What is Photosystem I (PS1)?
- Photosystem I (PSI), or plastocyanin–ferredoxin oxidoreductase, is a critical component of oxygenic photosynthesis. This process, vital for life on Earth, involves converting light energy into chemical energy. PSI functions as an integral membrane protein complex, primarily found in algae, plants, and cyanobacteria. It plays a pivotal role in the photosynthetic light reactions by facilitating the transfer of electrons across the thylakoid membrane from plastocyanin to ferredoxin.
- The discovery of PSI predates that of Photosystem II (PSII), though later research revealed that PSII is the first enzyme in the photosynthetic electron transport chain. The initial aspects of PSI were uncovered in the 1950s, but their significance wasn’t fully understood until the 1960s.
- Louis Duysens first proposed the concept of PSI and PSII in 1960, while Fay Bendall and Robert Hill developed a coherent theory of serial photosynthetic reactions that same year. Their hypothesis was confirmed through experiments by the Duysens and Witt groups in 1961.
- Oxygenic photosynthesis, catalyzed by PSI, is a key process for life on Earth, converting light energy into chemical energy and leading to the evolution of oxygen from water. This process is driven by two major protein complexes, PSI and PSII, which work sequentially and are linked by the cytochrome b6f complex.
- PSI specifically catalyzes light-induced transmembrane charge separation, transferring electrons from plastocyanin or cytochrome c6 to ferredoxin. This electrochemical gradient powers the synthesis of adenosine triphosphate (ATP) by ATP synthase, with the electrons ultimately used for the reduction of hydrogen, stored in the form of nicotinamide adenine dinucleotide phosphate (NADPH) and ATP. These products are crucial for CO2 fixation in the Calvin cycle.
- PSI, a complex bio-solar energy converter, consists of 12 proteins and 127 noncovalently bound cofactors, including chlorophylls, carotenoids, FeS clusters, and vitamin K1. It operates as a bio-solar cell, capturing solar energy through a large antenna system and transferring this energy to its core, where electron transfer occurs.
- Upon excitation, an electron is ejected and traverses the membrane to the terminal FeS cluster via a series of electron carriers. The electron is then transferred to ferredoxin and ultimately used to reduce NADP^+ to NADPH by the ferredoxin:NADP^+ reductase (FNR). The cycle completes with the re-reduction of the donor site of PSI by either plastocyanin or cytochrome c6.
- Therefore, PSI’s function is integral to the light reactions of photosynthesis, efficiently converting solar energy into a form usable for biological processes, ultimately sustaining life on Earth.
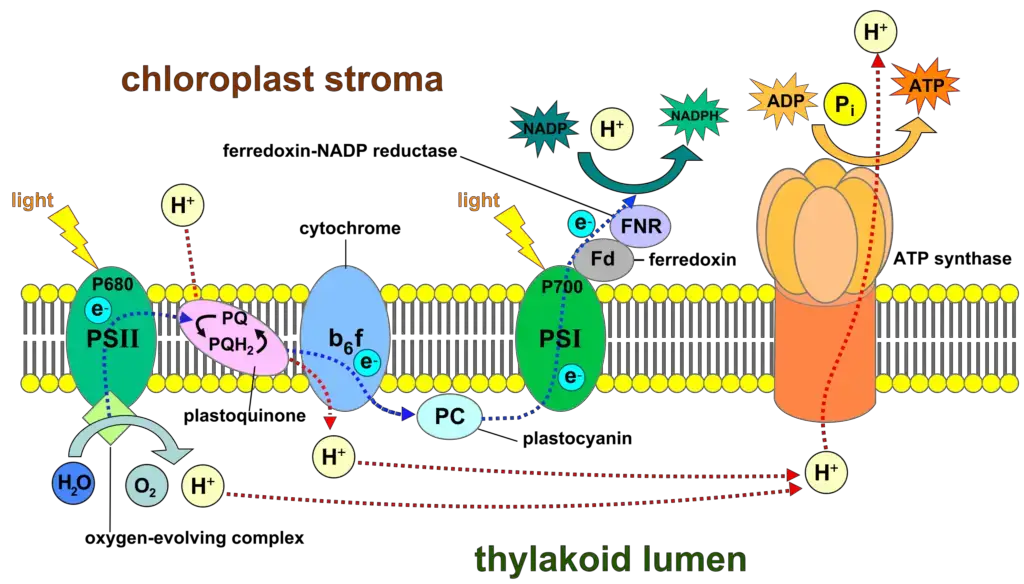
Definition of Photosystem I (PS1)
Photosystem I (PSI) is a crucial protein complex in oxygenic photosynthesis, primarily found in algae, plants, and cyanobacteria. It functions by capturing light energy to drive the transfer of electrons across the thylakoid membrane, from plastocyanin to ferredoxin. This process contributes to the synthesis of ATP and NADPH, which are essential for the Calvin cycle in photosynthesis. PSI is characterized by its complex structure, comprising multiple proteins and cofactors, including chlorophylls and carotenoids, essential for its role in energy conversion.
