What is Respiration?
- Respiration, in a physiological context, refers to the process by which oxygen is taken into the body from the external environment and transported to cells within the tissues, while carbon dioxide, a waste product of metabolism, is expelled in the opposite direction. This gas exchange is essential for maintaining cellular respiration, where cells utilize oxygen to generate energy in the form of ATP (adenosine triphosphate) and release waste products like carbon dioxide and water.
- There are two main aspects of respiration: physiological respiration and cellular respiration. While physiological respiration deals with the exchange of gases between the organism and its environment, cellular respiration is the metabolic process that occurs within individual cells. This metabolic process involves breaking down nutrients to release energy, primarily using oxygen in oxidation-reduction reactions.
- Physiological respiration in mammals involves a respiratory cycle of inhalation (breathing in) and exhalation (breathing out). Inhalation is usually an active process where the diaphragm contracts, causing air to flow into the lungs. In the lungs, gas exchange occurs in the alveoli, where oxygen moves from the air into the blood and carbon dioxide moves from the blood into the alveoli to be exhaled. Exhalation, in contrast, is often a passive process, though there are exceptions, such as when talking, singing, or performing strenuous activities.
- Gas exchange between the lungs and the blood happens through two key processes: ventilation and perfusion. Ventilation refers to the physical movement of air in and out of the lungs, while perfusion is the circulation of blood through the pulmonary capillaries. Together, these processes ensure that oxygen reaches the blood and carbon dioxide is expelled.
- One critical feature of respiration is the functional residual capacity of the lungs, a volume of air (around 2.5 liters in adults) that remains in the lungs even after exhalation. This residual air mixes with the fresh air inhaled during each breath, ensuring that the air in the lungs remains relatively stable in composition, which is necessary for efficient gas exchange.
- Cellular respiration, on the other hand, takes place inside cells. Here, cells oxidize nutrients to produce ATP, the primary energy carrier in biological systems. This process involves breaking carbon-hydrogen (C-H) bonds in molecules, releasing energy and producing by-products like carbon dioxide and water, which must be removed from the body through physiological respiration.
- Therefore, respiration as a whole can be understood as a two-part process: one part involves the mechanical movement of air and blood to exchange gases with the environment, and the other part is the biochemical process inside cells that generates energy. Both are essential for life, as one supplies the oxygen needed for cellular functions, and the other expels waste products.
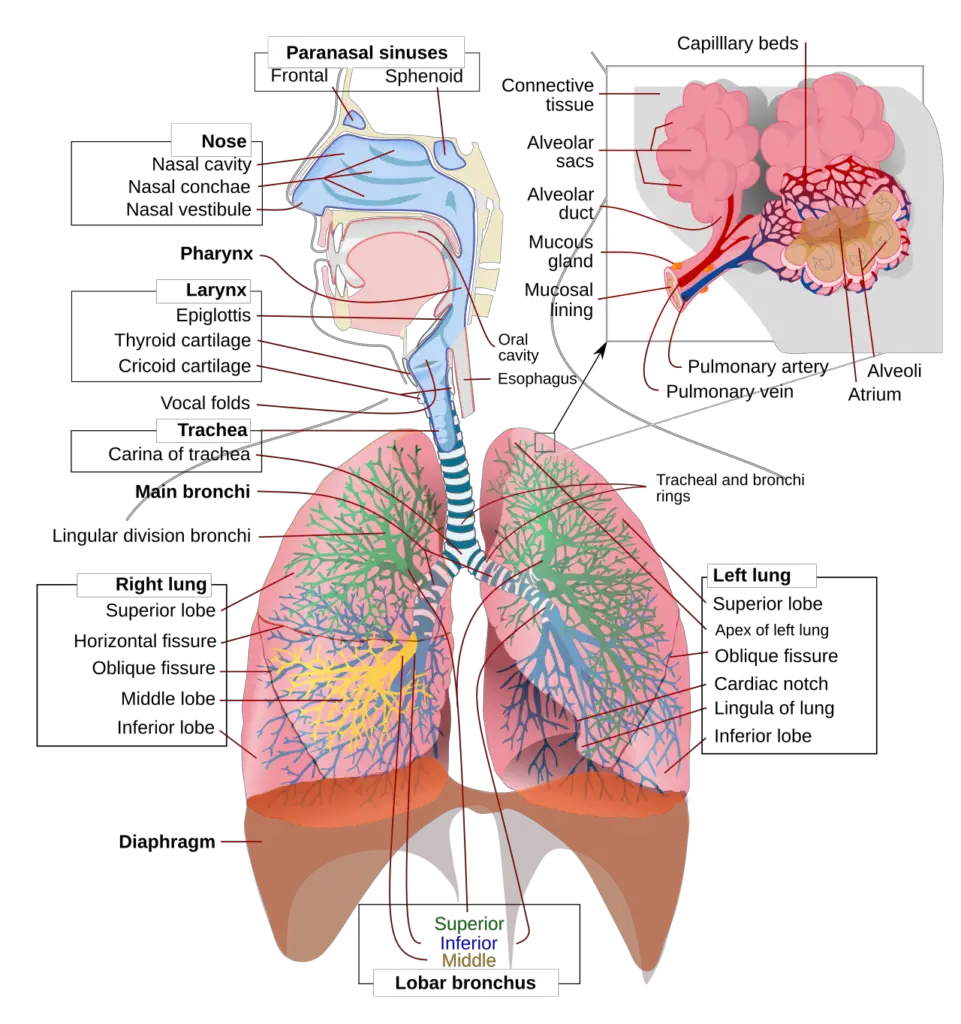
What is Respiration System?
- The respiratory system is a complex network of organs, tissues, and structures responsible for facilitating breathing and maintaining the exchange of gases crucial for survival. This system enables the body to take in oxygen, distribute it to cells, and remove carbon dioxide, a waste product of cellular metabolism. The primary components of the respiratory system include the airways, lungs, blood vessels, and respiratory muscles.
- The airways form the pathway for air to enter and exit the body. These airways include the nose, nasal passages, pharynx, larynx, trachea, bronchi, and bronchioles. The nose and nasal passages help filter, warm, and humidify the air before it enters the lungs. The trachea serves as a passage connecting the throat to the lungs, and it branches into bronchi, which then further divide into smaller bronchioles within the lungs.
- The lungs, the main organs of the respiratory system, are responsible for the exchange of gases. Inside the lungs are millions of tiny air sacs called alveoli. These alveoli are surrounded by capillaries, small blood vessels where oxygen is absorbed into the bloodstream, and carbon dioxide is removed. The alveoli provide a large surface area, which makes gas exchange more efficient.
- Blood vessels, particularly pulmonary arteries and veins, play a crucial role in transporting oxygenated and deoxygenated blood. Pulmonary arteries carry deoxygenated blood from the heart to the lungs, where carbon dioxide is released, and oxygen is absorbed. Once oxygenated, the blood returns to the heart through pulmonary veins, ready to be pumped throughout the body.
- Respiratory muscles, including the diaphragm and intercostal muscles, control the mechanics of breathing. The diaphragm, a dome-shaped muscle located below the lungs, contracts during inhalation, creating a vacuum that allows air to flow into the lungs. During exhalation, the diaphragm relaxes, pushing air out of the lungs. The intercostal muscles, located between the ribs, also assist by expanding and contracting the chest cavity during breathing.
- Together, these components ensure that oxygen is continuously supplied to the body’s cells and that carbon dioxide is efficiently expelled, maintaining proper gas balance essential for life. Therefore, the respiratory system is not just a set of organs but an integrated network that collaborates to perform a vital function for human health and survival.
Respiratory organs
The respiratory system consists of several critical organs that work together to facilitate breathing and gas exchange. These organs are structured to ensure the proper flow of oxygen into the body and the removal of carbon dioxide. Below is a detailed breakdown of the primary respiratory organs and their functions.
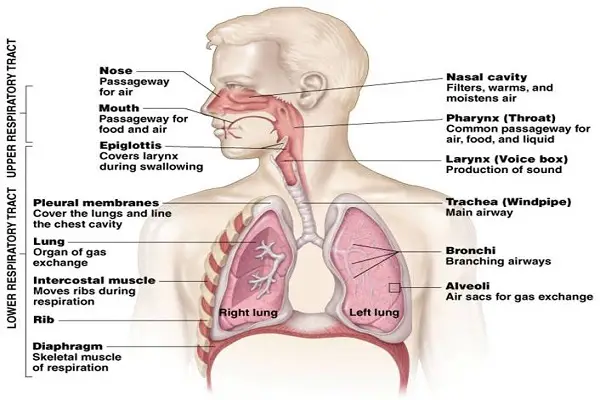
- Nose and Nasal Cavity:
- The nose is a key component of the respiratory system, situated above the mouth and made of hyaline cartilage. It is divided into two nasal cavities by the nasal septum, with the anterior part being cartilaginous and the posterior part bony.
- The nasal cavities open externally through nostrils and posteriorly into the nasopharynx through internal nares. Openings of the paranasal sinuses and nasolacrimal ducts are also present here.
- Lined with a mucous membrane and ciliated columnar epithelium, the nasal cavity contains hairs to trap dust and other particles.
- Functions:
- Traps dust particles in mucus or nasal hair, preventing them from entering the lungs.
- The ciliated epithelium sweeps out microorganisms to prevent infections.
- Warms and moistens incoming air.
