including humans. It is primarily stored in the liver and muscles. Glycogen functions as a readily available source of energy during periods of fasting or increased physical activity. Similar to starch, glycogen can be broken down into glucose units to fuel cellular metabolism.
2. Structural Polysaccharides:
Structural polysaccharides are involved in providing support and structure to cells and tissues. They contribute to the formation of various structural components in organisms. Examples of structural polysaccharides include:
- a. Cellulose: Cellulose is the most abundant structural polysaccharide found in plants. It forms the major component of plant cell walls, providing strength and rigidity. Cellulose consists of long chains of glucose molecules linked together by β-1,4 glycosidic bonds. It serves as a structural scaffold for plant cells and contributes to the overall integrity of plant tissues.
- b. Hemicellulose: Hemicellulose is another structural polysaccharide found in plant cell walls. It is a heterogeneous group of polysaccharides composed of various sugar units. Hemicellulose works in conjunction with cellulose to provide structural support to plant cells. It has a more amorphous structure compared to cellulose and plays a role in binding cellulose fibers together.
- c. Lignin: Although not strictly a polysaccharide, lignin is an important structural component in plants. It is a complex aromatic polymer that fills the spaces between cellulose and hemicellulose in the cell walls. Lignin provides rigidity and hydrophobicity to plant tissues, contributing to their mechanical strength and resistance to microbial degradation.
By classifying polysaccharides based on their functional roles, we can better understand their significance in various biological processes, including energy storage, structural support, and cellular integrity.
Homopolysaccharides
Homopolysaccharides are a type of polysaccharide that are composed of repeating units of only one type of monomer. These polysaccharides exhibit a uniform structure due to the repetitive nature of their monomeric units. Here are some examples of common homopolysaccharides:
1. Cellulose: Properties, Functions and Applications
Cellulose is a homopolysaccharide that serves as a fundamental structural component of the plant cell wall. It is composed of glucose units linked together by beta-1,4 glycosidic bonds, forming a linear and unbranched polymer. The discovery of cellulose is credited to the French scientist Anselme Payen.
In the biosphere, cellulose is one of the most abundant organic compounds. It provides strength and rigidity to plant cells, contributing to the overall structural integrity of plants. The interconnected network of cellulose fibers forms a strong matrix that supports the plant’s tissues and allows them to withstand mechanical stress.
While cellulose is present in the human diet through the consumption of plant-based foods, it is indigestible for humans. This is because humans lack the necessary enzymes, specifically cellulases, required to hydrolyze the beta-1,4 glycosidic bonds in cellulose. As a result, cellulose passes through the human digestive system largely unchanged and is excreted as fiber.
In contrast, certain animals, particularly herbivores, have developed a specialized digestive system to break down cellulose. These animals, such as cows, horses, and termites, possess symbiotic microorganisms, such as bacteria or protozoa, in their digestive tract. These microorganisms produce cellulases, enzymes capable of hydrolyzing the beta-1,4 glycosidic bonds in cellulose. As a result, herbivores can extract energy and nutrients from cellulose by allowing the microorganisms to ferment and break down cellulose in their digestive system.
The ability of herbivores to digest cellulose highlights the importance of cellulose as a renewable energy source in natural ecosystems. Through the action of specialized microorganisms, cellulose can be efficiently converted into usable energy and nutrients. Additionally, cellulose plays a crucial role in the carbon cycle, as the breakdown of cellulose releases carbon dioxide back into the atmosphere, which is then utilized by plants during photosynthesis.
In summary, cellulose is a linear, unbranched polymer of glucose units joined by beta-1,4 glycosidic linkages. It serves as a fundamental structural component of the plant cell wall and is abundantly found in the biosphere. While humans cannot digest cellulose due to the absence of cellulase enzymes, herbivores have evolved mechanisms to break down cellulose through symbiotic microorganisms in their digestive system, enabling them to derive energy from this complex carbohydrate.
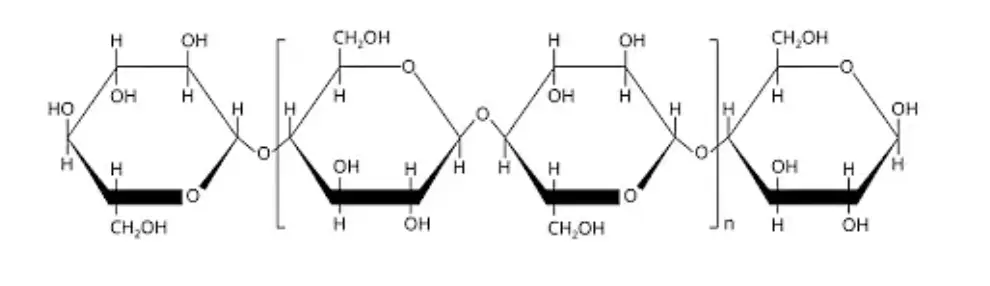
Properties of Cellulose
Cellulose exhibits several distinct properties that contribute to its unique characteristics and diverse applications:
- Tasteless and Odorless: Cellulose is devoid of any taste or odor, making it ideal for various food and non-food applications.
- Insolubility in Water: Cellulose is insoluble in water, meaning it does not dissolve in water to form a homogeneous solution. This property allows cellulose to retain its structural integrity in aqueous environments.
- Solubility in Organic Solvents: While insoluble in water, cellulose can be dissolved in certain organic solvents such as cuprammonium hydroxide or N-methylmorpholine N-oxide (NMMO). This solubility in organic solvents is often utilized in the production of regenerated cellulose fibers or films.
- Crystalline Structure: Cellulose possesses a crystalline structure, consisting of highly ordered regions. This arrangement gives cellulose a white, powdery appearance and contributes to its high tensile strength.
- Amorphous Transition: Cellulose can undergo an amorphous transition when exposed to high temperatures and pressure in the presence of water. This transition occurs at around 320°C and 25 MPa, causing the cellulose structure to become less ordered and lose its crystalline properties.
- Water Retention: The water retention capacity of cellulose varies depending on its source. Plant cellulose typically exhibits a water retention value of around 60%, while bacterial cellulose can retain up to 1000% of its own weight in water. This property is advantageous in applications such as wound dressings or moisture-absorbing materials.
- High Tensile Strength: Cellulose possesses remarkable tensile strength, comparable to that of steel. The strength of cellulose fibers arises from the alternating arrangement of glucose molecules in its structure, forming strong hydrogen bonds.
- Acid Hydrolysis: Cellulose can be broken down into glucose monomer units by treating it with concentrated mineral acids, typically under high temperatures. This process, known as acid hydrolysis, is utilized in various industries for the production of biofuels, paper pulp, and other cellulose-derived products.
- Cellulolysis and Thermolysis: Cellulolysis refers to the breakdown of cellulose, often through enzymatic or microbial action. Thermolysis, on the other hand, refers to the breakdown of cellulose when exposed to high temperatures without the involvement of enzymes or microorganisms. Both processes are significant in the degradation and utilization of cellulose.
Functions of Cellulose
Cellulose serves several important functions in different organisms and structures:
- Structural Support in Plants: Cellulose is a major component of plant cell walls, providing structural integrity and strength to plant tissues. It contributes to the rigidity and stability of plant structures, such as stems, leaves, and roots, enabling plants to maintain their shape and withstand mechanical stress.
- Flocculation and Attachment in Bacteria: In certain bacteria, cellulose plays a role in flocculation, which is the clumping together of bacterial cells. Cellulose allows bacteria to adhere to surfaces, including plant surfaces, aiding in their attachment and colonization. This attachment can be important for establishing beneficial interactions with plants or forming biofilms.
- Shape and Structure Maintenance in Bacteria: Cellulose also helps maintain the shape and structure of bacterial cells. It forms a protective layer around bacterial cells, providing stability and protection against environmental stresses. This is particularly important for bacteria living in aquatic or soil environments.
- Cell Wall Component in Algae and Fungi: Cellulose is a vital component of the cell walls in algae and fungi. It contributes to the structural strength of these organisms, enabling them to withstand osmotic pressure changes and maintain their overall shape. In fungi, cellulose is often found in the cell walls of certain groups, such as oomycetes.
Applications of Cellulose
Cellulose finds a wide range of applications due to its unique properties and abundance. Some notable applications of cellulose include:
- Paper and Cardboard Production: Cellulose is a key component in the manufacture of various paper products, including paper, cardboard, paperboard, and cardstocks. Its fibrous structure allows for the formation of a strong and flexible material that is widely used in packaging, printing, writing, and many other industries.
- Electrical Insulation: Cellulose is utilized in the production of electrical insulation paper. Its high dielectric strength and insulating properties make it suitable for applications where electrical conductivity must be minimized, such as in transformers, capacitors, and electrical cables.
- Gunpowder and Biofuel: Cellulose can be converted into cellulose nitrate, a key ingredient in the production of gunpowder. Its combustible nature and availability make it a valuable resource for this application. Moreover, cellulose-based biofuels, such as cellulosic ethanol, can be derived from cellulose-rich materials, offering a renewable and sustainable alternative to fossil fuels.
- Pharmaceutical Stabilizer: Cellulose is used in the pharmaceutical industry as a stabilizer in various formulations. It helps maintain the integrity, consistency, and shelf life of drugs by improving their stability, controlling moisture absorption, and enhancing the release of active ingredients.
- Textile and Apparel: Cellulose-based fibers, such as rayon and viscose, are widely used in the textile industry. These fibers are derived from cellulose and can be processed into fabrics with desirable properties, including softness, breathability, and absorbency. Cellulose fibers are used in the production of clothing, bedding, upholstery, and other textile products.
- Food and Personal Care Products: Cellulose derivatives, such as carboxymethyl cellulose (CMC) and microcrystalline cellulose (MCC), are employed in the food and personal care industries. CMC is used as a thickener, stabilizer, and emulsifier in a variety of food products, while MCC serves as a bulking agent, texture enhancer, and flow aid in dietary supplements, cosmetics, and pharmaceutical formulations.