Properties of Photosystem I (PS1)
Photosystem I (PSI) possesses several distinct properties that are crucial for its role in photosynthesis:
- Composition: PSI is a large membrane protein complex composed of about 12 core proteins and numerous cofactors, including approximately 127 molecules such as chlorophylls, carotenoids, iron-sulfur (FeS) clusters, and vitamin K1. These components are intricately arranged to facilitate its function.
- Light Absorption: PSI has a sophisticated antenna system comprising around 90 chlorophyll molecules and 22 carotenoids. This system efficiently captures light energy, particularly in the red and far-red wavelengths, making PSI highly effective in absorbing sunlight.
- Electron Transport: PSI plays a pivotal role in the electron transport chain of photosynthesis. It facilitates the transfer of electrons from the donor molecules (plastocyanin or cytochrome c6) across the thylakoid membrane to the acceptor molecule ferredoxin. This transfer is achieved through a series of electron carriers within the complex.
- Redox Potential: The primary electron acceptor of PSI has one of the most negative redox potentials among biological systems. This allows for the efficient reduction of NADP+ to NADPH, a crucial step in the photosynthetic process.
- Proton Gradient Formation: The electron transfer activity of PSI contributes to the creation of a proton gradient across the thylakoid membrane. This gradient is essential for ATP synthesis, which occurs via ATP synthase.
- Spatial Orientation: PSI is embedded in the thylakoid membrane of chloroplasts, with specific orientation. Its structure ensures that the electron transfer occurs from the luminal (inner) side of the membrane to the stromal (outer) side, facilitating the movement of electrons to the Calvin cycle.
- Functional Versatility: In addition to its primary role in linear electron flow, PSI can also participate in cyclic electron flow, which is crucial for balancing the ATP/NADPH ratio in cells and protecting the photosynthetic apparatus under stress conditions.
- Regulatory Mechanisms: PSI activity is regulated by various environmental conditions, including light intensity and quality. This ensures that photosynthesis is efficient and adaptive to changing environmental factors.
These properties underscore the complexity and efficiency of PSI as a vital component in the photosynthetic machinery of plants, algae, and cyanobacteria.
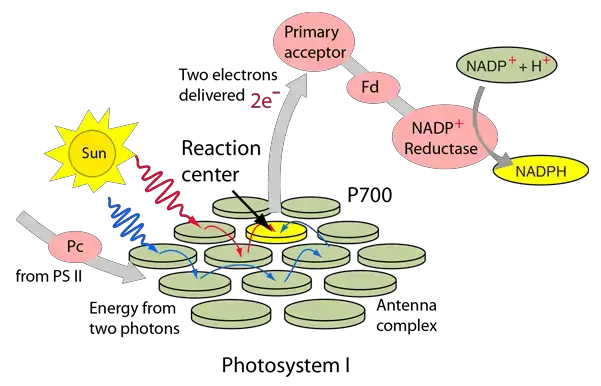
Mechanism of Photosystem I (PS1)
The mechanism of Photosystem I (PSI) involves several steps that are essential for the light-dependent reactions of photosynthesis:
- Photon Absorption: PSI contains pigments, primarily chlorophyll a, that absorb photons of light. This absorption elevates the energy level of electrons within the chlorophyll molecules.