- Detects odors via olfactory cells in the upper third of the nasal mucosa.
- Pharynx:
- The pharynx is a shared pathway for both the respiratory and digestive systems, extending from the base of the skull to the 5th cervical vertebra. It connects the nasal cavity to the mouth and is divided into three parts: nasopharynx, oropharynx, and laryngopharynx.
- Functions:
- Facilitates the passage of air from the nose to the trachea.
- Further warms and moistens the air as it passes through.
- Contains olfactory nerve endings for the sense of smell.
- Lymphatic tissues in the tonsils produce antibodies, providing protection.
- Auditory tubes help regulate ear pressure and contribute to hearing.
- Larynx:
- Also known as the voice box, the larynx is located in the anterior neck, connecting the pharynx and trachea. It is composed of several cartilages, including thyroid, cricoid, and arytenoid cartilages, which help keep it open. The larynx contains vocal cords responsible for sound production.
- Functions:
- Plays a crucial role in speech production.
- Provides a passage for air to travel from the pharynx to the trachea.
- Humidifies, filters, and warms air as it passes through.
- During swallowing, the epiglottis prevents food from entering the trachea, ensuring it passes into the esophagus.
- Trachea (Windpipe):
- The trachea is a hollow tube approximately 11-12 cm long and 2.5 cm in diameter, extending from the base of the larynx into the thoracic cavity. It is supported by 16-20 C-shaped cartilaginous rings that prevent its collapse during breathing. Its inner lining consists of pseudostratified ciliated epithelium and mucus-secreting goblet cells.
- Functions:
- The mucus and cilia help filter out dust and debris from the air.
- Constant ciliary movement moves mucus and trapped particles upward into the pharynx to be coughed out or swallowed.
- Plays a role in the cough reflex, which helps clear the airway.
- Bronchi:
- The trachea divides into two bronchi—right and left—that enter each lung. The right bronchus is shorter and wider than the left. Upon entering the lungs, the bronchi subdivide into secondary and tertiary bronchi, eventually forming bronchioles that lead to alveolar ducts.Functions:
- Bronchi serve as the primary passage for air into the lungs from the trachea.
- The trachea divides into two bronchi—right and left—that enter each lung. The right bronchus is shorter and wider than the left. Upon entering the lungs, the bronchi subdivide into secondary and tertiary bronchi, eventually forming bronchioles that lead to alveolar ducts.Functions:
- Alveoli:
- The alveoli are microscopic air sacs at the end of the bronchioles, where gas exchange occurs. Each alveolus is surrounded by capillaries, facilitating the diffusion of oxygen into the blood and the removal of carbon dioxide.
- Functions:
- Alveoli are responsible for the exchange of gases, primarily oxygen and carbon dioxide, between the lungs and blood.
- Type I pneumocytes in the alveoli facilitate gas exchange, while type II pneumocytes produce surfactant to reduce surface tension and prevent lung collapse.
- Lungs:
- The lungs are a pair of conical organs situated within the thoracic cavity. They are soft, spongy, and elastic, with the right lung consisting of three lobes and the left lung of two lobes, the latter containing a cardiac notch to accommodate the heart. The lungs are enveloped by two layers of pleural membranes, with the space between them filled with pleural fluid.
- Functions:
- Provide a large surface area (around 100 square meters) for efficient gas exchange.
- The pleural fluid between the membranes allows smooth, frictionless movement during breathing, protects against mechanical shocks, and keeps the lungs expanded.
The respiratory tract
The respiratory tract is an intricate system responsible for the intake of oxygen and the expulsion of carbon dioxide. This system ensures that air reaches the lungs and is purified, warmed, and filtered along the way, all while facilitating the vital exchange of gases in the body. The respiratory tract is composed of several distinct components, each playing a crucial role in this process.
- External Nostrils:
- The respiratory process begins at the external nostrils, where air is drawn into the body. These openings allow for the intake of air from the external environment.
- Nasal Chamber:
- After entering through the nostrils, the air passes into the nasal chamber. This chamber is lined with fine hair and mucus, both of which serve as essential filters that trap dust, dirt, and other airborne particles. Additionally, a network of blood vessels in the nasal chamber helps to warm the incoming air, ensuring that it is prepared for further travel into the respiratory system.
- Pharynx:
- The pharynx, located behind the nasal chamber, is a shared passageway for both air and food. Air flows through this tube, which functions as a common conduit for both the respiratory and digestive systems, guiding it toward the lower parts of the respiratory tract.
- Larynx:
- Also known as the voice box, the larynx houses the vocal cords. These vocal cords play a critical role in sound production. As air passes through the larynx, it enables the generation of sound necessary for speech.
- Epiglottis:
- The epiglottis is a flap-like structure that covers the glottis (the opening of the larynx). Its primary function is to prevent food from entering the windpipe (trachea) during swallowing. By doing so, it ensures that the respiratory and digestive pathways remain distinct and functional.
- Trachea:
- Commonly referred to as the windpipe, the trachea is a long, tubular structure that extends through the mid-thoracic cavity. It serves as the primary pathway for air to travel from the larynx toward the lungs. The trachea is supported by cartilaginous rings that keep it open, ensuring unobstructed airflow.
- Bronchi:
- At the base of the trachea, it divides into two main branches: the left and right bronchi, each leading to the corresponding lung. These bronchi serve as conduits for air to enter the lungs.
- Bronchioles:
- As the bronchi enter the lungs, they further subdivide into smaller and smaller branches known as bronchioles. These finer airways distribute air evenly throughout the lung tissues.
- Alveoli:
- The bronchioles terminate in tiny balloon-like structures called alveoli. Alveoli are the key sites for gas exchange, where oxygen is absorbed into the blood and carbon dioxide is expelled. These microscopic air sacs are surrounded by an extensive network of blood capillaries to facilitate this critical exchange of gases.
- Lungs:
- Humans possess a pair of lungs, which are large, sac-like organs that house the alveoli. The lungs are encased in a double-layered membrane called the pleura, which protects and cushions them. The pleura also helps reduce friction during the expansion and contraction of the lungs during breathing.
Respiratory System Functions
The following points summarize the key functions of the human respiratory system:
- Inhalation and Exhalation (Pulmonary Ventilation):
- The respiratory system facilitates breathing, also known as pulmonary ventilation, which includes both inhalation and exhalation. Air enters the body through the nose and moves down through the pharynx, larynx, and trachea, finally reaching the lungs. During exhalation, the air retraces this pathway, exiting the body. The process is driven by changes in lung volume and pressure, which allow air to flow into and out of the lungs efficiently.
- Exchange of Gases Between Lungs and Bloodstream:
- Inside the lungs, the primary site of gas exchange occurs in tiny air sacs called alveoli. Oxygen from the inhaled air diffuses across the alveolar walls into the pulmonary capillaries, where it binds to hemoglobin in the red blood cells and is transported throughout the body. Simultaneously, carbon dioxide, a metabolic waste product from the bloodstream, diffuses into the alveoli. This carbon dioxide is then expelled from the body during exhalation, ensuring that the blood remains oxygenated and free of excess carbon dioxide.
- Exchange of Gases Between Bloodstream and Body Tissues:
- Once oxygen-rich blood is circulated through the body, it reaches capillaries in various tissues. Here, oxygen diffuses from the blood into the cells, supporting cellular respiration. At the same time, carbon dioxide, produced as a byproduct of cellular metabolism, diffuses from the tissues into the bloodstream. This carbon dioxide-laden blood is then transported back to the lungs, where the gas is released into the alveoli and eventually exhaled.
- Vibration of the Vocal Cords (Sound Production):
- The respiratory system is integral to speech production. Within the larynx, vocal cords are housed and manipulated by muscles attached to the arytenoid cartilages. During exhalation, air passes through the vocal cords, causing them to vibrate. This vibration generates sound, which is further modified by the mouth, tongue, and lips to form speech. The coordinated action of the respiratory and phonatory systems enables humans to communicate through spoken language.
- Olfaction (Smelling):
- The respiratory system also plays a role in the sense of smell. During inhalation, air enters the nasal cavities, where odorant molecules bind to olfactory receptors located on the cilia of specialized sensory neurons. These receptors send signals to the olfactory bulbs in the brain, where the sensation of smell is processed. This function of olfaction is essential for detecting environmental cues, food, and potential hazards.
- Respiration Across Different Organisms:
- While humans and other higher organisms use lungs for respiration, various other species rely on different mechanisms. Lower organisms, such as unicellular creatures, depend on simple diffusion to exchange gases. Annelids like earthworms use a moist cuticle for gaseous exchange, while fish use gills to extract oxygen from water. This diversity in respiratory mechanisms highlights the adaptability of organisms to their environments.
Structure of Lung
The lungs play a critical role in the human respiratory system by facilitating the exchange of gases between the air and the bloodstream. The structure of the lungs is highly specialized, composed of multiple sections and cell types, each contributing to the lung’s function. The lung can be divided into the conducting portion and the respiratory portion, which together allow air to be conditioned and then exchanged with the blood.