These are just a few examples of the diverse applications of cellulose. Its abundance, renewable nature, and versatile properties make it a valuable resource in numerous industries, contributing to the development of sustainable materials, energy sources, and everyday products.
2. Chitin: Properties, Functions and Applications
Chitin is a polysaccharide that serves as a structural component in the exoskeleton of arthropods (such as insects, crustaceans, and spiders) and the cell walls of fungi. Here are some key points about chitin:
- Composition and Structure: Chitin is composed of long-chain polymers of N-acetyl-D-glucosamine, which is a derivative of glucose. The N-acetyl-D-glucosamine units are linked together by beta 1-4 glycosidic bonds, forming a linear and long-chain structure. Unlike cellulose, which consists of glucose units, chitin contains N-acetyl-glucosamine units.
- Abundance: Chitin is the second most abundant natural biopolymer after cellulose, and it is widely distributed in the natural world. It is particularly abundant in the exoskeletons of insects and crustaceans, as well as in the cell walls of fungi.
- Biodegradability: Chitin is biodegradable in the natural environment. The degradation process is catalyzed by chitinases, which are enzymes secreted by certain bacteria and fungi. These enzymes break down the chitin polymer into smaller molecules, which can be further metabolized by microorganisms.
- Similarities to Cellulose: Chitin shares some similarities with cellulose, another polysaccharide. Both chitin and cellulose are linear polymers, but their monomer units differ. Chitin is composed of N-acetyl-D-glucosamine, while cellulose is made up of glucose units. Despite these differences, chitin and cellulose both contribute to the structural integrity of organisms.
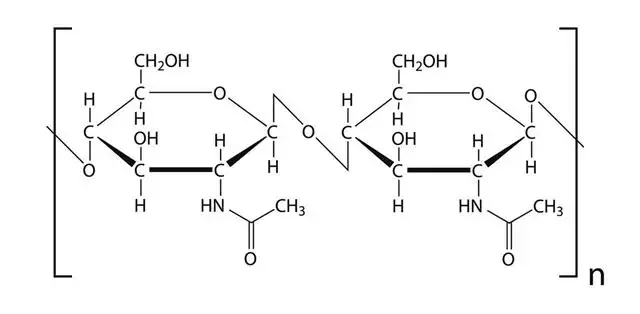
Chitin Properties
Chitin possesses several distinct properties that contribute to its versatility and various applications. Here are some key properties of chitin:
- N-deacetylation and Chitosan Formation: Chitin can undergo a process called N-deacetylation, resulting in the formation of chitosan. During this process, some of the acetyl groups attached to the N-acetyl-D-glucosamine units in chitin are removed, leading to the formation of chitosan. Chitosan is a crystalline, cationic, and hydrophilic polymer with unique properties.
- Crystalline Structure: Chitin exhibits polymorphism, meaning it can exist in different crystalline modifications. The three main crystalline forms of chitin are alpha, beta, and gamma. Each modification has a distinct arrangement of the chitin chains and different physical properties.
- Gelation and Film-Forming Properties: Chitosan, derived from chitin, demonstrates excellent gelation and film-forming properties. It has the ability to form gels in the presence of certain conditions such as pH, temperature, and concentration. Chitosan gels can find applications in various fields, including food, pharmaceuticals, and biomedical engineering. Additionally, chitosan can form films that possess barrier properties, making it useful for coating and packaging applications.
- Biocompatibility and Biodegradability: Chitin and chitosan are known for their biocompatibility, meaning they are well-tolerated by living organisms. This property is valuable in biomedical applications such as tissue engineering and drug delivery systems. Furthermore, chitin and chitosan are biodegradable, allowing them to naturally break down in the environment over time. This characteristic is advantageous for sustainable and eco-friendly applications.
- Chelation and Adsorption Properties: Chitosan has a unique ability to chelate or bind with metal ions. This property makes it useful in applications involving metal ion removal, such as water treatment and wastewater remediation. Chitosan can also adsorb or trap various substances, including dyes, heavy metals, and organic compounds, making it effective for purification and adsorption processes.
Chitin Functions
Chitin serves several important functions in organisms where it is naturally present. Here are some key functions of chitin:
- Structural Support: One of the primary functions of chitin is to provide stability and rigidity to the cell wall structures of organisms. It is a major component of the exoskeleton found in arthropods such as shrimps, insects, lobsters, and crabs. The chitin-based exoskeleton provides support and protection to the internal organs and tissues, allowing these organisms to maintain their shape and withstand mechanical stresses.
- Peritrophic Membrane Formation: Chitin plays a crucial role in the formation of the peritrophic membrane in insects’ midgut. The peritrophic membrane is a protective layer that lines the gut and aids in the digestion process. It acts as a physical barrier, preventing the entry of harmful substances, such as toxins and pathogens, into the gut epithelium. The chitin-based peritrophic membrane also facilitates the mechanical breakdown of food, enhances nutrient absorption, and protects the gut from mechanical damage.
- Defense Mechanism: Chitin contributes to the defense mechanisms of certain organisms. In the cell walls of certain fungi and molds, chitin acts as a barrier against environmental stresses and provides protection against pathogens and predators. The presence of chitin in these cell walls enhances the structural integrity of the organisms, making them more resistant to mechanical damage and potential attacks.
- Molting and Growth: In arthropods, chitin plays a vital role during growth and molting processes. As arthropods grow, they need to shed their exoskeleton to accommodate their increasing body size. Chitin provides the necessary flexibility for the exoskeleton to expand during growth, and it also forms the new exoskeleton after molting. This process allows arthropods to continue their development and adapt to changing environmental conditions.
- Medical Applications: Chitin and its derivatives have found applications in the medical field. Chitosan, a derivative of chitin, possesses antimicrobial properties and has been utilized in wound healing and tissue engineering. Chitosan-based materials can promote tissue regeneration, control infections, and assist in drug delivery systems.
Chitin Applications
Chitin finds a wide range of applications due to its unique properties and biocompatibility. Here are some notable applications of chitin:
- Agriculture and Fertilizers: Chitin is used in the production of organic fertilizers. Chitin-based fertilizers are environmentally friendly, non-toxic, and biodegradable. They can improve soil health and promote sustainable agriculture by enhancing nutrient availability, stimulating microbial activity, and increasing crop productivity. Chitin helps in nutrient retention, water management, and disease resistance, making it an excellent choice for enhancing soil fertility and promoting plant growth.
- Health and Nutrition: Chitin has been studied for its potential health benefits. Consuming chitin in the diet, such as through dietary supplements or certain food sources, has been found to reduce cholesterol absorption efficiency. This property makes chitin a promising natural compound in managing cholesterol levels and promoting cardiovascular health.
- Biomedical Applications: Chitin and its derivative, chitosan, have several applications in the biomedical field. Chitosan’s unique properties, such as biocompatibility, biodegradability, and antimicrobial activity, make it suitable for various medical applications. Chitosan-based materials are used in wound healing as sutures or surgical threads. These materials facilitate wound closure, promote tissue regeneration, and reduce the risk of infection. Chitin and chitosan have also been explored for drug delivery systems, tissue engineering, and as scaffolds for regenerative medicine.
- Industrial and Environmental Applications: Chitin’s properties make it useful in various industrial applications. Chitin can be processed into chitosan, which is a versatile material used in the production of films, coatings, and membranes. These materials find applications in water treatment, filtration, and biomedical devices. Chitin and chitosan also have potential uses in the textile, paper, and cosmetic industries.
- Waste Management: Chitin can be derived from various sources, including waste materials such as shrimp shells, crab shells, and fungal mycelium. By utilizing these waste materials, chitin production contributes to waste reduction and recycling efforts. Chitin extraction and processing from waste materials provide an additional value to these resources, promoting sustainable waste management practices.
3. Starch: Properties, Functions and Applications
Starch is a complex carbohydrate that serves as a major energy storage molecule in plants. Here are some key points about starch:
- Composition: Starch is composed of repeating units of D-glucose molecules joined together by alpha-linkages. It consists of a mixture of two components: amylose and amylopectin. Amylose is a linear polymer of glucose with alpha-1,4 linkages, while amylopectin is a branched polymer with both alpha-1,4 and alpha-1,6 linkages.
- Abundance: Starch is one of the most abundant polysaccharides found in plants. It serves as the primary energy storage molecule in various plant tissues, such as seeds, tubers, and roots. Cereals (such as rice, wheat, and corn) and potatoes are examples of common food sources rich in starch.
- Digestion: Starch can be readily digested by both humans and animals due to the presence of the enzyme amylase. Amylase breaks down the alpha-linkages between glucose molecules, leading to the release of glucose units that can be absorbed and used as an energy source. Humans have amylase enzymes in their saliva and pancreas, while animals produce amylase in their saliva or within their digestive systems.
- Structure and Function: The structure of starch, with its combination of amylose and amylopectin, contributes to its unique properties. Amylose forms a helical structure, allowing it to pack tightly and form insoluble granules. Amylopectin, with its branched structure, provides increased solubility and accessibility for enzymatic degradation. The balance between amylose and amylopectin influences the texture and cooking properties of starchy foods.
- Industrial Applications: Starch has various industrial applications due to its versatile properties. It is widely used in the food industry as a thickening agent, stabilizer, and gelling agent. Starch-based products are employed in the production of sauces, soups, bakery products, and confectionery. Starch is also used in the manufacturing of biodegradable packaging materials, adhesives, and paper products.
- Modified Starches: Starch can be modified through chemical or enzymatic processes to enhance its functionality for specific applications. Modified starches are often used to improve texture, stability, and viscosity in food products. They can also be employed in pharmaceutical formulations, textile sizing, and as binders in various industries.