- Charge Separation: When chlorophyll in the PSI reaction center, known as P700, absorbs light, an electron is excited to a higher energy level. This creates a charge separation as the excited electron leaves the P700 molecule, which now has a positive charge (P700+).
- Electron Transport: The excited electron is then transferred to a series of acceptors embedded within PSI. These include chlorophylls, phylloquinones, and a series of iron-sulfur clusters named A0, A1, and Fx.
- Iron-Sulfur Clusters: The electron is passed through a chain of iron-sulfur clusters within the PSI complex, including Fx, Fa, and Fb. These clusters facilitate the transfer of electrons one at a time through the protein.
- Electron Donation to Ferredoxin: The final acceptor in the electron transport chain is ferredoxin (Fd), a soluble iron-sulfur protein. Electrons are transferred to ferredoxin, which then moves away from PSI to carry out further functions.
- NADP+ Reduction: Ferredoxin transfers the electrons to the enzyme ferredoxin-NADP+ reductase (FNR), which catalyzes the reduction of NADP+ to NADPH. This molecule provides the reducing power necessary for the Calvin cycle, which synthesizes sugars from CO2.
- Reoxidation of P700+: Meanwhile, the P700+ molecule is returned to its ground state by accepting an electron from plastocyanin or cytochrome c6, which have obtained their electrons from Photosystem II (PSII) via the cytochrome b6f complex.
- Proton Gradient Formation: Although PSI itself does not pump protons across the thylakoid membrane, it works in conjunction with the cytochrome b6f complex and PSII to establish a proton gradient that is used by ATP synthase to generate ATP.
Components and Structure of PSI
Photosystem I (PSI) is a complex and multifaceted protein complex integral to the process of photosynthesis. Its components and structure can be systematically analyzed as follows:
- Core Subunits PsaA and PsaB: These are two closely related proteins, each comprising 730 to 750 amino acids with 11 transmembrane segments. They play a critical role in binding the vital electron transfer cofactors P700, Acc, A0, A1, and Fx. A unique feature is the [4Fe-4S] iron-sulfur cluster named Fx, which is coordinated by four cysteines – two from each PsaA and PsaB. The arrangement of these cysteines, located in a loop between the ninth and tenth transmembrane segments, is vital for the function of PSI. Additionally, a leucine zipper motif, situated downstream of these cysteines, may contribute to the dimerization of PsaA and PsaB.
- Terminal Electron Acceptors FA and FB: Found in a 9-kDa protein called PsaC, these also consist of [4Fe-4S] iron-sulfur clusters and are located close to the PsaA/PsaB core near FX.
- Additional Protein Subunits: Each subunit of PSI has a specific role, such as PsaD, which is required for assembly and helps bind ferredoxin; PsaE; PsaI, which may stabilize PsaL and the light-harvesting complex II; and others like PsaJ, PsaK, PsaL, PsaM, and PsaX.
- Cytochrome b6f Complex: This is a soluble protein that plays a role in the electron transport chain.
- Lipid Components: PSI includes various lipids like Monogalactosyldiglyceride (MGDG) and different forms of Phosphatidylglycerol (PG), which contribute to the stability and function of the complex.
- Pigments: The antenna complex of PSI contains numerous pigment molecules, including Chlorophyll a and β-Carotene, crucial for light absorption and energy transfer.
- Coenzymes and Cofactors: These include QK-A and QK-B (forms of vitamin K1 phylloquinone), which are early electron acceptors in the electron transport chain, and the enzyme Ferredoxin-NADP+ oxidoreductase (FNR), along with essential ions like Ca2+ and Mg2+.
- Antenna Complex: This is composed of chlorophyll and carotenoids mounted on proteins, absorbing light within the visible spectrum. The number of these pigment molecules varies among different organisms.
- P700 Reaction Center: This comprises modified chlorophyll a, which absorbs light at 700 nm. It uses the energy from photons to excite electrons to a higher energy state, facilitating their movement in an oxidation/reduction process.
- Modified Chlorophyll A0 and A1: These molecules are early electron acceptors in PSI, accepting electrons from P700* and passing them through the electron transport chain.