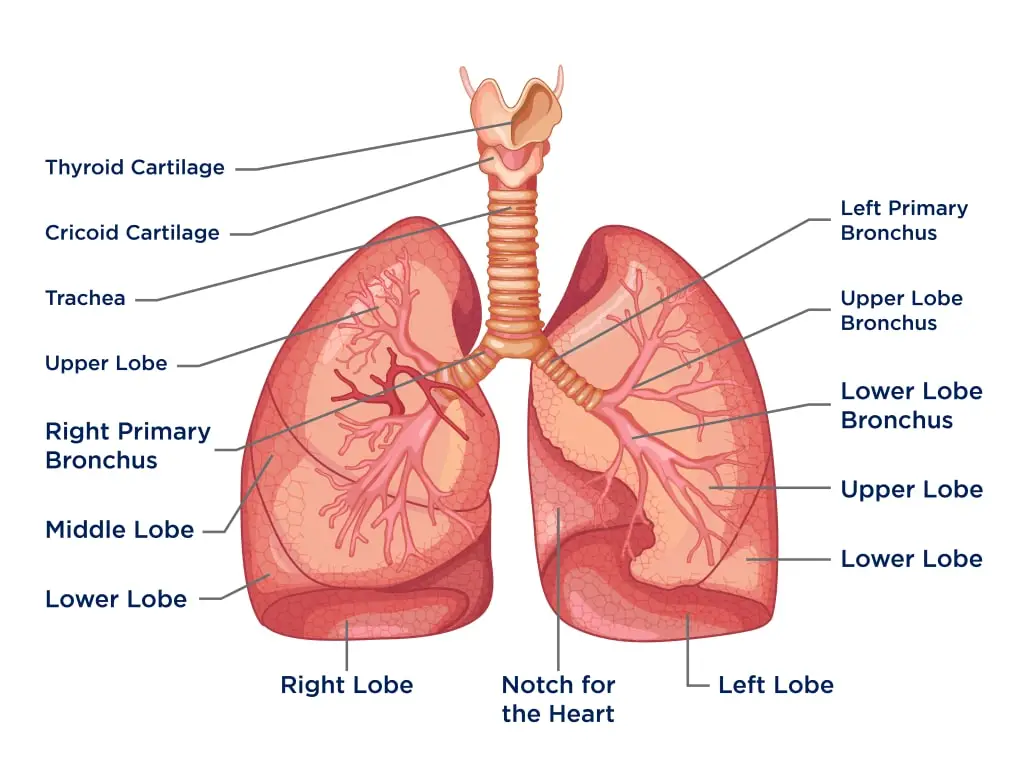
- Conduction Portion of the Lung:
- The conducting portion of the lung starts at the trachea and extends down to the terminal bronchioles.
- Outside the lungs, the conduction system includes the nasal cavities, nasopharynx, larynx, and trachea.
- Inside the lungs, the conducting portion begins with the paired main bronchi, which branch into lobar (secondary) bronchi, and further into segmental (tertiary) bronchi.
- The bronchi divide progressively into smaller bronchioles. As this occurs, cartilage disappears, marking the histological shift from bronchi to bronchioles.
- The terminal bronchioles are the last part of the conducting portion, which then transitions into the respiratory bronchioles—the starting point of the respiration portion of the lung.
- Functions of the Conducting Portion:
- This section of the lung serves as a pathway for air movement and conditioning before it reaches the deeper areas for gas exchange.
- Specialized cells in this portion work together to warm, moisturize, and remove particles from the air. These processes ensure the air is properly conditioned for respiration.
- Respiratory Epithelium Cells:
- The respiratory epithelium, which lines the entire respiratory tract, consists mainly of ciliated pseudostratified columnar epithelium.
- There are five major types of cells in the conducting portion:
- Ciliated Cells: These are the most abundant cells and are responsible for the mucociliary escalator, a primary defense mechanism of the lungs. The cilia beat rhythmically to move trapped debris in the mucus towards the pharynx, where it is swallowed or expelled through coughing.
- Goblet Cells: These cells, named for their goblet-like appearance, secrete mucus that traps particles in the air. As the airways get smaller, the number of goblet cells decreases, eventually being replaced by club cells in the bronchioles.
- Basal Cells: These cells serve as attachment points for ciliated cells and goblet cells. Acting as stem cells of the respiratory epithelium, they have the potential to differentiate into either type, thus maintaining the integrity of the respiratory lining.
- Brush Cells: Also referred to as type III pneumocytes, these cells are sparsely distributed and feature short microvilli on their apical surface. Though their function is not definitively known, they are believed to have a chemoreceptive role due to their association with nerve endings.
- Neuroendocrine Cells (Kulchitsky Cells): Found in small clusters within the bronchial mucosa, these cells contain neurosecretory granules and secrete various factors like catecholamines and polypeptides such as serotonin and calcitonin.
- Submucosal Glands and Smooth Muscle:
- The bronchial submucosa contains submucosal glands that consist of both serous and mucinous cells, similar to those found in salivary glands. These glands secrete substances onto the bronchial mucosa through ducts, contributing to the moist environment needed for proper air conditioning.
- Smooth muscle bundles are present throughout the airways and become progressively less prominent as the airways transition from the bronchi to the alveoli. These smooth muscles help regulate airflow by contracting and relaxing, allowing for fine-tuning of the respiratory process.
Function of Lung
The lungs serve as the central organs of respiration, performing crucial roles in both gas exchange and various non-respiratory functions. Their intricate structure enables them to efficiently support life by ensuring the intake of oxygen and removal of carbon dioxide, while also engaging in several other physiological processes.
- Respiratory Functions:
- Gas Exchange: The primary function of the lungs is the exchange of gases, a process essential for sustaining life. This occurs within the alveoli, which are the functional units of the lungs. Oxygen is absorbed from the air into the bloodstream, while carbon dioxide, a metabolic waste product, is expelled from the blood through the alveolar-capillary bed. This exchange of gases maintains the necessary oxygen levels for cellular processes and removes carbon dioxide to prevent toxicity.
- Air Conditioning: The conducting portion of the lungs has the secondary function of conditioning the air before it reaches the alveoli. Air is warmed or cooled to body temperature as it passes through the nasal and bronchial passages. The air is also humidified, ensuring it is saturated with moisture to protect the delicate tissues of the lungs. Moreover, foreign particles like dust, bacteria, and viruses are filtered out. This is accomplished through mucus secretion, which traps particles, and cilia, whose coordinated beating helps clear the mucus from the respiratory passages, keeping the airways clean.
- Non-Respiratory Functions:
- Chemical Conversion: The lungs also perform non-respiratory functions that play a role in regulating blood pressure. One example is the conversion of angiotensin-I into its active form, angiotensin-II, within the lungs. Angiotensin-II is a potent vasoconstrictor that raises blood pressure, making the lungs an important site for blood pressure regulation.
- Degradation of Chemical Mediators: In addition to converting angiotensin, the lungs are involved in the inactivation of various vasoactive substances. These include bradykinin, serotonin, and norepinephrine, which are all involved in the regulation of vascular tone. By degrading these chemical mediators, the lungs help regulate systemic vascular functions.
- Secretion of Hormones: The bronchial mucosa houses a small group of neuroendocrine cells, also known as Kulchitsky cells. These cells secrete several factors, including catecholamines and polypeptide hormones such as calcitonin, serotonin, and gastrin-releasing factors (bombesin). These hormones play roles in diverse physiological processes, including metabolism, neurotransmission, and gastrointestinal function.
- Defense Mechanism: The pulmonary epithelium serves as the first line of defense for the inspired air. Acting as a barrier, it prevents harmful substances from entering the bloodstream, safeguarding the body from infections and environmental pollutants.
Histochemistry and Cytochemistry of Lung
The study of lung tissue using histochemical and cytochemical methods reveals essential cellular and molecular insights, particularly in relation to lung pathology, disease diagnosis, and treatment approaches. These techniques utilize specific staining methods and molecular markers to identify and categorize various lung cell types and pathological states.
- Histochemistry of the Lung:
- Lectin Histochemistry (LC): Lectin histochemistry enables the identification and typing of lung cells based on glycoconjugate profiles. In the lungs, cells like type I and type II pneumocytes can be differentiated using lectin typing, which focuses on the sugars present on the cell surfaces. For example, type I pneumocytes are identifiable with Triticum vulgaris lectin, while type II pneumocytes can be marked by Hippeastrum hybrid or Maclura pomifera lectins.
- Alveolar Macrophages: In normal lung tissue, alveolar macrophages display positive reactions to multiple N-linked saccharides, such as N-acetylgalactosamine, N-acetylglucosamine, terminal β-D-galactose, and sialyl groups. These macrophages are also positive for the CD68 marker, confirming their presence and functionality in immune defense within the lung.
- Immunohistochemistry (IHC): Immunohistochemical analysis helps to identify specific proteins in lung tissues. This method is particularly valuable in diagnosing lung cancer, where adenocarcinomas can be detected using markers like TTF-1 and NapsinA, both expressed in over 85% of adenocarcinoma cases. Additionally, IHC can distinguish between primary lung cancers and metastatic tumors by examining CK7 and CK20 profiles and other molecular markers.
- Lung Cancer and Targeted Therapy: IHC also plays a role in the modern treatment of lung cancer, particularly in cases involving non-small cell lung carcinoma (NSCLC). Actionable mutations such as EGFR mutations and ALK translocations are key targets for tyrosine kinase inhibitors (TKIs), forming the basis for personalized treatment strategies in NSCLC.