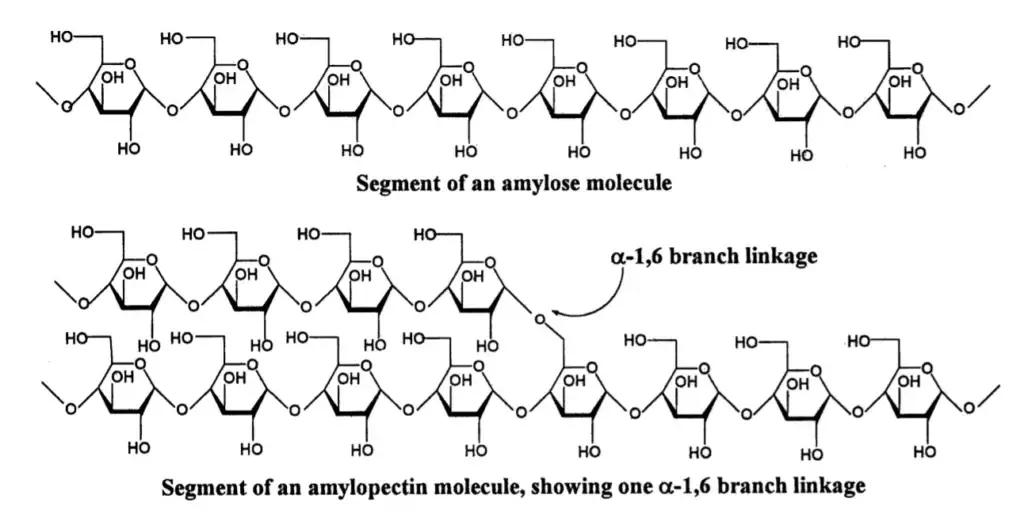
Starch Properties
Starch possesses several distinct properties, which contribute to its widespread applications. Here are some key points about the properties of starch:
- Appearance and Solubility: Starch is typically a white, tasteless powder. It is insoluble in cold water, alcohol, and many organic solvents. When starch is added to cold water, it forms a suspension but does not dissolve, resulting in a cloudy mixture.
- Chemical Composition: The chemical formula for starch is generally represented as (C6H10O5)n, where n represents the number of glucose molecules in the starch chain. Starch is a polysaccharide composed of glucose units joined together by alpha-linkages.
- Gelatinization: When starch is heated in the presence of water, it undergoes a process called gelatinization. During gelatinization, the starch granules absorb water, swell, and rupture, leading to the formation of a viscous gel. This property is important in various food applications, such as thickening sauces and providing structure to baked goods.
- Retrogradation: After gelatinization, when a starch gel cools or ages, retrogradation occurs. Retrogradation involves the reassociation of amylose and amylopectin molecules, resulting in the formation of a more rigid and less soluble starch network. This phenomenon contributes to the firming or staling of starchy foods over time.
- Thermal Degradation: Starch is susceptible to thermal degradation when exposed to dry heat. The breakdown of starch molecules by high temperatures leads to the formation of pyrodextrins. Pyrodextrins contribute to the browning of baked goods, such as bread crust, through the Maillard reaction.
- Granular Structure: Starch occurs naturally in the form of granules within plant cells. These granules exhibit different shapes and sizes depending on the plant source. The granular structure influences the properties and functionality of starch, including its gelatinization and thickening abilities.
- Functionality: Starch is valued for its thickening, gelling, and stabilizing properties in various food and industrial applications. It acts as a thickener by absorbing water and forming a viscous solution or gel. Starch also contributes to the texture, mouthfeel, and stability of food products.
- Modified Starches: Through physical, chemical, or enzymatic modifications, starch can be modified to enhance or alter its properties. Modified starches are commonly used in food applications to improve stability, freeze-thaw resistance, and tolerance to processing conditions.
Starch Functions
Starch serves important functions in both plants and animals. Here are the key functions of starch:
- Food Reserve in Plants: In plants, starch acts as a storage form of energy. During photosynthesis, plants produce glucose, which is then converted into starch and stored in various plant tissues, such as roots, tubers, seeds, and fruits. Starch provides a readily available source of energy for plants during periods of growth, development, or when external energy sources are limited, such as during winter or germination.
- Energy Source in Humans and Animals: In the human and animal diet, starch serves as a significant source of dietary carbohydrates. When consumed, starch is broken down into its constituent sugar molecules by the enzyme amylase. In the mouth and small intestine, amylase initiates the digestion of starch, breaking it down into smaller molecules like maltose and glucose. These sugar molecules are then absorbed into the bloodstream and serve as a readily available energy source for the body’s metabolic processes.
- Blood Glucose Regulation: Starch, being a complex carbohydrate, has a slower rate of digestion compared to simple sugars. This slower digestion and gradual release of glucose into the bloodstream help regulate blood sugar levels and provide a sustained energy supply. Starch-rich foods, such as whole grains, legumes, and starchy vegetables, are often recommended as part of a balanced diet for their contribution to long-lasting energy and stable blood glucose levels.
- Dietary Fiber: Certain types of starch, such as resistant starch, can act as dietary fiber. Resistant starch resists digestion in the small intestine and reaches the large intestine intact. In the colon, it undergoes fermentation by gut bacteria, producing short-chain fatty acids that contribute to gut health and provide additional energy for the body. Dietary fiber from starch-rich foods supports healthy digestion, promotes bowel regularity, and may have beneficial effects on overall gut health.
- Culinary and Food Industry Applications: Starch is widely used in the food industry for its functional properties. It serves as a thickening agent, stabilizer, and texturizer in a variety of food products, including soups, sauces, gravies, desserts, and processed foods. Starch helps improve texture, consistency, and mouthfeel in these applications, enhancing the sensory experience and shelf stability of the products.
Starch Applications
Starch finds a wide range of applications across various industries and in everyday use. Here are some key applications of starch:
- Paper Industry: Starch is extensively used in the paper industry. It is employed for paper strengthening, improving the surface properties of paper, and enhancing printability. Starch-based coatings and sizing agents are applied to paper to increase its strength, reduce ink penetration, and improve the surface for better printing and writing.
- Brewing Industry: Starch plays a vital role in the brewing process. During the production of beer, starch is converted into fermentable sugars through the enzymatic action of amylases. These sugars are then fermented by yeast to produce alcohol and carbon dioxide. Starch from malted grains, such as barley, provides the source of fermentable sugars necessary for brewing.
- Culinary Uses: Starch is widely used in kitchens for various culinary purposes. It serves as a thickening agent in sauces, gravies, soups, and custards. When heated in the presence of liquid, starch granules absorb moisture, swell, and gelatinize, giving the desired thick consistency to the food preparations. Starch is also used as a coating for frying, providing a crispy texture to fried foods.
- Paper and Packaging: Starch is a key component in the manufacture of paper products and packaging materials. It is used to produce paperboard, paper bags, paper boxes, gummed paper, and adhesive tapes. Starch-based adhesives are employed for bonding paper fibers and as a coating for improving the strength and surface properties of paper products.
- Industrial Applications: Starch finds applications beyond the food and paper industries. For example, corn starch is used as a lubricant in the manufacturing of surgical gloves. The starch coating on gloves prevents them from sticking together, facilitating their use in medical and healthcare settings.
- Other Uses: Starch is also utilized in various other applications. It can be found in pharmaceuticals as an excipient or filler in tablets and capsules. Additionally, starch-based biodegradable materials are being explored as sustainable alternatives in packaging, disposable cutlery, and other single-use products.
4. Xylan: Properties, Functions and Applications
- Xylan is a polysaccharide that consists of repeating units of xylose, a pentose sugar. These xylose units are connected by beta-1,4-linkages. It is a significant component found in the cell walls of plants, making it the third most abundant polymer on Earth.
- In various plant species, xylans are present in significant quantities. They account for approximately 25-30% of the dry biomass of woody tissues in dicots and lignified tissues in monocots. In certain cereals and grasses, xylans can constitute up to 50% of their overall weight.
- The structure of xylan, characterized by its beta-1,4-linkages, provides it with unique properties. Due to its complex branched structure, xylan contributes to the stability and integrity of plant cell walls. It forms a matrix with other cell wall components like cellulose and lignin, providing structural support and strength to plant tissues.
- Xylan also plays a crucial role in plant growth and development. It is involved in processes such as cell elongation, differentiation, and secondary cell wall formation. Moreover, xylans are known to participate in plant defense mechanisms against pathogens and environmental stress.
- Beyond its natural occurrence in plants, xylan has gained attention for its potential applications. It can be extracted and utilized for various purposes in different industries. For example, xylan can be processed to obtain xylooligosaccharides, which have prebiotic properties and find applications in the food and pharmaceutical industries.
- In the field of biofuels and renewable energy, xylan is of particular interest. It can be enzymatically hydrolyzed to release xylose, which can then be fermented to produce bioethanol or other value-added chemicals. The abundant availability of xylan-rich biomass, such as agricultural residues and wood, makes it a promising feedstock for sustainable biofuel production.
- In summary, xylan is a polysaccharide consisting of xylose units connected by beta-1,4-linkages. It is a prominent component of plant cell walls, accounting for a significant portion of the biomass in various plant tissues. Xylan contributes to the structural integrity of plants and is involved in growth and defense mechanisms. Its potential applications range from prebiotics in food and pharmaceuticals to renewable energy production through biofuel processes.
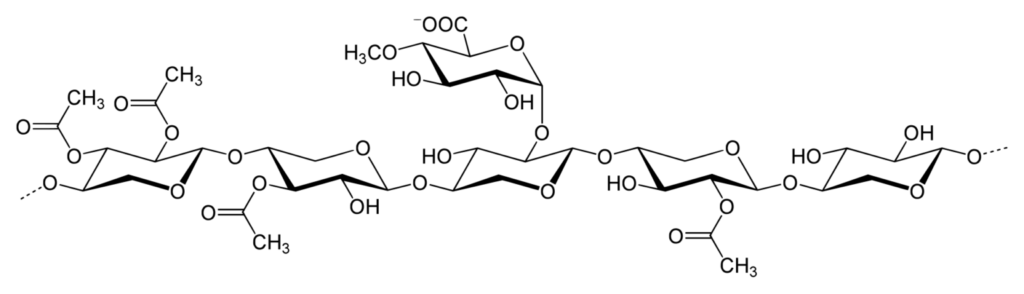
Xylan Properties
Xylan possesses several properties that contribute to its unique characteristics and interactions with other molecules:
- Hydrolysis: Xylans can undergo hydrolysis through acidic or enzymatic processes. Acidic hydrolysis or the action of xylanases, enzymes that specifically break down xylan, can convert xylan into smaller units called xylooligosaccharides. These xylooligosaccharides have various applications in the food, pharmaceutical, and biotechnology industries.