- Phylloquinone: Also known as vitamin K1, phylloquinone acts as an electron acceptor, playing a crucial role in the electron transport process within PSI.
- Iron–Sulfur Complex: This includes the iron-sulfur reaction centers Fx, Fa, and Fb, which serve as electron relays in PSI.
- Ferredoxin: This soluble protein transfers electrons to the enzyme ferredoxin–NADP+ reductase.
- Ferredoxin–NADP+ Reductase (FNR): This enzyme completes the reduction of NADP+ to NADPH by receiving an electron from reduced ferredoxin.
- Plastocyanin: As an electron carrier, plastocyanin transfers electrons from the cytochrome b6f complex to the P700 cofactor of PSI.
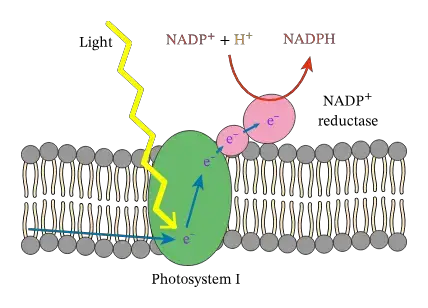
Components of PSI (protein subunits, lipids, pigments, coenzymes, and cofactors).
Photosystem I (PSI) is a complex and multifaceted component of the photosynthetic machinery, comprised of various protein subunits, lipids, pigments, coenzymes, and cofactors. Each element plays a specific role in the overall function of PSI:
- Protein Subunits:
- PsaA and PsaB: These are large transmembrane proteins involved in the binding of the core electron transfer cofactors such as P700, A0, A1, and Fx. They are part of the photosynthetic reaction center protein family and are essential for the electron transport process.
- PsaC: This subunit contains the iron-sulfur center and acts as an apoprotein for the Fa and Fb clusters.
- PsaD: Essential for PSI assembly, PsaD also aids in binding ferredoxin.
- PsaE, PsaI, PsaJ, PsaK, PsaL, PsaM, PsaX: These subunits have various roles in stabilizing PSI, assisting in light-harvesting, and facilitating electron transport.
- Cytochrome b6f Complex: A soluble protein, it is instrumental in the electron transport chain (ETC).
- Fa, Fb, and Fx: Located within PsaC and PsaAB, these are part of the ETC, transferring electrons within the system.
- Ferredoxin: This acts as an electron carrier within the ETC.
- Plastocyanin: Another soluble protein, plastocyanin transfers electrons to the P700 cofactor of PSI.
- Lipids:
- Various forms of lipids such as Monogalactosyldiglyceride (MGDG) and Phosphatidylglycerol (PG) are present, contributing to the structural integrity and functional efficiency of PSI.
- Pigments:
- Chlorophyll a: Present in large numbers (90 molecules) in the antenna system and a few (5 molecules) in the ETC, Chlorophyll a plays a critical role in light absorption and energy transfer.
- Chlorophyll a0 and a′: Modified forms of Chlorophyll a, these are involved in early electron acceptance within the ETC.
- β-Carotene: This pigment, with 22 molecules, aids in light absorption and protection against oxidative stress.
- Coenzymes and Cofactors:
- QK-A and QK-B: Forms of vitamin K1 phylloquinone, these serve as early electron acceptors in the ETC.
- Ferredoxin-NADP+ Oxidoreductase (FNR): This enzyme plays a crucial role in the reduction of NADP+ to NADPH.
- Calcium (Ca2+) and Magnesium (Mg2+) Ions: These ions are vital for the structural stability and functional activity of PSI.