- Cytochemistry of the Lung:
- Electron Microscopy and Flow Cytometry: Cytochemical studies of lung tissue use advanced methods like electron microscopy and flow cytometry to analyze cellular components and disease markers. Specific enzyme activities, such as alpha-naphthyl acetate esterase (ANAE), butyrate esterase (BUT), and chloroacetate esterase (CHL), can be assessed to identify lung diseases.
- Assessment of Pathological Changes: In obstructive pulmonary diseases, the remodeling of the extracellular matrix (ECM) is a key factor in disease progression. The second-harmonic generation (SHG) technique measures collagen organization, which helps in diagnosing conditions like asthma, chronic obstructive pulmonary disease (COPD), and idiopathic pulmonary fibrosis (IPF). Changes in collagen structure and deposition are biochemical hallmarks of these conditions, contributing to their pathology.
- Alveolar Macrophage Modulation: Cytochemical markers, such as chloroacetate esterase (CHL) and Perls’ reaction (for intracellular iron detection), can reveal changes in alveolar macrophages associated with lung cancer. Increased CHL and iron levels in macrophages are indicative of non-small cell lung cancer, making these markers useful in early detection.
- Galectin-3 in Pulmonary Fibrosis: Galectin-3 (Gal-3) has emerged as a key biomarker in pulmonary fibrosis. Elevated levels of Gal-3 in lung tissue have been observed in patients with this condition, offering a potential target for diagnostic and therapeutic interventions.
Light and Electron Microscopy structure of Lung
The structure of the lung can be examined using both light and electron microscopy, each offering distinct insights into the organization and function of lung tissues. Light microscopy allows for the visualization of overall architecture and cellular arrangements, while electron microscopy provides a detailed view of the fine structural components essential for gas exchange and lung function.
- Light Microscopy:
- Conducting Zone: The conducting portion of the lung includes structures up to the terminal bronchiole. This zone is responsible for moistening, warming, and filtering the air before it reaches the respiratory region, which starts at the respiratory bronchioles.
- Respiratory Zone: This zone encompasses the respiratory bronchiole, alveolar duct, alveolar sac, and alveoli, where gas exchange occurs. The acinus, which is the region distal to the terminal bronchioles, is essential for respiratory function and is made up of respiratory bronchioles, alveolar ducts, and alveolar sacs, resembling a grape bunch in appearance.
- Cellular Composition: The alveolar epithelium consists primarily of type I and type II pneumocytes, along with occasional brush cells, club cells, and alveolar macrophages. The type I pneumocytes (90-95%) are thin, flat cells facilitating gas exchange, while type II pneumocytes (5-10%) secrete pulmonary surfactant to reduce surface tension in the alveoli. The presence of pores of Kohn in alveolar walls facilitates air movement between adjacent alveoli, which is beneficial in cases of blockage.
- Goblet and Club Cells: As the respiratory tree transitions from the conducting zone to the respiratory zone, goblet cells decrease while club cells increase in number. These club cells contribute to airway secretions and possess stem cell-like properties.
- Serous Membrane: The lungs are enveloped by a serous membrane called the pleura, which comprises a visceral layer lined with mesothelial cells and connective tissue containing elastic fibers.
- Electron Microscopy:
- Respiratory Bronchiole: Electron microscopy reveals that the respiratory bronchiole is the transition zone between the conducting and respiratory portions. Its walls are interrupted by sac-like alveoli, and it is lined with ciliated cuboidal epithelium, although cilia may be absent in more distal sections. Clara cells, which have roles in surfactant production, detoxification, and serving as stem cells, are present in small numbers.
- Alveolar Ducts: Alveolar ducts result from the branching of respiratory bronchioles. Unlike bronchioles, alveolar ducts do not possess walls but are formed by the openings of numerous alveoli. Surrounding these ducts are aggregations of smooth muscle, collagen, and elastic fibers, which help regulate air movement within the alveoli.
- Alveoli: The alveoli are the specialized air sacs, approximately 200 µm in diameter, serving as the primary sites for gas exchange. The lungs contain about 300 million alveoli, providing a vast surface area for oxygen and carbon dioxide exchange, approximately 140 m². The alveolar wall comprises three main tissue components: surface epithelium, supporting tissue, and a continuous capillary network. The thin barrier (0.1-1.5 µm) formed by the epithelium and capillaries ensures efficient gas diffusion, known as the blood-gas barrier.
- Type I Pneumocytes: These squamous cells account for 95-97% of the alveolar surface area and are characterized by their thin cytoplasm, which facilitates gas diffusion. Their close arrangement with tight junctions prevents the leakage of fluid into the alveolar space.
- Type II Pneumocytes: Comprising about 3-5% of the alveolar surface, type II pneumocytes are cuboidal and produce pulmonary surfactant, critical for maintaining alveolar stability. Their cytoplasm contains abundant organelles, such as rough endoplasmic reticulum and lamellar bodies, which store surfactant components.
- Alveolar Macrophages: Known as dust cells, these immune cells arise from blood monocytes and serve as defenders against inhaled particulates and pathogens. They can be found free in the alveolar spaces or in the inter-alveolar septa, actively phagocytosing harmful substances. In cases of industrial lung diseases like silicosis or asbestosis, macrophages engulf inhaled particles, leading to chronic inflammation and fibrosis.
Mechanism of Breathing
The mechanism of breathing is a fundamental physiological process that involves the movement of air into and out of the lungs, allowing for gas exchange essential for survival. This process encompasses two primary phases: inspiration (inhalation) and expiration (exhalation). Understanding the mechanics of breathing is crucial for both students and educators as it illustrates how the respiratory system functions to maintain homeostasis.
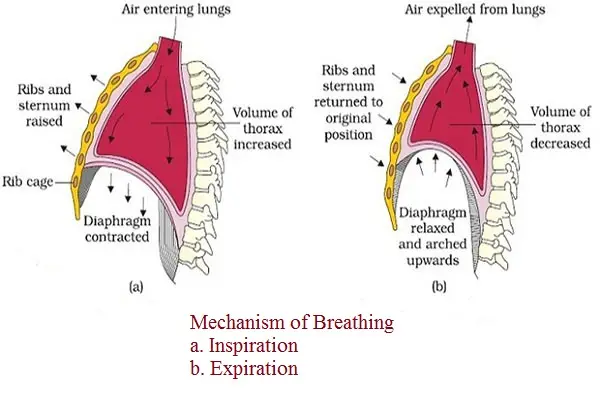
- Ventilation Overview
- Ventilation consists of two phases: inspiration, where air is drawn into the lungs, and expiration, where air is expelled from the lungs.
- Key anatomical features include:
- A continuous column of air extending from the pharynx to the alveoli of the lungs.
- The lungs are housed within a sealed thoracic cavity formed by the rib cage, which connects posteriorly to the vertebral column and anteriorly to the sternum.
- The intercostal muscles are situated between the ribs, and the diaphragm along with connective tissue forms the floor of the thoracic cavity.
- The pleura, a double-layered membrane, ensures that the lungs adhere to the thoracic wall while minimizing space due to the surface tension of the fluid between the pleurae.
- Inspiration
- Inspiration is classified as the active phase of breathing, necessitating muscular contraction.
- During inspiration:
- The diaphragm, initially dome-shaped, contracts and flattens.
- The external intercostal muscles contract, resulting in the upward and outward movement of the rib cage.
- These contractions increase the volume of the thoracic cavity.
- Consequently, lung volume expands since the lungs adhere to the thoracic cavity wall.
- As lung volume increases, the pressure within the alveoli decreases, creating a partial vacuum.
- The lower alveolar pressure compared to atmospheric pressure facilitates the inflow of air from outside the body into the respiratory passages and ultimately into the alveoli.
- It is important to note that air enters the lungs due to this partial vacuum rather than forcing the lungs open. Therefore, humans inhale through negative pressure.
- Although inspiration is an active process, the actual flow of air into the alveoli is passive.
- Expiration
- In contrast, expiration is typically a passive process requiring little to no effort.
- During expiration:
- The diaphragm and external intercostal muscles relax.
- The rib cage returns to its resting position, moving down and inward.
- The elastic properties of the thoracic wall and lung tissue enable them to recoil, aiding in expelling air.
- As the thoracic cavity decreases in size, the pressure within the lungs exceeds atmospheric pressure, leading to the expulsion of air through the respiratory passages.
- Volumes of Air Exchanged During Ventilation
- Various volumes of air are involved during breathing:
- Tidal Volume: This refers to the amount of air exchanged during normal, relaxed breathing, approximately 500 mL.
- Vital Capacity: This is the maximum volume of air that can be inhaled or exhaled in a single breath, combining the maximum inspiratory and expiratory volumes.
- Inspiratory Reserve Volume: This represents the additional air that can be inhaled beyond tidal volume, typically about 2,900 mL.
- Expiratory Reserve Volume: This is the additional air that can be exhaled after normal expiration, averaging around 1,400 mL.
- Residual Volume: Approximately 1,000 mL of air remains in the lungs even after a forceful exhalation; this air does not participate in gas exchange and occupies the “dead air space” in the respiratory passages.
- Various volumes of air are involved during breathing:
- Air Pressure Dynamics
- The air pressure within the lungs is variable and crucial to the mechanics of breathing.
- When alveolar pressure falls, air enters the lungs during inspiration; conversely, when alveolar pressure exceeds atmospheric pressure, air is expelled during expiration.
- The flow rate of air is proportional to the magnitude of the pressure difference between the lungs and the external environment.