- Interactions with polysaccharides: Xylans have the ability to interact with other polysaccharides present in plant cell walls through non-covalent interactions, such as hydrogen bonding. These interactions contribute to the overall structural integrity of the cell wall and influence its mechanical properties.
- Interactions with lignin and phenolic compounds: Xylans can also interact with lignins and other phenolic compounds present in plant cell walls. Unlike the non-covalent interactions with polysaccharides, the interaction between xylans and lignin involves covalent bonding. This interaction plays a crucial role in the formation of lignocellulosic complexes, which contribute to the rigidity and strength of plant cell walls.
- Solubility: Xylans exhibit varying solubility depending on their structure and environmental conditions. Some xylans are soluble in water, while others may require specific solvents or treatment to enhance their solubility. The solubility of xylans is important for their extraction and utilization in various industrial processes.
- Branched structure: Xylans possess a branched structure, which contributes to their versatility and functionality. The branching pattern can vary, affecting the overall properties and interactions of xylans with other molecules.
- Molecular weight: Xylans can exhibit a wide range of molecular weights, which can influence their behavior and functional properties. The molecular weight of xylans can be modified through various processing techniques, such as enzymatic hydrolysis or chemical modifications, to obtain desired characteristics for specific applications.
Xylan Functions
Xylans play important functional roles in plant cell walls, contributing to various biological processes and defense mechanisms. Here are the key functions of xylan:
- Structural integrity: Xylans provide rigidity and strength to the plant’s cell wall. As a major component of the hemicellulose fraction, xylans contribute to the overall architecture and stability of the cell wall. The crosslinking between xylans, cellulose, and lignin helps create a complex and robust network, providing mechanical support and protection to plant tissues.
- Recalcitrance to enzymatic digestion: Xylans contribute to the recalcitrant nature of plant cell walls, making them resistant to enzymatic digestion. The complex structure and interactions of xylans with other cell wall components, such as lignin, hinder the accessibility of enzymes, thereby reducing the efficiency of degradation by microbial or enzymatic systems.
- Defense against herbivores: Xylans play a role in plant defense against herbivores. When plants are attacked by herbivorous insects, xylans can be released from the cell wall, leading to the formation of physical barriers that restrict the movement and feeding of herbivores. This defense mechanism helps protect the plant from excessive damage caused by herbivory.
- Protection against pathogenic attacks: Xylans also contribute to the plant’s defense against pathogenic attacks. When plants are exposed to pathogens, the synthesis and modification of xylans can be induced, leading to structural changes in the cell wall. These changes can enhance the physical barriers and provide additional protection against pathogen invasion.
- Regulation of plant growth and development: Xylans are involved in regulating plant growth and development processes. They play a role in cell expansion, cell wall deposition, and cell signaling pathways, influencing various aspects of plant growth, including stem elongation, tissue differentiation, and organ development.
Xylan Applications
Xylan finds application in various industries due to its unique properties and conversion products. Here are some notable applications of xylan:
- Food industry: Xylan plays a significant role in the bread industry. When added to bread dough, xylan enhances the quality and toughness of the final product. It improves the texture, moisture retention, and shelf life of bread, making it more appealing to consumers.
- Xylitol production: Xylan is a valuable source of xylitol, a sugar substitute widely used in the food and pharmaceutical industries. Xylitol has a similar sweetness to sugar but with a lower caloric content and a lower impact on blood sugar levels. It is commonly used as a sugar substitute in various products, including chewing gum, candies, baked goods, and oral care products. Xylitol is particularly beneficial for diabetic patients as it does not raise blood sugar levels.
- Biorefinery and biofuel production: Xylan can be extracted from lignocellulosic biomass, such as agricultural residues and wood waste, as part of the biorefinery process. Xylan-rich biomass can be hydrolyzed and fermented to produce biofuels, such as ethanol and other valuable chemicals. This utilization of xylan contributes to the development of sustainable and renewable energy sources.
- Biocompatible materials: Xylan and its derivatives have potential applications in the development of biocompatible materials. Xylan can be modified to improve its properties, such as solubility, compatibility, and stability, making it suitable for various applications in biomedicine, drug delivery systems, and tissue engineering.
- Agricultural and horticultural applications: Xylan-based products are used in agriculture and horticulture as soil amendments and plant growth enhancers. Xylan-based materials can improve soil structure, water retention, and nutrient availability, leading to enhanced crop productivity and plant health.
Heteropolysaccharides
Heteropolysaccharides are a type of polysaccharide composed of two or more different types of monomer units. Unlike homopolysaccharides, which consist of repeating units of the same monomer, heteropolysaccharides exhibit structural diversity due to the presence of multiple monomer types. These polysaccharides play important roles in various biological systems. Here are some key points about heteropolysaccharides:
- Composition: Heteropolysaccharides are characterized by the presence of different monomer units in their structure. These monomers can vary in terms of their sugar type, arrangement, and linkage. The combination of different monomers gives heteropolysaccharides distinct properties and functions.
- Examples: Some common examples of heteropolysaccharides include glycosaminoglycans (such as hyaluronic acid, chondroitin sulfate, and heparin), agarose (found in seaweeds), and peptidoglycans (a major component of bacterial cell walls). These heteropolysaccharides have diverse functions in biological systems.
- Association with other biomolecules: Heteropolysaccharides are often associated with peptides, proteins, or lipids. These associations can provide structural stability, influence solubility, and contribute to the overall function of the biomolecule complex. For example, glycosaminoglycans are commonly found attached to core proteins, forming proteoglycans that play crucial roles in connective tissues and extracellular matrix.
- Biological functions: Heteropolysaccharides exhibit a wide range of biological functions. They can contribute to cell adhesion, cell signaling, lubrication, and tissue development. For instance, glycosaminoglycans are involved in maintaining the structural integrity of tissues, regulating cell behavior, and modulating inflammatory responses.
- Medical and industrial applications: Heteropolysaccharides have important applications in medicine and industry. They are used as therapeutic agents, drug delivery systems, wound dressings, and tissue engineering scaffolds. In the food industry, heteropolysaccharides are utilized as gelling agents, stabilizers, and thickeners.
- Structural diversity: The presence of multiple monomer units in heteropolysaccharides allows for structural diversity and versatility. The arrangement and linkage of different monomers give rise to complex three-dimensional structures, leading to a wide range of physical and chemical properties.
1. Glycosaminoglycans (GAGs): Properties, Functions and Applications
Glycosaminoglycans (GAGs) are a group of negatively charged unbranched heteropolysaccharides found in animals and bacteria. They are composed of repeating units of disaccharides that consist of acidic and amino sugars. GAGs play essential roles in various biological processes, particularly in the extracellular matrix and cell signaling.
The structure of GAGs follows a general formula, represented as (GAG)n, where “n” indicates the variable number of repeating units. One of the characteristic features of GAGs is their negative charge, which is primarily conferred by the presence of acidic sugars, usually in the form of uronic acids such as glucuronic acid or L-iduronic acid. The amino sugar component in GAGs can be either N-acetylglucosamine or N-acetylgalactosamine.
Several major glycosaminoglycans are known, each with distinct compositions and functions. Here are some examples along with their corresponding acidic and amino sugars:
- Hyaluronic acid:
- Acidic sugar: D-Glucuronic acid
- Amino sugar: N-acetylglucosamine
- Chondroitin sulfate:
- Acidic sugar: D-Glucuronic acid
- Amino sugar: N-acetylgalactosamine
- Heparan sulfate:
- Acidic sugar: D-Glucuronic acid or L-iduronic acid
- Amino sugar: N-acetylglucosamine
- Heparin:
- Acidic sugar: D-Glucuronic acid or L-iduronic acid
- Amino sugar: N-acetylglucosamine
- Dermatan sulfate:
- Acidic sugar: D-Glucuronic acid or L-iduronic acid
- Amino sugar: N-acetylgalactosamine
- Keratan sulfate:
- Acidic sugar: D-Galactose
- Amino sugar: N-acetylglucosamine
These GAGs differ in their structural arrangement, length, and specific sulfation patterns, contributing to their unique functions within the body. For example, hyaluronic acid provides viscosity and lubrication in synovial fluid and plays a role in tissue hydration. Chondroitin sulfate and keratan sulfate are major components of cartilage, contributing to its resilience and shock-absorbing properties.
Glycosaminoglycans participate in various physiological processes, including cell adhesion, cellular signaling, and tissue development. They interact with proteins, forming proteoglycans, which are important components of the extracellular matrix. GAGs also serve as binding sites for growth factors, cytokines, and enzymes, regulating their activity and availability.
The study of glycosaminoglycans is crucial for understanding their roles in health and disease. Imbalances or abnormalities in GAG metabolism can lead to pathological conditions, such as osteoarthritis, cardiovascular diseases, and certain genetic disorders. Researchers continue to investigate GAGs and their potential therapeutic applications, aiming to develop treatments targeting these intricate carbohydrate molecules.
In summary, glycosaminoglycans are negatively charged heteropolysaccharides composed of repeating disaccharide units. They are essential components of the extracellular matrix and play diverse roles in cellular processes. Understanding the structure and function of GAGs provides valuable insights into their contributions to biological systems and opens avenues for future research and medical interventions.