Tertiary structure of the PSI
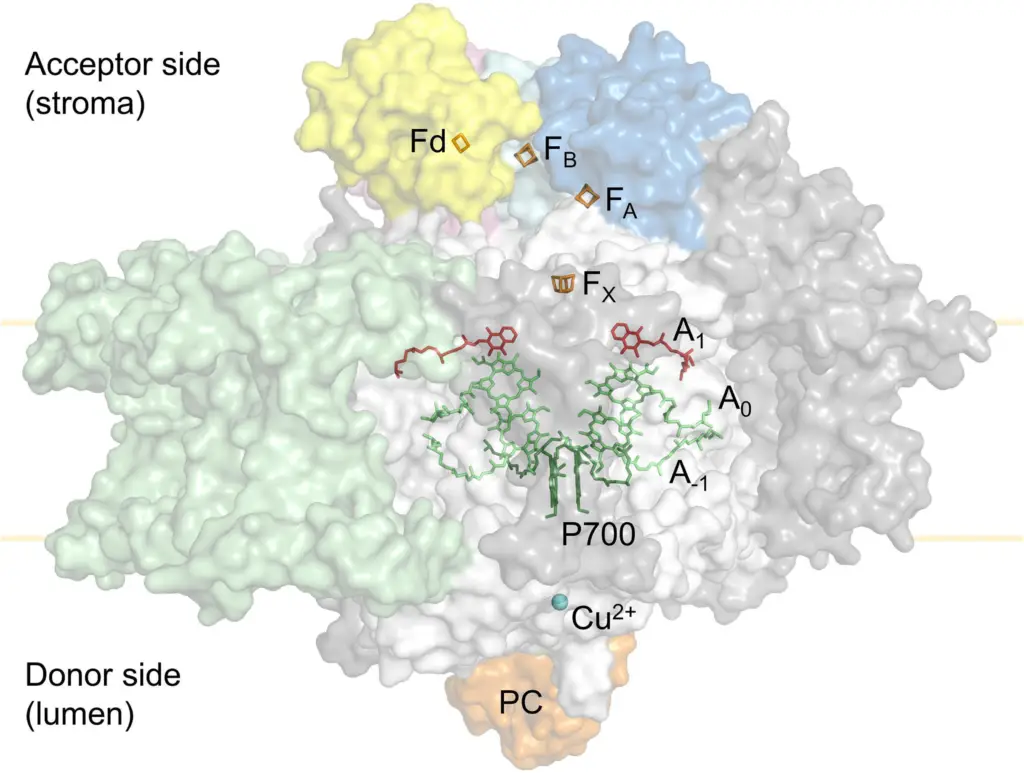
The tertiary structure of the Photosystem I (PSI) complex is a multi-subunit complex with a distinct arrangement of proteins and cofactors that facilitate electron transport during photosynthesis.
- Core Protein Subunits: The core of the PSI complex is composed of subunits PsaA and PsaB, depicted in white in the image. These subunits form a central heterodimer around which the entire complex is organized.
- Additional Subunits: PsaC, shown in cyan, is bound to the stromal side of PSI and coordinates the iron-sulfur clusters FA and FB. PsaD (blue) and PsaE (pink) are also present, along with the light-harvesting complex I (LHCI) subunits, which are illustrated in green. Other non-core PSI subunits are colored grey.
- Electron Donor and Acceptor: Plastocyanin (PC), shown in orange, is located at the PSI donor side (lumenal side). It donates electrons to PSI, specifically to the P700 chlorophyll pair after absorbing light energy. On the acceptor side (stromal side), ferredoxin (Fd), depicted in yellow, accepts electrons from PSI.
- Cofactors and Electron Transport Path: The cofactors involved in electron transport are highlighted, with the copper ion (Cu2+) at the plastocyanin active site shown in blue. The primary electron donor within PSI, P700, is displayed in dark green. The early electron acceptors, the A–1 and A0 chlorophylls, are shown in lime, and the A1 phylloquinone, which is the next electron acceptor in the sequence, is in red.
- Iron-Sulfur Clusters: The PSI complex contains iron-sulfur clusters that play a critical role in electron transfer. These clusters, FX, FA, and FB, are shown in orange and are involved in the final steps of electron transfer within PSI before the electron is passed to ferredoxin. The 2Fe-2S cluster of ferredoxin is also depicted in orange, indicating its role in receiving and transferring electrons outside of PSI to the ferredoxin:NADP+ reductase (FNR) for the production of NADPH.
- Spatial Arrangement: The spatial orientation of these components is carefully arranged to facilitate efficient electron transfer. The P700 chlorophyll pair is strategically placed to receive electrons from plastocyanin and pass them along through a series of cofactors embedded in the protein matrix until they reach ferredoxin.