Mechanism of Respiration in Human
Respiration in humans is a vital biological process involving the exchange of oxygen and carbon dioxide within the body. This process can be dissected into four primary phases: external respiration, transport of oxygen, internal respiration, and transport of carbon dioxide. Each phase plays a critical role in ensuring that the body receives adequate oxygen while efficiently removing carbon dioxide.
- External Respiration
- This phase occurs in the lungs and is the initial step of respiration.
- It involves the diffusion of gases between the alveoli and blood across the respiratory membrane.
- During inspiration, oxygen is drawn into the lungs, where the partial pressure of oxygen is greater than that in the blood capillaries.
- Consequently, oxygen diffuses from the lungs into the blood.
- In contrast, venous blood contains a higher concentration of carbon dioxide compared to the alveolar air.
- This difference in partial pressures allows carbon dioxide to diffuse from the blood into the lungs, facilitating gas exchange and maintaining equilibrium.
- Transport of Oxygen
- After external respiration, oxygen must be transported from the lungs to body tissues.
- Oxygen is transported in two main ways: through plasma and red blood cells (RBCs).
- Due to the poor solubility of oxygen in plasma, only about 3% of the total oxygen is carried in this manner.
- The majority of oxygen (approximately 97%) is transported by red blood cells.
- Hemoglobin, a respiratory pigment found in RBCs, combines with oxygen to form oxyhemoglobin due to its high affinity for oxygen.
- The reaction can be represented as follows:
- Hb4+4O2→Hb4O8
- Oxyhemoglobin is an unstable compound, allowing for rapid dissociation as it reaches the tissues, where it releases oxygen:
- Hb4O8→Hb4+4O2
- Hemoglobin contains four heme groups, enabling it to bind to four molecules of oxygen.
- In a typical adult, there are about 15 grams of hemoglobin per 100 mL of blood, with each gram capable of carrying approximately 1.34 mL of oxygen, culminating in about 20 mL of oxygen per 100 mL of blood.
- Internal Respiration
- This process occurs at the cellular level, commonly referred to as tissue respiration.
- Internal respiration involves two significant steps:
- Dissociation of Oxyhemoglobin:
- As oxyhemoglobin reaches the tissues, it dissociates into free oxygen and hemoglobin.
- This oxygen then diffuses into the tissues, while hemoglobin returns to the RBCs to bind more oxygen.
- Oxidation of Food:
- In the tissues, oxygen facilitates the oxidation of glucose in the presence of respiratory enzymes, releasing energy, water, and carbon dioxide.
- This reaction can be summarized as:
- C6H12O6→6CO2+6H2O+Energy
- The energy produced is stored in the form of ATP within the mitochondria, supporting various metabolic activities, while carbon dioxide is released as a waste product.
- Dissociation of Oxyhemoglobin:
- Transport of Carbon Dioxide
- Carbon dioxide is a byproduct of tissue respiration and must be effectively transported from the tissues back to the lungs for exhalation.
- Carbon dioxide is transported in three primary forms:
- As Carbonic Acid:
- Carbon dioxide reacts with water in the red blood cells to form carbonic acid, catalyzed by the enzyme carbonic anhydrase.
- This accounts for approximately 7% of total carbon dioxide transport:
- CO2 + H2O + carbonic anhydrase→H2CO3(carbonic acid)
- As Bicarbonate Ions:
- Carbonic acid ionizes quickly into bicarbonate and hydrogen ions, with bicarbonate ions being transported through the RBC membrane into the plasma.
- This form comprises around 70% of total carbon dioxide transport:
- H2CO3→HCO3−+H+
- In the plasma, bicarbonate may combine with sodium or potassium to form sodium bicarbonate or potassium bicarbonate.
- At the lungs, bicarbonate combines with hydrogen ions to form water and carbon dioxide, which is then expelled during expiration.
- As Carbaminohemoglobin:
- Approximately 20-23% of carbon dioxide is transported as carbaminohemoglobin, formed when carbon dioxide reacts with the amino groups of hemoglobin:
- CO2+HHb-NH2→Hb-NH-COOH
- Approximately 20-23% of carbon dioxide is transported as carbaminohemoglobin, formed when carbon dioxide reacts with the amino groups of hemoglobin:
- As Carbonic Acid:
Transport of oxygen and carbon dioxide in blood
The transport mechanisms of oxygen (O₂) and carbon dioxide (CO₂) in the blood are fundamental to maintaining cellular respiration and overall metabolic functions in the body. These processes involve intricate interactions between the gases and blood components, ensuring efficient delivery and removal of respiratory gases.
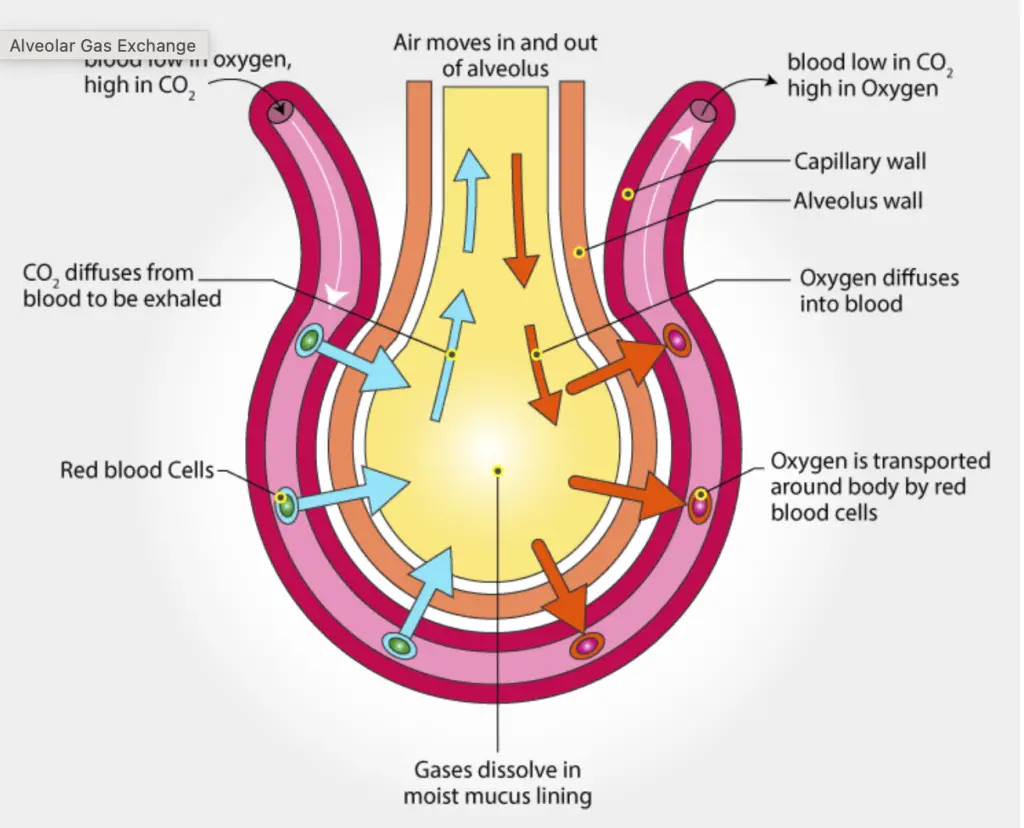
Transport of Oxygen
- The transport of oxygen during respiration is a crucial physiological process that ensures the delivery of oxygen from the lungs to tissues throughout the body. The majority of oxygen is transported in a bound form, primarily through red blood cells, with various physiological factors influencing its binding and release.
- Transport Mechanism:
- Approximately 97% of oxygen is carried by red blood cells, while only 3% is dissolved in the plasma.
- The red blood cells contain hemoglobin, a pigment that imparts the characteristic red color to blood. Hemoglobin plays a central role in oxygen transport by binding with oxygen to form oxyhemoglobin.
- Formation of Oxyhemoglobin:
- The formation of oxyhemoglobin is contingent upon several factors:
- Partial Pressure of Oxygen (pO₂): Higher pO₂ levels facilitate the binding of oxygen to hemoglobin.
- Hydrogen Ion Concentration (H⁺): Lower pH levels (higher acidity) can reduce the affinity of hemoglobin for oxygen, promoting oxygen release.
- Temperature: Lower temperatures are favorable for oxyhemoglobin formation.
- A single hemoglobin molecule can bind to up to four molecules of oxygen, which allows for efficient oxygen transport.
- The formation of oxyhemoglobin is contingent upon several factors:
- Ideal Conditions in the Alveoli:
- In the alveoli, conditions are optimal for the formation of oxyhemoglobin, characterized by:
- High pO₂ levels
- Low hydrogen ion concentration
- Lower temperatures
- These conditions promote the loading of oxygen onto hemoglobin, resulting in the saturation of hemoglobin with oxygen.
- In the alveoli, conditions are optimal for the formation of oxyhemoglobin, characterized by:
- Dissociation in Tissues:
- Conversely, in peripheral tissues, the conditions are less favorable for oxygen binding:
- Lower pO₂ levels, higher hydrogen ion concentrations, and increased temperatures favor the dissociation of oxyhemoglobin.
- As a result, oxygen is released from hemoglobin to meet the metabolic demands of the tissues.
- Conversely, in peripheral tissues, the conditions are less favorable for oxygen binding:
- Oxygen Delivery Efficiency: On average, every 100 mL of oxygenated blood can deliver approximately 5 mL of oxygen to tissues. This efficiency is vital for sustaining cellular respiration and supporting overall metabolic activity.