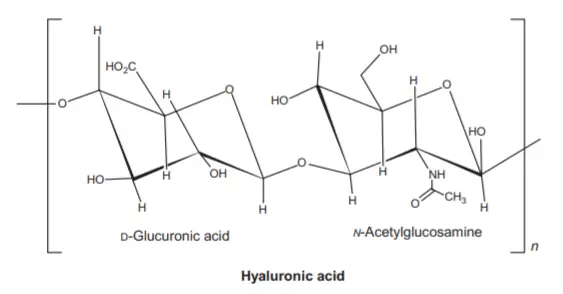
Glycosaminoglycans (GAGs) Properties
Glycosaminoglycans (GAGs) possess unique properties that contribute to their diverse functions in biological systems. Here are some key properties of GAGs:
- Composition: GAGs are composed of amino sugars (N-acetylglucosamine or N-acetylgalactosamine) and uronic acids (such as glucuronic acid or L-iduronic acid). These monosaccharides form repeating disaccharide units, which make up the backbone of GAG molecules.
- Sulfation: With the exception of hyaluronic acid, all GAGs are sulfated. Sulfate groups are covalently attached to the amino or hydroxyl groups within GAG structures. Sulfation occurs as O-esters or N-sulfates, enhancing the overall negative charge of GAGs.
- Proteoglycans: GAGs, apart from hyaluronic acid, are covalently attached to proteins, forming proteoglycans. These proteins provide a scaffold for GAGs and play a crucial role in their organization and function. The combination of GAGs and proteins in proteoglycans contributes to the diverse functions of these molecules.
- Negative Charge: The presence of sulfate groups on the surface of GAGs imparts a negative charge to the molecules. This negative charge is responsible for the high density of negative charges in the extracellular matrix. The negative charges create an osmotic pressure that attracts and retains water molecules. As a result, tissues containing GAGs can imbibe water and swell, enhancing their ability to bear load and providing resistance against compression.
The property of water retention and tissue swelling is particularly important for GAG-rich tissues like cartilage, where it allows for shock absorption and maintenance of tissue hydration. This property also contributes to the viscoelastic properties of synovial fluid in joints, providing lubrication and reducing friction during movement.
Overall, the composition, sulfation, association with proteins, and negative charge of GAGs are crucial for their roles in maintaining tissue integrity, providing mechanical strength, and regulating cellular processes. The properties of GAGs make them important components of the extracellular matrix and essential contributors to various physiological functions in the body.
Glycosaminoglycans (GAGs) Functions
Glycosaminoglycans (GAGs) exhibit diverse functions in various biological processes. Here are some key functions of specific GAGs:
- Hyaluronic acid: Hyaluronic acid is a vital component of the vitreous humor in the eye and synovial fluid, which acts as a lubricant in joints. It provides viscosity and lubrication, allowing for smooth joint movement. Additionally, hyaluronic acid plays a role in developmental processes such as tumor metastasis, angiogenesis (formation of new blood vessels), and blood coagulation.
- Heparin: Heparin functions as a natural anticoagulant by inhibiting blood clotting. It prevents the formation of blood clots by interfering with the coagulation cascade, making it essential in medical applications to prevent excessive clotting during surgeries or in the treatment of thrombotic disorders.
- Keratan sulfate: Keratan sulfate is found in tissues such as the cornea, cartilage, and bones. In joints, it acts as a cushion, absorbing mechanical stresses and providing structural support. Keratan sulfate contributes to the resilience and shock-absorbing properties of these tissues, ensuring their durability and integrity.
- Chondroitin: Chondroitin is a crucial component of cartilage, playing a significant role in providing compression resistance. It helps maintain the structural integrity of cartilage and provides elasticity, allowing it to withstand mechanical forces and absorb impact. Chondroitin is commonly used as a dietary supplement for joint health due to its potential benefits in maintaining cartilage function.
- Dermatan sulfate: Dermatan sulfate has diverse functions in various physiological processes. It is involved in wound repair, where it modulates the proliferation and migration of cells involved in tissue regeneration. Dermatan sulfate also plays a role in blood coagulation regulation, interacting with clotting factors. Additionally, it contributes to immune responses and has implications in cardiovascular disorders.
These examples highlight the functional diversity of GAGs in different tissues and processes throughout the body. The specific composition and structure of each GAG contribute to its unique biological functions. Understanding the functions of GAGs is crucial for comprehending their roles in health and disease and can lead to potential therapeutic applications in various medical fields.
Glycosaminoglycans (GAGs) Applications
Glycosaminoglycans (GAGs) have various applications in the field of medicine and research. Here are some notable applications of GAGs:
- Chondroitin sulfate: Chondroitin sulfate is commonly used as a dietary supplement in alternative medicine to treat osteoarthritis. It is believed to help reduce joint pain and improve joint function in individuals with this condition. Chondroitin sulfate supplements are often used in combination with other supplements like glucosamine. Research is ongoing to explore its effectiveness and potential benefits.
- Heparin: Heparin is a widely used medication in the treatment of venous thromboembolism (VTE), a condition characterized by the formation of blood clots in veins. It is also used in atherothrombotic syndromes, which involve the formation of blood clots in arteries. Heparin acts as an anticoagulant, preventing the formation and growth of blood clots. It is administered via injection and is commonly used in both acute and long-term treatment.
- Hyaluronic acid: Hyaluronic acid has shown promise in various applications. In research using mouse models of cystic fibrosis, it has been demonstrated to decrease inflammation associated with the condition. This suggests its potential as a therapeutic agent in managing cystic fibrosis-related inflammation. Hyaluronic acid is also widely used in cosmetic and dermatological procedures, such as dermal fillers and skincare products, due to its ability to retain moisture and improve skin hydration.
- Antitumor therapeutics: Both chondroitin sulfate and heparin have been investigated for their potential application in antitumor treatments. Studies have explored their ability to inhibit tumor growth, metastasis, and angiogenesis. Chondroitin sulfate and heparin have demonstrated promising results in preclinical studies, suggesting their potential as components of future antitumor therapies. However, further research and clinical trials are required to establish their efficacy and safety in cancer treatment.
The applications of GAGs extend beyond the examples mentioned above. Ongoing research aims to uncover additional therapeutic uses of GAGs, such as tissue engineering, wound healing, and regenerative medicine. GAGs’ diverse properties, including their ability to modulate cellular interactions, make them attractive candidates for various biomedical applications.
It’s important to note that while GAGs have shown potential in certain applications, their specific use and effectiveness should be evaluated under appropriate medical guidance and based on individual circumstances.
2. Peptidoglycans: Properties, Functions and Applications
Peptidoglycans are an essential component of bacterial cell walls, providing structural integrity and protection. Here are some key characteristics and functions of peptidoglycans:
- Composition: Peptidoglycans are composed of a heteropolymer made up of alternating units of N-acetylglucosamine (NAG) and N-acetylmuramic acid (NAM). These units are linked together by beta-1,4-glycosidic bonds, forming long chains.
- Gram-positive and gram-negative bacteria: Peptidoglycans play a significant role in the structural differences between gram-positive and gram-negative bacteria. In gram-positive bacteria, peptidoglycans make up a substantial portion of the cell wall, comprising 40-90% of the dry weight. In contrast, in gram-negative bacteria, peptidoglycans account for only about 10% of the dry weight of the cell wall. Gram-negative bacteria have an additional outer membrane composed of lipopolysaccharides, which contributes to the overall structure of their cell wall.
- Structural integrity: The main function of peptidoglycans is to provide strength and rigidity to the bacterial cell wall. The alternating NAG-NAM units form a mesh-like network that surrounds the cell, creating a protective barrier against osmotic pressure and maintaining the shape of the bacterium. Peptidoglycans contribute to the overall stability of the cell and protect it from mechanical stresses.
- Antibiotic target: Peptidoglycans are a crucial target for many antibiotics. Antibiotics such as beta-lactams (e.g., penicillin) and glycopeptides (e.g., vancomycin) inhibit the synthesis or proper functioning of peptidoglycans. These antibiotics interfere with the enzymes involved in peptidoglycan synthesis, leading to cell wall weakening and bacterial cell lysis. Understanding the structure and function of peptidoglycans has been instrumental in the development of antibiotics to combat bacterial infections.
- Immunogenicity: Peptidoglycans can trigger an immune response in the host organism. The recognition of peptidoglycan fragments by the innate immune system, such as pattern recognition receptors (PRRs), can activate immune cells and initiate the inflammatory response. This immune response plays a crucial role in defending against bacterial infections and shaping the overall immune response to bacterial pathogens.
Peptidoglycans are fundamental components of bacterial cell walls, conferring strength, protection, and immunogenic properties. Their unique structure and composition make them important targets for antibiotics and contribute to the overall understanding of bacterial physiology and host-pathogen interactions.
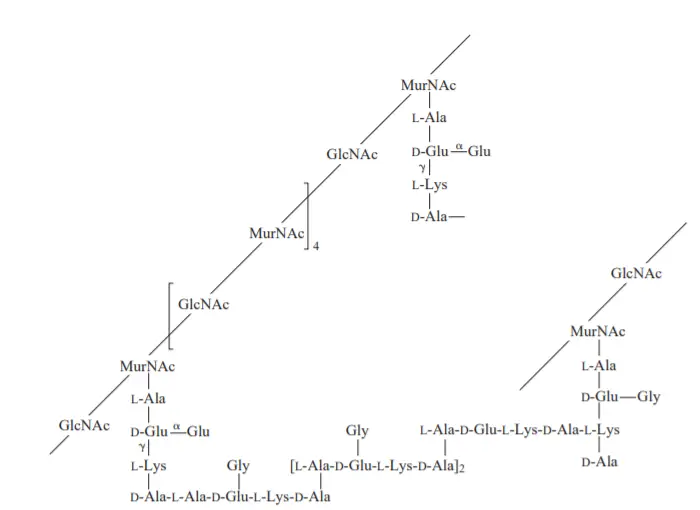
Peptidoglycans Properties
Peptidoglycans possess several notable properties that contribute to their structure and vulnerability to degradation. Here are some key properties of peptidoglycans:
- Susceptibility to lysozyme: Peptidoglycans are susceptible to degradation by the enzyme lysozyme. Lysozyme catalyzes the hydrolysis of the beta-1,4-linkage between N-acetylglucosamine (NAG) and N-acetylmuramic acid (NAM) units, leading to the breakdown of peptidoglycan chains. This enzymatic degradation weakens the bacterial cell wall and can result in cell lysis.