Photosystem I Photoinhibition Mechanisms
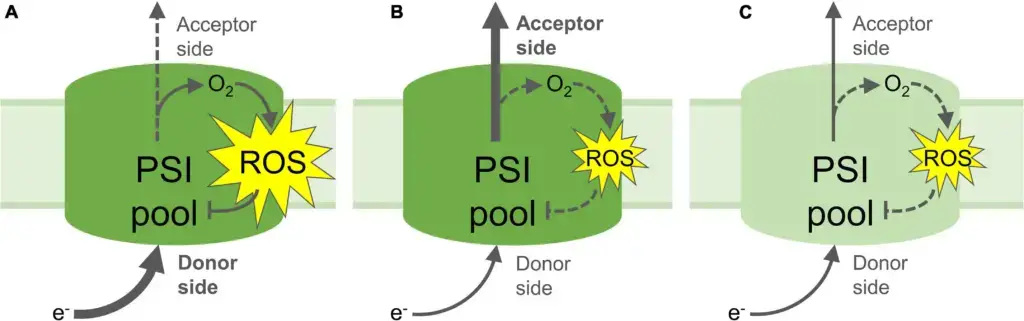
- Photoinhibition Mechanisms:
- Electron Flow Imbalance (Panel A): When there is an increased electron flow to PSI’s donor side, or a decreased flow from the acceptor side, an imbalance occurs. This leads to the reduction of O2 and the subsequent formation of reactive oxygen species (ROS). The ROS generated can result in the inactivation of part of the PSI pool. The inactivation occurs because the excess electrons, which cannot be properly utilized, interact with oxygen to form ROS, causing damage to the PSI components.
- Photoprotection Mechanisms:
- Down-regulation of Electron Flow to PSI (Panel B): Mechanisms such as non-photochemical quenching, photosynthetic control, or PSII inactivation can down-regulate the flow of electrons to PSI’s donor side. By limiting the electron flow, the production of ROS is curtailed, thus protecting PSI from photoinhibition. Additionally, upregulated electron acceptor capacity in the stroma, possibly due to increased CO2 fixation or photorespiration, can enhance the flow of electrons away from PSI, further preventing ROS formation and providing photoprotection.
- Partial Inactivation of PSI Pool (Panel C): Partial inactivation of the PSI pool by photoinhibition can create a feedback loop that down-regulates the transport of electrons to the acceptor side. This self-limiting mechanism prevents further PSI photoinhibition and reduces the rate of electron-consuming reactions in the stroma, which includes the formation of ROS and the initiation of signaling pathways. The pale green color of the PSI pool denotes partial inactivation due to ROS-induced damage to FeS clusters and/or protein components.
Photosystem I (PSI) photoinhibition occurs when the photosynthetic apparatus is subjected to excess light energy that exceeds the processing capacity of the chloroplast, leading to the production of reactive oxygen species (ROS) and potential damage to the photosystem. The following outlines the mechanisms, impact, and recovery associated with PSI photoinhibition:
- Mechanisms of Photoinhibition:
- Excess light energy can cause over-reduction of PSI, leading to the transfer of excess electrons to O2, thereby generating ROS such as superoxide (O2•‒) and hydrogen peroxide (H2O2).
- The interaction between H2O2 and the FeS clusters at the acceptor side of PSI can lead to the formation of the hydroxyl radical (•OH), which can inactivate PSI electron transport.
- Damage to PSI can also be caused by the ROS attacking protein subunits, lipid membranes, and metabolites.
- Impact of Photoinhibition:
- Photoinhibition can lead to a decrease in photosynthetic capacity, affecting plant growth and crop yield.
- Environmental stresses such as low temperature, drought, and salinity can exacerbate PSI photoinhibition by limiting CO2 assimilation and overwhelming the electron sink capacity.
- Red and blue light, which preferentially excite PSII, can intensify PSI photoinhibition compared to white and green light.
- Recovery from Photoinhibition:
- The recovery of PSI activity after photoinhibition is a slow process, often taking several days, in contrast to the rapid repair mechanisms of PSII.