- Transport Mechanism:
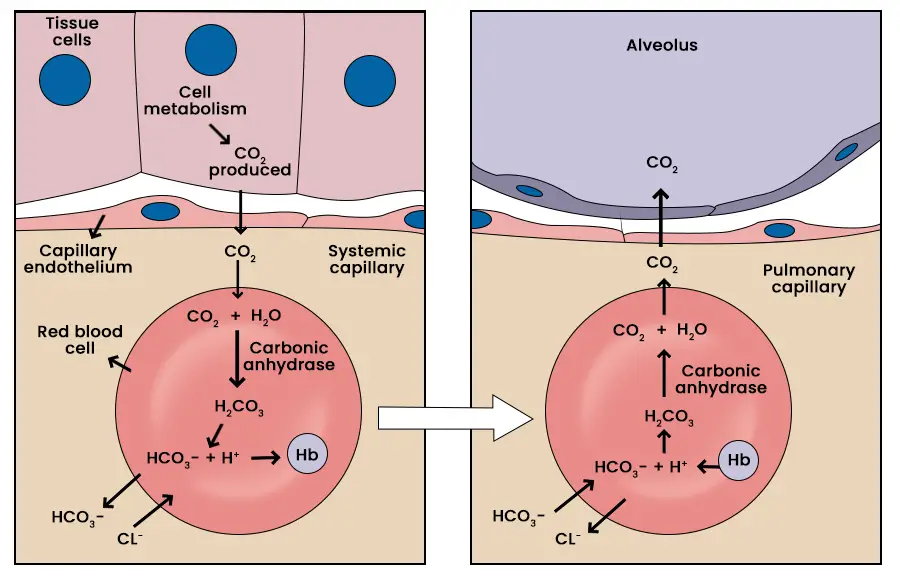
Transport of Carbon Dioxide
The transport of carbon dioxide (CO₂) during respiration is a vital physiological process that facilitates the removal of CO₂, a metabolic waste product, from the tissues to the lungs for exhalation. Carbon dioxide is transported in the blood via several mechanisms, each serving to ensure effective gas exchange and maintain acid-base balance within the body.
- Overview of Carbon Dioxide Transport:
- Carbon dioxide produced as a byproduct of cellular respiration disperses into the bloodstream from tissues.
- Approximately 20-25% of carbon dioxide binds with hemoglobin, forming carbaminohemoglobin.
- 7% is transported in a dissolved state within the plasma, and the remaining 70% is carried as bicarbonate ions (HCO₃⁻).
- Mechanisms of Carbon Dioxide Transport:
- Dissolved Carbon Dioxide:
- About 7% of CO₂ is transported directly dissolved in blood plasma.
- This dissolved CO₂ contributes approximately 0.3 mL per deciliter (dL) of blood.
- The partial pressure of CO₂ affects the tension of dissolved CO₂ in the blood.
- Formation of Carbonic Acid:
- The majority of carbon dioxide is converted into carbonic acid (H₂CO₃) in the presence of water (H₂O), primarily within red blood cells (RBCs).
- The enzyme carbonic anhydrase, which is zinc-activated, catalyzes the reaction: CO2+H2O→H2CO3
- This conversion predominantly occurs under low partial pressure of oxygen (pO₂) conditions, enhancing CO₂ transport efficiency.
- Bicarbonate Ion Transport:
- Approximately 70% of carbon dioxide is transported as bicarbonate ions (HCO₃⁻).
- In RBCs, carbonic acid rapidly dissociates into bicarbonate and hydrogen ions (H⁺): H2CO3→H++HCO3−
- Bicarbonate ions then exit the RBCs and enter the plasma, while chloride ions (Cl⁻) move from plasma into RBCs in exchange. This exchange is essential for maintaining electrical neutrality within the cells and is known as the chloride shift or Hamburger shift.
- Carbaminohemoglobin Formation:
- About 23% of carbon dioxide is transported as carbaminohemoglobin, formed by the reversible combination of CO₂ with amino groups of hemoglobin: Hb+CO2→Carbaminohemoglobin
- The binding of CO₂ to hemoglobin is favored in tissues where there is a high partial pressure of carbon dioxide (PCO₂) and low partial pressure of oxygen (pO₂).
- The formation of carbaminohemoglobin facilitates the transport of carbon dioxide back to the lungs.
- Dissolved Carbon Dioxide:
- Release of Carbon Dioxide in the Alveoli:
- In the lungs, where pO₂ is high, carbon dioxide dissociates from both carbaminohemoglobin and carbonic acid.
- The presence of carbonic anhydrase enables the reverse reactions, facilitating the release of CO₂ for exhalation.
- Thus, carbon dioxide is effectively exchanged for oxygen during the external respiration process.
- Efficiency of Carbon Dioxide Delivery:
- On average, every 100 mL of deoxygenated blood can deliver approximately 4 mL of carbon dioxide to the alveoli.
- This efficient transport mechanism is crucial for maintaining homeostasis and ensuring proper gas exchange during respiration.
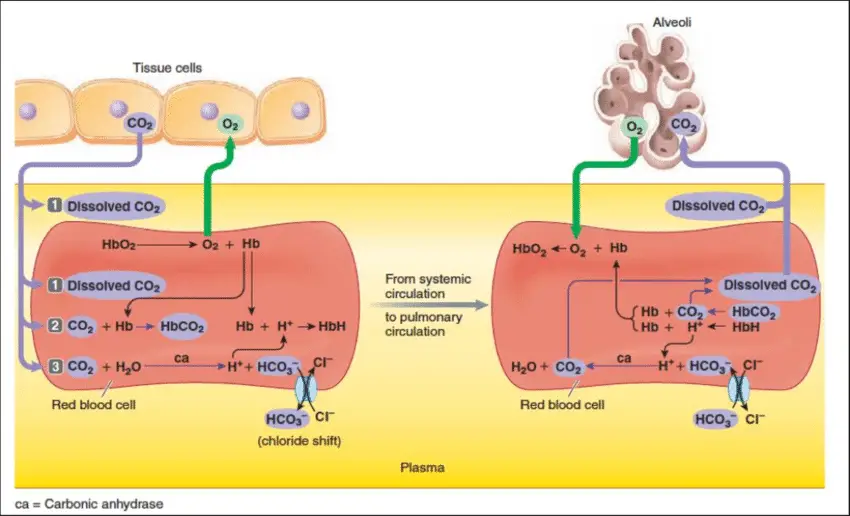
Regulation of respiration
The regulation of respiration is a complex interplay of neural and non-neural factors that ensures the body maintains appropriate levels of oxygen (O₂) and carbon dioxide (CO₂) during various physiological states. Understanding these mechanisms is essential for students and educators as it provides insight into the intricate processes that govern breathing.
- Neural Regulation
- Phrenic and Intercostal Nerves: These two nerves are critical in regulating the activity of respiratory muscles, specifically the diaphragm and external intercostals. The phrenic nerve stimulates the diaphragm to contract, while the intercostal nerves activate the intercostal muscles.
- Medulla and Pons: The medulla and pons house the neural centers responsible for controlling the rhythm and depth of respiration.
- The medulla contains a pacemaker, known as the self-exciting inspiratory center, which sets the basic rhythm of breathing. It also includes an expiratory center that inhibits the pacemaker, creating a rhythmic pattern of inhalation and exhalation.
- The pons helps to smooth out the basic rhythm established by the medulla, ensuring a seamless transition between inspiration and expiration.
- Eupnea: This term refers to the normal respiratory rate, typically maintained at 12 to 15 respirations per minute under resting conditions.
- Hyperpnea: During physical exertion, the brain centers send increased impulses to the respiratory muscles, resulting in a more vigorous and deeper breathing pattern known as hyperpnea.
- Non-Neural Factors
- Physical Factors: While the medulla’s respiratory centers dictate the basic breathing rhythm, physical activities—such as talking, coughing, and exercising—can modify both the rate and depth of breathing. Additionally, an increase in body temperature can elevate the respiratory rate.
- Volition (Conscious Control): Although individuals can exert some voluntary control over their breathing, this control is limited. When oxygen levels in the blood drop or blood pH decreases, the respiratory centers override conscious efforts, ensuring the body’s needs are met.
- Emotional Factors: Breathing patterns can also be altered by emotional states. Reflexes initiated by emotional stimuli, acting through centers in the hypothalamus, can modify both the rate and depth of breathing.
- Chemical Factors: The most critical factors affecting respiratory rate and depth are the chemical levels of O₂ and CO₂ in the blood.
- Elevated CO₂ levels and decreased blood pH are significant stimuli for increasing both the rate and depth of breathing. Conversely, oxygen levels become important stimuli when they drop to dangerously low levels.
- Hyperventilation: This condition occurs when breathing becomes excessively rapid, leading to the expulsion of more CO₂. This process decreases carbonic acid levels in the blood, returning blood pH to a normal range when acidosis begins to occur.
- Hypoventilation: In contrast, hypoventilation refers to slow or shallow breathing, which allows CO₂ to accumulate in the blood. This situation brings blood pH back into a normal range when blood begins to become slightly alkaline.