- Sensitivity to penicillin: Penicillin and other beta-lactam antibiotics interfere with the synthesis of peptidoglycans. They target enzymes involved in peptidoglycan biosynthesis, such as penicillin-binding proteins (PBPs), which are responsible for cross-linking the peptidoglycan chains. By inhibiting these enzymes, penicillin disrupts the proper assembly of peptidoglycan, leading to cell wall defects and bacterial cell death.
- Role of N-acetylmuramyl-L-alanine amidases: N-Acetylmuramyl-L-alanine amidases are enzymes that hydrolyze the peptidoglycan structure by breaking down the amide bond between N-acetylmuramic acid and L-alanine. This enzymatic activity leads to the dissolution of peptidoglycan and contributes to the remodeling and turnover of the cell wall during bacterial growth and division.
- Hydrolysis by lysozyme: Lysozyme, an enzyme found in various body fluids and secretions, including tears and saliva, targets the beta-1,4-linkage between NAG and NAM units in peptidoglycans. This hydrolytic activity of lysozyme can weaken the cell wall of bacteria, making them more susceptible to immune responses and antimicrobial agents.
Understanding the properties of peptidoglycans, such as their vulnerability to degradation by lysozyme and susceptibility to antibiotics like penicillin, has been instrumental in the development of antibacterial strategies. Targeting peptidoglycan synthesis and integrity is a common approach for combating bacterial infections and controlling bacterial growth.
Peptidoglycans Functions
Peptidoglycans play crucial roles in the structure and function of bacterial cells. Here are the main functions of peptidoglycans:
- Structural integrity: Peptidoglycans are a vital component of bacterial cell walls, providing strength and rigidity. The cross-linked network of peptidoglycan chains forms a protective layer surrounding the bacterial cell. This structural integrity is essential for maintaining the shape of the cell and protecting it from mechanical stresses.
- Binary fission: Peptidoglycans are involved in the process of binary fission, which is how bacteria reproduce. During binary fission, a bacterial cell divides into two daughter cells. Peptidoglycan synthesis and remodeling are crucial for the expansion and separation of the cell wall during this reproductive process. The coordinated synthesis and modification of peptidoglycan allow the bacterial cell to divide and generate two genetically identical daughter cells.
- Osmotic regulation: Peptidoglycans play a role in preventing bacterial cells from bursting due to the osmotic pressure difference between the cell’s cytoplasm and the external environment. The peptidoglycan layer acts as a protective barrier, counteracting the inward osmotic pressure exerted by the cytoplasm. This prevents the cell from swelling and bursting when exposed to hypotonic conditions. Peptidoglycans contribute to maintaining the osmotic balance and stability of bacterial cells.
- Antibiotic target: The unique composition and structure of peptidoglycans make them a target for antibiotics. Antibiotics like penicillin and other beta-lactams interfere with the synthesis or proper functioning of peptidoglycans, leading to cell wall defects and bacterial cell death. Targeting peptidoglycan biosynthesis is a key strategy in antibiotic therapy to control bacterial infections.
By fulfilling these essential functions, peptidoglycans contribute to the overall physiology, survival, and reproduction of bacteria. The understanding of peptidoglycan’s functions has not only advanced our knowledge of bacterial biology but also facilitated the development of antimicrobial strategies to combat bacterial infections.
Peptidoglycans Applications
Peptidoglycans have significant applications in the field of microbiology and clinical diagnostics. Here is a key application of peptidoglycans:
- Bacterial identification: The presence of peptidoglycans in the cell walls of bacteria serves as a useful tool for bacterial identification, specifically in distinguishing between gram-positive and gram-negative bacteria. The Gram staining technique, developed by Hans Christian Gram, is widely used to differentiate bacterial species based on their cell wall composition. When subjected to the Gram stain, gram-positive bacteria retain the purple crystal violet dye due to their thick layer of peptidoglycan, while gram-negative bacteria lose the purple stain and take up the counterstain, appearing pink or red. This differentiation is essential in clinical diagnostics to guide treatment decisions and determine appropriate antibiotic regimens.
By utilizing the presence or absence of peptidoglycans in the bacterial cell wall, clinicians and microbiologists can quickly identify the nature of the bacteria, whether it is gram-positive or gram-negative. This information plays a crucial role in guiding treatment strategies and determining the appropriate course of action in combating bacterial infections.
Peptidoglycans, as a diagnostic tool, aid in the accurate classification and identification of bacteria, facilitating targeted therapeutic approaches and improved patient outcomes in clinical practice.
3. Agarose: Properties, Functions and Applications
Agarose is a polysaccharide that is widely used in various scientific and biomedical applications. Here are some key points about agarose:
- Composition: Agarose is composed of repeating units of agarobiose, which is a disaccharide made up of D-galactose and 3,6-anhydro-L-galactopyranose. These repeating units are linked together to form a linear polymer.
- Source: Agarose is primarily extracted from red seaweed (Rhodophyta), which is abundant in marine environments. The seaweed is harvested and processed to obtain agarose, making it a natural and renewable resource.
- Gel formation: One of the remarkable properties of agarose is its ability to form gels. Agarose molecules can associate with each other and form a three-dimensional network through hydrogen bonding. This gel formation occurs when agarose is dissolved in a hot aqueous solution and then cooled, allowing the polymer chains to reassociate and entrap water molecules within the gel structure.
- Gel strength and porosity: The gel strength and porosity of agarose can be adjusted by varying the concentration of agarose in the gel solution. Higher agarose concentrations result in stronger gels with smaller pore sizes, while lower concentrations yield weaker gels with larger pores. This tunable property makes agarose suitable for various applications where different gel properties are required.
In summary, agarose is a polysaccharide derived from red seaweed, composed of repeating units of agarobiose. Its ability to form gels with adjustable properties makes it a valuable tool in various scientific and biomedical applications, particularly in molecular biology and biochemistry.
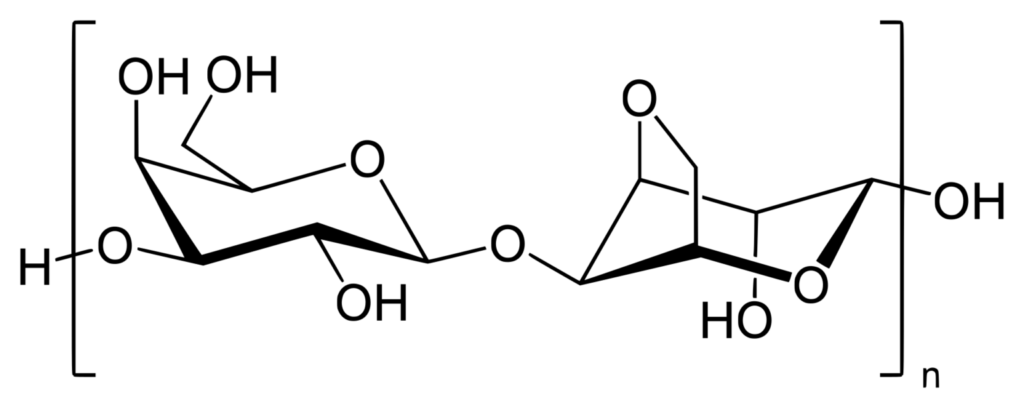
Agarose Properties
Agarose exhibits specific properties that make it suitable for various applications. Here are some key properties of agarose:
- Molecular weight: The average molecular weight of a polymer chain of agarose is approximately 120,000. This high molecular weight contributes to the formation of a stable gel structure.
- Physical state: Agarose is commonly found in the form of a white powder. It is insoluble in cold water but readily dissolves in hot water, forming a clear solution.
- Gel formation: Agarose demonstrates a unique property of gel formation. When the hot agarose solution is cooled down, it undergoes gelation due to the reassociation of agarose molecules. The three-dimensional gel structure is primarily held together by hydrogen bonding between the polymer chains.
- Melting and gelling temperature: The melting and gelling temperature of agarose vary depending on the source and type of agarose. Agarose extracted from Gelidium typically exhibits a gelling temperature range of 34–38°C (93–100°F) and a melting temperature range of 90–95°C (194–203°F). On the other hand, agarose extracted from Gracilaria typically has a higher gelling temperature range of 40–52°C (104–126°F) and a lower melting temperature range of 85–90°C (185–194°F).
- Pore size and resolution: Agarose gels can be customized to have different pore sizes and resolutions by adjusting the concentration of agarose in the gel solution. Higher agarose concentrations result in gels with smaller pore sizes, allowing better separation and resolution of biomolecules during gel electrophoresis.
These properties make agarose a versatile material for applications such as gel electrophoresis, DNA and protein separation, purification of biomolecules, and immobilization of enzymes or cells. The ability to control the gel properties, combined with its ease of use and availability, has made agarose an indispensable tool in various scientific and biomedical research fields.
Agarose Functions
Agarose serves important functions in both the natural environment and scientific applications. Here is a key function of agarose:
- Supporting structure in marine algae: Agarose plays a vital role in providing a supporting structure in the cell walls of marine algae, particularly in the Rhodophyta (red algae) phylum. Within the cell wall, agarose molecules form a complex network that contributes to the overall strength and integrity of the algal cells.
In marine algae, agarose is synthesized and deposited in the cell wall, helping to maintain the structural integrity of the organisms. The presence of agarose in the cell wall provides mechanical support and protection against external factors such as water currents, wave action, and predation. It helps the algae maintain their shape and withstand the physical stresses of their marine environment.
Additionally, the gel-like nature of agarose in the cell wall aids in water retention, preventing excessive dehydration and maintaining the optimal hydration levels required for the survival and functioning of marine algae.
While the primary function of agarose in marine algae is related to their structural support, it is also the basis for the extraction of agarose for various scientific and industrial applications. The unique properties of agarose, including its ability to form gels and its biocompatibility, make it valuable in a range of laboratory techniques, biotechnology processes, and biomedical applications.
In summary, agarose functions as a supporting structure in the cell walls of marine algae, contributing to their overall strength and integrity. This function is essential for the survival and adaptation of marine algae in their natural habitat.
Structural polysaccharides
- Structural polysaccharides are a type of carbohydrate that serve as building blocks and provide structural support to organisms. Unlike storage polysaccharides, which are used for energy storage, structural polysaccharides are involved in forming and maintaining the physical structures of cells, tissues, and organisms.
- These polysaccharides are typically composed of long chains of sugar molecules, which are linked together in various patterns and configurations. The arrangement and bonding between these sugar units give structural polysaccharides their unique properties and functions.
- Structural polysaccharides are found in a variety of organisms, including plants, animals, and microorganisms. They are essential for maintaining the integrity and strength of cell walls, extracellular matrices, exoskeletons, and other structural components.
- Examples of structural polysaccharides include cellulose, chitin, arabinoxylans, and pectins. Cellulose is the main component of plant cell walls, providing rigidity and support to plant cells. Chitin is a major component of the exoskeletons of arthropods and the cell walls of fungi, contributing to their structural integrity. Arabinoxylans and pectins are also important components of plant cell walls, aiding in cell adhesion, elasticity, and flexibility.
- Overall, structural polysaccharides play a crucial role in maintaining the physical structure and form of organisms. They provide support, protection, and functional properties that are essential for their survival and proper functioning.
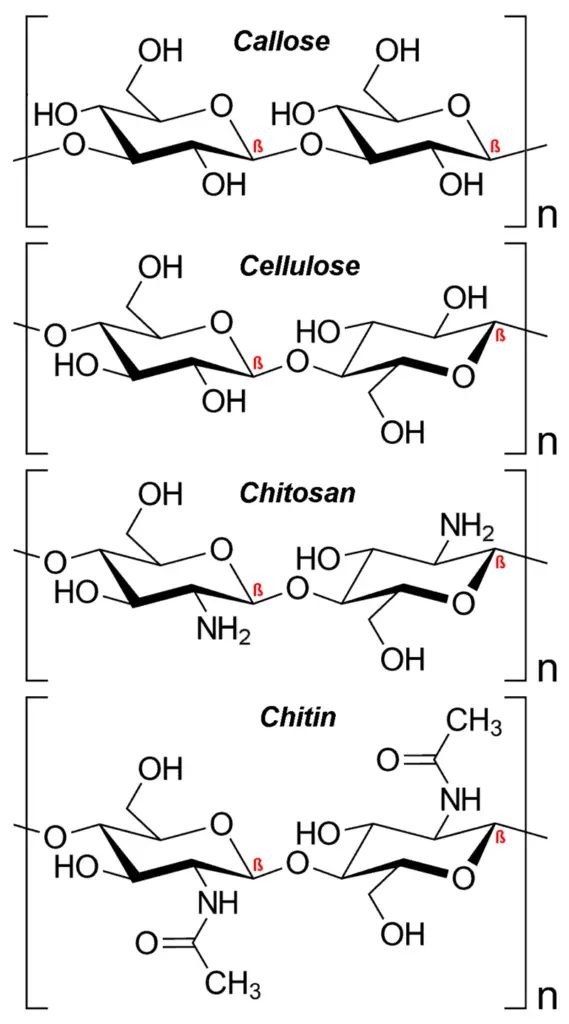
Examples of Structural polysaccharides
Structural polysaccharides play vital roles in providing support, strength, and protection in various biological structures. Here are some examples of structural polysaccharides:
- Arabinoxylans: Arabinoxylans are copolymers of arabinose and xylose sugars. They are present in both the primary and secondary cell walls of plants. Arabinoxylans contribute to the structural integrity of plant tissues and may also have beneficial effects on human health.
- Cellulose: Cellulose is a major component of plant cell walls. It is a polymer consisting of repeated glucose units bonded together by beta-linkages. Cellulose provides rigidity and strength to plant structures. Wood, paper, and cotton are examples of materials rich in cellulose. Humans and many animals lack the enzyme required to break down cellulose, making it indigestible for them.
- Chitin: Chitin is a structural polysaccharide found in the exoskeletons of arthropods, such as insects and crustaceans, as well as in the cell walls of fungi. It is composed of long chains of N-acetylglucosamine units. Chitin provides rigidity and support to these organisms’ structures. It is biodegradable and can be broken down by enzymes called chitinases, which are produced by microorganisms and some plants.
- Chitosan: Chitosan is a derivative of chitin and is more water-soluble. It shares a similar chemical structure with chitin and exhibits similar structural properties. Chitosan has applications in various fields, including biomedicine, agriculture, and environmental sciences.
- Pectins: Pectins are complex polysaccharides composed of 1,4-linked α-D-galactosyl uronic acid residues. They are present in the primary cell walls and nonwoody parts of terrestrial plants. Pectins contribute to the strength and flexibility of plant tissues and are involved in cell adhesion and signaling processes.
These examples highlight the diversity of structural polysaccharides and their crucial roles in providing support, strength, and protection in biological systems. From plant cell walls to insect exoskeletons, these polysaccharides contribute to the structural integrity and functionality of various organisms and materials.
Storage polysaccharides
Storage polysaccharides are carbohydrates that serve as a form of energy storage in organisms. They are synthesized and accumulated when the organism has excess glucose or other simple sugars available, and they can be later broken down and used as a source of energy when needed. Storage polysaccharides are typically large, insoluble molecules that can be stored within cells or tissues.
Examples of Storage polysaccharides
Examples of storage polysaccharides include:
- Starch: Starch is a glucose polymer composed of both amylose and amylopectin. It serves as the primary storage polysaccharide in plants. Amylose is a linear chain of glucose molecules, while amylopectin is a highly branched molecule. Starch is insoluble in water and can be found in sources like potatoes, rice, wheat, and maize. It is a major source of dietary carbohydrates for humans and can be broken down into glucose through digestion.
- Glycogen: Glycogen is the storage polysaccharide in animals and some microorganisms. It is structurally similar to amylopectin but is more extensively branched. Glycogen is primarily synthesized and stored in the liver and muscles. It serves as a readily accessible source of glucose for energy production during periods of fasting or increased energy demand. Glycogen can be broken down into glucose through glycogenolysis.
- Galactogen: Galactogen is a polysaccharide composed of galactose. It functions as an energy storage polysaccharide in pulmonate snails and some Caenogastropoda. It is found in the albumen gland of female snails and in the perivitelline fluid of eggs. Galactogen serves as an energy reserve for developing embryos and hatchlings.
- Inulin: Inulin is a naturally occurring polysaccharide composed of fructose units. It belongs to a class of dietary fibers known as fructans. Inulin is found in various plants and is used as a means of storing energy. It is typically present in roots or rhizomes. Human digestive enzymes cannot completely break down inulin, so it functions as a dietary fiber. Inulin has been approved by the FDA as a dietary fiber ingredient.
These storage polysaccharides provide organisms with a means of storing excess glucose or other sugars in a form that can be readily mobilized and used as an energy source when needed.
What is Acidic polysaccharides?
- Acidic polysaccharides are a specific type of polysaccharide that contain carboxyl groups, phosphate groups, and/or sulfuric ester groups. These additional chemical groups contribute to the acidic properties of these polysaccharides.
- Polysaccharides that contain sulfate groups can be found in algae or obtained through chemical modification processes. Sulfated polysaccharides extracted from algae are known for their various biological activities and potential therapeutic applications.
- Polysaccharides, in general, are important biomolecules that serve a variety of functions in living organisms. They are composed of long chains of carbohydrate molecules, which are made up of smaller units called monosaccharides. Polysaccharides can be classified as either homopolysaccharides, consisting of a single type of monosaccharide, or heteropolysaccharides, composed of different types of monosaccharides.
- These polysaccharides can exist in different structural forms. They can be linear, with the monosaccharide units arranged in a straight chain, or they can be branched, with side chains extending from the main chain. The branching or linear arrangement of monosaccharide units determines the overall structure and function of the polysaccharide.
- Acidic polysaccharides, with their carboxyl, phosphate, or sulfate groups, possess unique properties and functionalities. These additional groups contribute to their acidic nature and can influence their interactions with other molecules, such as proteins or ions. The presence of acidic groups can also affect the solubility, stability, and biological activities of these polysaccharides.
- Overall, acidic polysaccharides represent a specific subset of polysaccharides that possess acidic functional groups. Their unique chemical composition gives them distinctive properties and allows them to play important roles in various biological processes.
Agarose Applications
Agarose finds a wide range of applications in laboratory settings due to its unique properties. Here are some key applications of agarose:
- DNA separation: Agarose gel electrophoresis is a commonly used technique in molecular biology laboratories for the separation and analysis of DNA molecules. Agarose is used to create a gel matrix with pores of specific sizes. When an electric current is applied, DNA molecules migrate through the gel based on their size, allowing researchers to separate and visualize different DNA fragments. The lower degree of complexity of agarose compared to other gelling agents makes it less likely to interact with DNA, ensuring accurate separation.
- Protein separation: Agarose gel electrophoresis can also be employed for the separation of proteins based on their size. Similar to DNA separation, proteins migrate through the gel matrix, allowing researchers to analyze protein samples and study their characteristics.
- Microorganism motility assays: Agarose is used in laboratory experiments to assess the motility and mobility of microorganisms, such as bacteria or algae. Agarose plates or gels are prepared with appropriate growth media, and microorganisms are inoculated onto the gel surface. Researchers can observe and measure the movement of the microorganisms over time, providing insights into their motility patterns and behavior.
- Immobilization matrix: Agarose gels can serve as an immobilization matrix for enzymes, cells, or other biomolecules in various biotechnological processes. The porous nature of agarose gels allows for the entrapment or attachment of biomolecules while providing a suitable environment for their activity and stability. This immobilization can be utilized in applications such as enzyme purification, biocatalysis, and biosensors.