Histology of the Respiratory System
The respiratory system is a complex network of organs and tissues essential for the exchange of carbon dioxide (CO₂) and oxygen (O₂) between the blood and the atmosphere. It facilitates the process of breathing, which involves inhaling oxygen and exhaling carbon dioxide, thereby enabling cellular respiration and energy production. This system can be divided into two main parts: the upper and lower respiratory tracts.
Components of the Respiratory System
- Upper Respiratory Tract
- Nasal Cavity: The entry point for air, lined by ciliated pseudostratified columnar epithelium that contains bipolar olfactory nerve cells. These olfactory cells have proteins that act as odorant receptors.
- Nasal Mucosa: Comprises olfactory nerves and glands that secrete a proteinaceous substance, maintaining moisture and trapping aromatic substances.
- Pharynx: Contains mucous glands and is lined with stratified squamous epithelium, transitioning to similar epithelium at the larynx’s proximal end.
- Lower Respiratory Tract
- Trachea: Serves as a conduit between the throat and bronchi.
- Bronchi: Divides into two primary bronchi leading to the lungs.
- Bronchioles: Smaller branches of the bronchi that lead to the pulmonary alveoli.
- Lungs: Composed of the bronchial tree, with airways branching into smaller tubes, ultimately terminating in alveoli where gas exchange occurs.
Respiratory Epithelium
The respiratory epithelium is crucial for protecting the respiratory system and maintaining moisture. It consists of several specialized cell types, each with distinct functions:
- Ciliated Cells: Most abundant, present from the trachea to respiratory bronchioles, containing 200-300 cilia per cell to facilitate mucous movement.
- Goblet Cells: Mucous-secreting cells located in the trachea and bronchi, contributing to the airway’s protective mucous layer.
- Basal Cells: Short cells found in the trachea and bronchi, positioned near the basal lamina, contributing to the epithelium’s pseudostratified appearance.
- Clara Cells (Bronchiolar Epithelial Cells): Non-ciliated cells in bronchioles involved in metabolizing pollutants and serving as progenitor cells after lung injury.
- Brush Cells: Non-ciliated columnar cells with microvilli, suspected to have chemoreceptor functions.
- Dense Core Granule Cells: Found throughout the airways, these cells are involved in neuroendocrine functions, though their role remains partially understood.
- Serous Cells: Non-ciliated cells in the trachea and bronchi that secrete glycoproteins and lysozymes, contributing to mucous viscosity.
- Intermediate Cells: Non-ciliated cells that replace lost epithelial cells, capable of differentiating into either goblet or ciliated cells.
Epithelial Transitions
The respiratory system showcases notable epithelial transitions, characterized by the following changes:
- The pseudostratified ciliated columnar epithelium of the trachea and bronchi gradually shifts to a simple columnar ciliated epithelium in bronchioles, and ultimately to simple squamous epithelium in alveolar ducts and alveoli.
- Ciliated cells progressively decrease in height from the trachea to terminal bronchioles.
Histology of the Alveolar Region
In the alveolar region, five primary cell types are identified:
- Alveolar Type I Cells: Squamous epithelial cells that cover approximately 95% of the alveolar surface. They are flattened to facilitate gas exchange.
- Alveolar Type II Cells: Granular pneumocytes that form tight junctions with Type I cells. They are secretory cells located in alveolar corners, producing surfactant to reduce surface tension.
- Capillary Endothelial Cells: Form the pulmonary capillary bed, which is the largest in the body, facilitating gas exchange by allowing the diffusion of O₂ and CO₂.
- Alveolar Macrophages: Large, wandering cells in the alveoli that ingest microorganisms and particulate matter, serving as a critical component of the immune response.
- Interstitial Cells: Fibroblast-like cells with the potential to differentiate into smooth muscle cells or fibroblasts, playing a role in lung development.
Lung Volumes and Lung Capacities
Understanding lung volumes and capacities is essential for comprehending respiratory physiology. These measurements indicate the various amounts of air the lungs can hold and are crucial for assessing respiratory function. The terms used to describe lung volumes and capacities provide insights into how the lungs operate during different phases of breathing.
Lung Volumes
- Tidal Volume (TV)
- Tidal volume is defined as the total amount of air inhaled or exhaled during normal, relaxed breathing.
- In a healthy adult, the tidal volume is approximately 500 mL.
- Inspiratory Reserve Volume (IRV)
- Inspiratory reserve volume refers to the additional volume of air that can be inhaled beyond the tidal volume.
- This volume ranges from 2500 to 3100 mL and represents the supplemental air that can be effectively inhaled after a normal breath.
- Expiratory Reserve Volume (ERV)
- Expiratory reserve volume is the additional air that can be forcibly exhaled after the expiration of a tidal volume.
- The ERV is about 1200 mL, indicating the capacity to exhale more air than what is typically done during a normal breath.
- Residual Volume (RV)
- Residual volume is the amount of air that remains in the lungs after a forceful exhalation, preventing lung collapse.
- This volume ranges from 1100 to 1200 mL and is crucial for maintaining a continuous gas exchange.
Lung Capacities
Lung capacities are the sums of various lung volumes and provide more comprehensive information about lung function:
- Total Lung Capacity (TLC)
- Total lung capacity is defined as the maximum amount of air that can fill the lungs after a maximal inhalation.
- In healthy adults, the total lung capacity is approximately 6000 mL.
- The formula for calculating TLC is:
TLC=TV+ERV+IRV+RV
- Vital Capacity (VC)
- Vital capacity refers to the maximum volume of air that can be exhaled after a maximum inhalation.
- This measure is essential for evaluating a person’s respiratory health.
- A decreased vital capacity can indicate restrictive lung diseases, which limit lung expansion, while normal vital capacity coupled with abnormal lung function may suggest obstructive lung diseases affecting airway patency.
- The formula for calculating VC is:
VC=TV+ERV+IRV
- Inspiratory Capacity (IC)
- Inspiratory capacity is the total volume of air that can be inhaled after a normal expiration.
- This capacity is approximately 3600 mL.
- The formula for calculating IC is:
IC=TV+IRV
- Functional Residual Capacity (FRC)
- Functional residual capacity refers to the volume of air remaining in the lungs after normal exhalation.
- This capacity is about 2400 mL.
- The formula for calculating FRC is:
FRC=ERV+RV
The oxyhemoglobin dissociation curve
The oxyhemoglobin dissociation curve is a crucial representation of how hemoglobin (Hb) binds to and releases oxygen in the bloodstream. This curve illustrates the relationship between the partial pressure of oxygen (PaO₂) and the oxygen saturation of hemoglobin (SaO₂), providing insights into the efficiency of oxygen transport and delivery to tissues throughout the body.
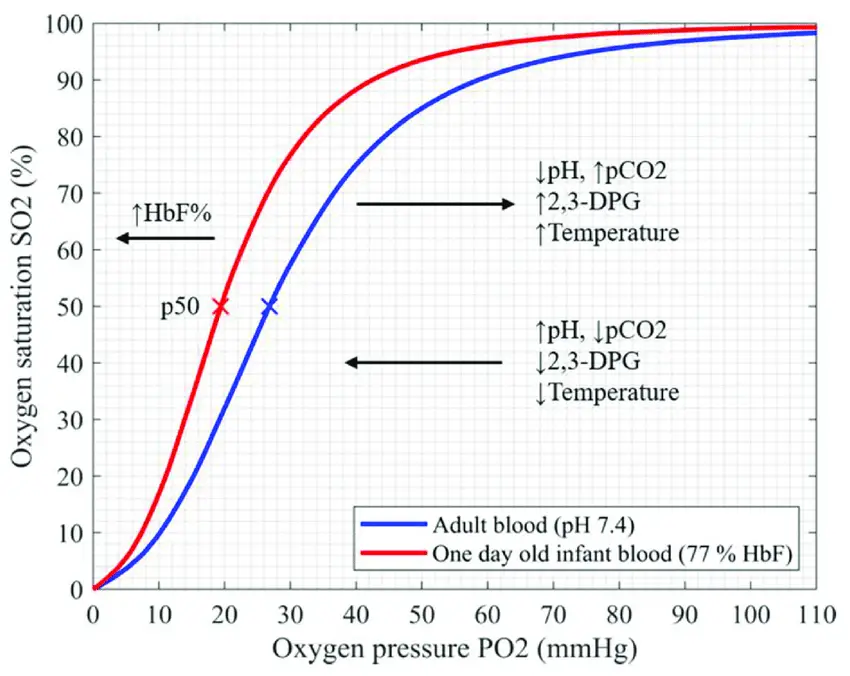
- Oxygen Transport by Hemoglobin:
- Hemoglobin is a protein found in red blood cells that is primarily responsible for transporting oxygen. While oxygen can also be carried by dissolving in blood plasma, this method accounts for only about 2% of the total oxygen transported. In contrast, approximately 98% of oxygen is bound to hemoglobin.
- Differentiating Concepts:
- It is essential to distinguish between:
- Oxygen Saturation (SaO₂): This measures the percentage of hemoglobin molecules in the blood that are bound to oxygen.
- Partial Pressure of Oxygen (PaO₂): This quantifies the amount of oxygen dissolved in the blood.
- It is essential to distinguish between:
- Mechanism of Hemoglobin Function:
- The hemoglobin molecule consists of four subunits: two alpha and two beta, each containing a heme group and globin chain. Each heme group contains an iron atom (in the ferrous form, Fe²⁺) capable of binding one oxygen molecule. Consequently, a single hemoglobin molecule can bind up to four oxygen molecules.