- Pharmaceutical and cosmetic industries: Agarose is also utilized in the pharmaceutical and cosmetic industries. It can be used as a stabilizing agent in drug formulations or as a thickening and gelling agent in cosmetic products.
Overall, agarose’s applications span from molecular biology research, DNA and protein analysis, to microbiology studies, biotechnological processes, and industrial uses. Its versatility, ease of use, and compatibility with biological systems make agarose a valuable tool in various scientific fields.
Functions of Polysaccharides
Polysaccharides play diverse roles in organisms and serve various functions. Some of the key functions of polysaccharides include:
- Energy Storage: Polysaccharides serve as a storage form of energy in living organisms. Glycogen, found in animals and fungi, and starch, found in plants, fruits, and seeds, are examples of polysaccharides that store energy. When energy is needed, these polysaccharides can be broken down into glucose units for metabolic processes.
- Hydrophobicity: Polysaccharides exhibit hydrophobic characteristics due to the presence of multiple hydrogen bonds. This property prevents water from infiltrating the molecules, making them hydrophobic. This can be important for maintaining the structural integrity of certain tissues and organs.
- Concentration Gradient and Nutrient Uptake: Polysaccharides can influence the concentration gradient, which in turn affects the uptake of nutrients and water by cells. By regulating the movement of substances across cellular membranes, polysaccharides contribute to maintaining homeostasis and proper cellular function.
- Formation of Glycolipids and Glycoproteins: Many polysaccharides can covalently bond with lipids and proteins, leading to the formation of glycolipids and glycoproteins. These compounds play crucial roles in cell signaling and communication. Glycolipids and glycoproteins are involved in intercellular signaling, cell adhesion, and recognition processes.
- Structural Support: Polysaccharides provide structural support in various organisms. For example, cellulose, a polysaccharide found in the cell walls of plants, contributes to the rigidity and strength of plant cell walls. In insects and fungi, chitin, another polysaccharide, is a major component of the extracellular matrix that surrounds cells and provides structural support.
- Protective Coating: Some polysaccharides, such as mucopolysaccharides, form protective coatings in organisms. These coatings can be found in mucus secretions and help to protect tissues and organs from physical damage, pathogens, and dehydration.
- Water Retention: Certain polysaccharides, like hyaluronic acid, have the ability to retain water molecules. This property contributes to the lubrication and hydration of tissues, particularly in connective tissues and joints.
The functions of polysaccharides are diverse and play critical roles in maintaining the structure, function, and overall health of organisms.
Examples of Polysaccharides
Polysaccharides are diverse and can be found in various organisms, serving different functions. Here are some examples of polysaccharides:
- Starch: Starch is the most common food storage polysaccharide found in plants. It is a polymer made up of α-D-glucose subunits and exists in two forms: amylose and amylopectin. Amylose is an unbranched polymer, while amylopectin is a branched form of starch. Starch serves as a source of energy for plants and can be broken down into glucose by enzymes called amylases.
- Cellulose: Cellulose is another important polysaccharide found in plants. It is a fibrous and insoluble polymer composed of β-D-glucose units linked by β-1,4 glycosidic bonds. Cellulose forms the structural component of plant cell walls, providing strength and rigidity. While humans lack the enzyme cellulase to digest cellulose, it serves as dietary fiber.
- Glycogen: Glycogen is the major storage polysaccharide in animals, often referred to as animal starch. Similar to starch, glycogen is a polymer of glucose. It consists of straight chains of glucose units linked by α-1,4 glycosidic bonds with frequent branching through α-1,6 glycosidic bonds. Glycogen is stored in the liver and muscles and serves as an energy reserve in animals.
- Hemicellulose: Hemicellulose is a polysaccharide composed of various sugars, including glucose and acetyl derivatives of glucose. It is found in plant cell walls and provides structural support to cells, along with cellulose. Hemicellulose has a more amorphous structure compared to cellulose.
- Inulin: Inulin is a naturally occurring carbohydrate found in many plants. It is a polymer made up of β-fructose residues linked by β-1,2 glycosidic bonds. Inulin serves as a storage polysaccharide in plants that do not store starch. It is extracted commercially from sources like chicory root and is used as a sugar and fat substitute in food manufacturing. Inulin is also utilized in medicine for kidney function assessment and has antibacterial properties.
- Chitin: Chitin is a nitrogen-containing carbohydrate primarily found in arthropods and fungi. It is composed of N-acetyl-D-glucosamine monomers linked by β-1,4 glycosidic bonds. Chitin is a major component of the exoskeleton of insects and the cell walls of fungi. It has applications in various industries, including agriculture as a biopesticide and medicine due to its therapeutic properties. Chitosan, a water-soluble derivative of chitin, is also used in different fields.
- Pectin: Pectin is a heteropolysaccharide found in the cell walls of plant cells. It consists mainly of galacturonic acid, a derivative of galactose. Pectin is present in the primary and middle lamellae of plant cells and is commercially used as a gelling agent in food products like jams and jellies. It also has applications in wound healing and medical adhesives.
- Bacterial Polysaccharides: Bacterial polysaccharides are found in bacterial cell walls and membranes. Examples include peptidoglycans, lipopolysaccharides (LPS), and exopolysaccharides. Peptidoglycan is a major component of the bacterial cell wall, providing structural support. LPS is found in the outer membrane of Gram-negative bacteria and plays a role in the immune response. Exopolysaccharides are thick, mucus-like polysaccharides secreted by pathogenic bacteria, forming a protective capsule-like structure around them.
These examples highlight the diversity and importance of polysaccharides in various biological processes and applications.
FAQ
What are polysaccharides?
Polysaccharides are complex carbohydrates made up of long chains of monosaccharide (simple sugar) units. They are macromolecules found in various natural sources, including plants, animals, and microorganisms.
What is the function of polysaccharides in living organisms?
Polysaccharides serve a variety of functions, including energy storage, structural support, cell-cell recognition, and immune response modulation.
What are some examples of polysaccharides?
Common examples of polysaccharides include starch, cellulose, glycogen, chitin, and agarose.
How do polysaccharides differ from monosaccharides and disaccharides?
Monosaccharides are single sugar units, while disaccharides are composed of two sugar units. Polysaccharides are long chains of monosaccharide units, typically containing hundreds to thousands of sugar molecules.
Are all polysaccharides digestible by humans?
No, not all polysaccharides are digestible by humans. For example, cellulose, a major component of plant cell walls, cannot be digested by human enzymes. However, some polysaccharides, such as starch and glycogen, can be broken down into glucose and used as a source of energy.
How do polysaccharides contribute to dietary fiber?
Polysaccharides, especially non-digestible ones like cellulose and certain hemicelluloses, contribute to dietary fiber. These fibers provide bulk to the diet, promote healthy digestion, and offer other health benefits, such as improving bowel regularity.
What are the applications of polysaccharides in the food industry?
Polysaccharides find wide application in the food industry as thickeners, stabilizers, gelling agents, and emulsifiers. They enhance texture, improve shelf stability, and create desired food structures.
Can polysaccharides be used in biomedical applications?
Yes, polysaccharides have numerous biomedical applications. They are used as drug delivery systems, wound dressings, tissue engineering scaffolds, and in other areas of regenerative medicine due to their biocompatibility and biodegradability.
How are polysaccharides classified?
Polysaccharides can be classified based on their monosaccharide composition, linkage types, branching patterns, and overall structure. They are categorized into groups such as homopolysaccharides, heteropolysaccharides, and glycosaminoglycans.
Are polysaccharides environmentally friendly?
Polysaccharides are often considered environmentally friendly due to their natural abundance, renewable sources, and biodegradability. They are increasingly being explored as sustainable alternatives to synthetic materials in various industries.
References
- Agoda-Tandjawa, G., Gao, Y., & Rinaudo, M. (2015). Polysaccharide-based nanocomposites and their applications. Carbohydrate Polymers, 125, 196–213. doi:10.1016/j.carbpol.2015.02.063
- Azizi, S., Ahmad, M. B., Hussein, M. Z., & Ibrahim, N. A. (2015). Recent advances in polysaccharides-based hydrogels for wound healing applications. Journal of Applied Polymer Science, 132(23), 42099. doi:10.1002/app.42099
- Braccini, I., & Pérez, S. (2001). Molecular basis of Ca2+-induced gelation in alginates and pectins: The egg-box model revisited. Biomacromolecules, 2(4), 1089–1096. doi:10.1021/bm010008g
- Cui, S. W., Zhang, L., & Sun, J. (2019). Polysaccharides: Structure, Properties, and Applications. CRC Press.
- Heinze, T., & Nikolajski, M. (2020). Cellulose-based hydrogels for biomedical applications: Current state and future perspectives. Biomacromolecules, 21(6), 1893–1912. doi:10.1021/acs.biomac.0c00091
- Kołodziejczyk, M., & Ścibisz, I. (2019). Polysaccharides as fat replacers in food products: A review. Trends in Food Science & Technology, 91, 103–112. doi:10.1016/j.tifs.2019.06.005
- Kumar, P., & Sandeep, K. P. (2018). Polysaccharides and their derivatives for versatile tissue engineering application. Macromolecular Bioscience, 18(7), 1800055. doi:10.1002/mabi.201800055
- Morelli, A., Piscopo, A., & Drioli, E. (2019). Polysaccharides for drug delivery and pharmaceutical applications. Polysaccharide Carriers for Drug Delivery, 263–288. doi:10.1016/b978-0-08-102553-6.00010-6
- Rinaudo, M. (2006). Main properties and current applications of some polysaccharides as biomaterials. Polymer International, 55(6), 572–576. doi:10.1002/pi.1966
- Zhang, Y., Shi, L., & Mei, L. (2017). Polysaccharide-based nanoparticles for drug delivery. Applications of Nanoscience in Photomedicine, 343–358. doi:10.1016/b978-0-323-46152-8.00012-4