- Hemoglobin exists in two distinct states:
- T State (Tense): The deoxygenated form with a low affinity for oxygen.
- R State (Relaxed): The oxygenated form with a high affinity for oxygen.
- In the absence of oxygen, hemoglobin remains in the T state, requiring a higher partial pressure of oxygen (pO₂) for oxygen binding. However, when oxygen binds to one of the heme groups, it induces a conformational change that destabilizes the T state, facilitating the transition of the remaining subunits to the high-affinity R state. This process demonstrates positive cooperativity, allowing subsequent oxygen molecules to bind more easily.
- Oxygen Loading in the Lungs:
- In normal lungs, the pO₂ is significantly high at the alveolar-capillary junction, promoting the rapid loading of oxygen onto hemoglobin. This efficient process allows hemoglobin to reach nearly 100% saturation before exiting the pulmonary capillaries.
- Oxygen Release in Peripheral Tissues:
- Once in the systemic circulation, hemoglobin encounters lower pO₂ levels in peripheral tissues. In this environment, the T state is favored due to the reduced oxygen concentration, which facilitates the release of oxygen from hemoglobin. This mechanism enables hemoglobin to efficiently deliver oxygen to metabolically active cells.
- Oxyhemoglobin Dissociation Curve Representation:
- The oxyhemoglobin dissociation curve is graphically represented with oxygen saturation (SaO₂) plotted on the y-axis and partial pressure of oxygen (PaO₂) on the x-axis. The resulting curve typically exhibits a sigmoid shape, reflective of the positive cooperativity inherent in hemoglobin’s oxygen-binding dynamics.
- In the pulmonary capillaries, the high pO₂ results in a steep initial rise in saturation as oxygen molecules bind to hemoglobin. As saturation approaches maximum levels, the curve flattens, indicating that additional oxygen binding becomes less likely.
- Conversely, in systemic capillaries, lower pO₂ levels correspond to a more significant release of oxygen from hemoglobin, depicted by a steeper portion of the curve, emphasizing hemoglobin’s ability to provide oxygen to tissues as needed.
- The oxyhemoglobin dissociation curve is graphically represented with oxygen saturation (SaO₂) plotted on the y-axis and partial pressure of oxygen (PaO₂) on the x-axis. The resulting curve typically exhibits a sigmoid shape, reflective of the positive cooperativity inherent in hemoglobin’s oxygen-binding dynamics.
Factors Affecting Hb-O2 Dissociation Curve Respiratory System
The factors affecting the hemoglobin-oxygen (Hb-O₂) dissociation curve are essential for understanding how oxygen transport and release are regulated in the respiratory system. These factors can cause the curve to shift either to the left or to the right, influencing hemoglobin’s affinity for oxygen.
- Shift Dynamics:
- A rightward shift in the curve signifies a decreased affinity of hemoglobin for oxygen, facilitating oxygen unloading.
- Conversely, a leftward shift indicates an increased affinity for oxygen, meaning hemoglobin is less likely to release oxygen.
- pH (Acidity):
- A decrease in pH (increased acidity) results in a rightward shift of the dissociation curve, while an increase in pH (alkalinity) causes a leftward shift.
- At higher concentrations of hydrogen ions, hemoglobin stabilizes in the deoxygenated T-state. Consequently, as pH decreases and CO₂ levels rise, the affinity of hemoglobin for oxygen decreases.
- This inverse relationship is known as the Bohr effect, which becomes evident when metabolically active tissues convert glucose and oxygen into CO₂ and organic acids, thus promoting oxygen delivery to those tissues.
- Carbon Dioxide (CO₂):
- CO₂ affects the dissociation curve through two primary mechanisms: the Bohr effect and the formation of carbamino compounds. These compounds, including carbaminohemoglobin, stabilize the T-state of hemoglobin, thereby reducing its affinity for oxygen and facilitating oxygen unloading.
- Although a small portion of CO₂ is transported as carbamino compounds, most is carried in the bicarbonate buffer system. In red blood cells, CO₂ rapidly converts to carbonic acid via the enzyme carbonic anhydrase, which then dissociates into bicarbonate and hydrogen ions.
- The resulting increase in hydrogen ions further stabilizes hemoglobin in the T-state, promoting oxygen unloading and shifting the dissociation curve to the right.
- 2,3-Diphosphoglycerate (2,3-DPG):
- 2,3-Diphosphoglycerate is an intermediate glycolytic product produced within red blood cells that significantly influences hemoglobin’s oxygen affinity.
- High concentrations of 2,3-DPG lead to a rightward shift of the dissociation curve, while low concentrations cause a leftward shift.
- The relationship between hydrogen ions and 2,3-DPG is inversely proportional. An increase in hydrogen ions in red blood cells leads to a decrease in 2,3-DPG levels. This is particularly evident at high altitudes, where reduced oxygen levels induce hyperventilation, lowering pCO₂ and hydrogen ions and resulting in a leftward shift of the curve. This shift increases the production of 2,3-DPG in red blood cells, shifting the curve back to the right and establishing a critical mechanism for respiratory compensation.
- Temperature:
- The influence of temperature on the dissociation curve is straightforward. Higher temperatures favor oxygen unloading, resulting in a rightward shift of the curve, while lower temperatures lead to a leftward shift.
- For example, during exercise, muscle temperature increases due to metabolic activity, prompting a rightward shift that facilitates greater oxygen unloading from hemoglobin to meet increased tissue demand.
- Carbon Monoxide (CO):
- Hemoglobin binds carbon monoxide 200 to 300 times more strongly than oxygen, forming carboxyhemoglobin and blocking oxygen from binding to hemoglobin due to competitive inhibition.
- The binding of one CO molecule enhances the affinity of the remaining binding sites for oxygen, resulting in a leftward shift of the dissociation curve. This shift hinders oxygen unloading in peripheral tissues, leading to significantly lower tissue oxygen concentrations. Consequently, individuals exposed to carbon monoxide can experience severe tissue hypoxia, despite maintaining a normal arterial partial pressure of oxygen (PaO₂). Symptoms of carbon monoxide poisoning may include headache, malaise, altered mental status, shortness of breath, seizures, and characteristic cherry-red lips. Standard pulse oximetry may not detect carboxyhemoglobin, yielding falsely normal readings.
- Fetal Hemoglobin (HbF):
- Fetal hemoglobin differs structurally from adult hemoglobin, consisting of two alpha and two gamma chains. The gamma chains confer a lower affinity for 2,3-DPG, allowing HbF to exhibit a higher affinity for oxygen at lower partial pressures, resulting in a leftward shift of the dissociation curve.
- This property is advantageous in utero, enabling the fetus to extract oxygen from maternal circulation more efficiently. At the placenta, 2,3-DPG more readily interacts with adult hemoglobin, promoting oxygen unloading, while fetal hemoglobin remains unaffected by 2,3-DPG and can bind oxygen effectively.
- Davies A, Moores C. STRUCTURE OF THE RESPIRATORY SYSTEM, RELATED TO FUNCTION. The Respiratory System. 2010:11–28. doi: 10.1016/B978-0-7020-3370-4.00002-5. Epub 2016 Dec 9. PMCID: PMC7150121.
- Patel S, Jose A, Mohiuddin SS. Physiology, Oxygen Transport And Carbon Dioxide Dissociation Curve. [Updated 2023 Mar 27]. In: StatPearls [Internet]. Treasure Island (FL): StatPearls Publishing; 2024 Jan-. Available from: https://www.ncbi.nlm.nih.gov/books/NBK539815/
- Khan YS, Lynch DT. Histology, Lung. [Updated 2023 May 1]. In: StatPearls [Internet]. Treasure Island (FL): StatPearls Publishing; 2024 Jan-. Available from: https://www.ncbi.nlm.nih.gov/books/NBK534789/
- https://www.slideshare.net/slideshow/mechanism-of-respirationbreathing-mechanismgaseous-exchangeinspiration-and-expiration/253197007
- https://www.embibe.com/exams/mechanism-of-respiration/
- https://gyansanchay.csjmu.ac.in/wp-content/uploads/2023/03/2-MECHANISM-OF-RESPIRATION.pdf
- https://pharmdguru.com/mechanism-of-respiration-and-regulation-of-respiration/
- https://monad.edu.in/img/media/uploads/respiratory%20system.pdf
- https://microbiologynotes.com/respiration-and-respiratory-organs/
- https://www.geeksforgeeks.org/respiratory-system-diagram/
- https://www.vdh.virginia.gov/content/uploads/sites/23/2016/05/AIR-213-2.pdf
- http://www.histology.leeds.ac.uk/respiratory/conducting.php
- https://www.vedantu.com/biology/mechanism-of-breathing
- https://www.slideshare.net/slideshow/histology-of-trachea-and-lung/59562326
- https://www.kgmu.org/download/virtualclass/anatomy/A-RESPIRATORY_SYSTEM_HISTOLOGY-16-12-14.pdf
- https://www2.victoriacollege.edu/dept/bio/belltutorials/histology%20tutorial/respiratory/respiratory%20histology.html
- https://uomus.edu.iq/img/lectures21/MUCLecture_2024_1102928.pdf
- https://www.pw.live/chapter-respiration-class-seven/mechanism-of-breathing-in-human
- https://microbiologynotes.com/mechanism-of-respiration-in-human/
- https://training.seer.cancer.gov/anatomy/respiratory/capacity.html