- Recovery involves the potential degradation and rebuilding of the entire PSI complex, which is a more complex process compared to the dedicated repair cycle of PSII.
- Decreased abundance of PSI subunit proteins, particularly PsaA and PsaB, indicates lasting PSI inhibition, while electron transport measurements suggest a slightly quicker recovery.
- CO2 assimilation rates tend to recover more rapidly than PSI activity, suggesting that electron consumption in the chloroplast may be partly independent of PSI activity.
- Higher light intensity can enhance the activity of the partially inactive PSI pool, indicating that PSI activity can be increased through excitation from the light-harvesting complex II (LHCII) or activation of reserve PSI complexes.
- Adaptation Mechanisms:
- It has been suggested that photoinhibited PSI centers may not require complete replacement, as thermal dissipation of excitation energy from LHCII via oxidized P700+ can occur in these centers.
Therefore, PSI photoinhibition represents a complex interplay between environmental factors and the intrinsic protective and repair mechanisms of the photosynthetic machinery. Understanding these interactions is crucial for improving plant resilience to stress and optimizing photosynthetic efficiency.
Electron donors of PS1: plastocyanin and cytochrome c6
In the process of photosynthesis, Photosystem I (PSI) can receive electrons from two distinct electron donors: plastocyanin and cytochrome c6. The utilization of these two donors in Chlamydomonas, a model organism, exemplifies the versatility in electron transport mechanisms in oxygenic phototrophs.
- Plastocyanin: This is a small, acidic, blue copper protein commonly found in most oxygenic phototrophs. Its structure, as revealed by X-ray crystallography to a resolution of 1.5 Å, consists of an antiparallel β-barrel with a single copper atom. The coordination of this copper atom is facilitated by two imidazole nitrogens (His37 and His87), a thioether sulfur (Met92), and thiolate sulfur (Cys84). The unique polypeptide structure of plastocyanin distorts the copper’s coordination environment away from ideal tetrahedral geometry, thereby stabilizing the Cu(I) form. This structural feature contributes to plastocyanin’s high reduction potential, approximately 370 mV.
- Cytochrome c6: The structure of Chlamydomonas chloroplast cytochrome c6 has also been determined through X-ray diffraction, revealing a typical (class I) cytochrome c6 structure. It comprises four α-helices connected by short loops with tight turns and a very short β-sheet, which may play a role in the oligomerization of cyt c6 in vivo. This is suggested by crystal forms that display oligomerization with contacts near the heme crevice. Cytochrome c6 shares a similar midpoint potential with plastocyanin.
- Functional Interchangeability: Although plastocyanin and cytochrome c6 differ significantly in their structures and electron transfer cofactors, they have almost identical reduction potentials. Their overall sizes (about 10 kD), shape, charge distribution, and surface polarity patterns are also remarkably similar, allowing PSI to dock with either of them efficiently. This structural and functional similarity facilitates their interchangeable roles as electron donors to PSI, depending on environmental conditions such as copper availability.
- Adaptability in Different Organisms: Chlamydomonas, along with many other green algae, represents an intermediate stage in the evolution of photosynthetic organisms. While photosynthetic bacteria predominantly use a type of cytochrome c as the electron donor to their photosynthetic reaction center, higher plants typically use plastocyanin exclusively. Most cyanobacteria can use either a plastocyanin or a cytochrome c6, showing adaptability in their electron transport systems.
Electron acceptor of PS1: ferredoxin
Ferredoxin serves as the primary electron acceptor in Photosystem I (PSI), playing a crucial role in the photosynthetic electron transport chain.
- Structure and Function of Ferredoxin: Ferredoxin is a small protein, consisting of 94 amino acid residues, and contains an Fe2S2 cluster. In the chloroplast, it functions as an electron donor to two key components: NADP (via ferredoxin-NADP reductase) and thioredoxin (via ferredoxin-dependent thioredoxin reductase). These processes are essential for the synthesis of NADPH and the regulation of enzymatic activity through the thioredoxin system, both of which are critical for the Calvin cycle and other cellular functions.
- Characteristics of Chlamydomonas Ferredoxin: The ferredoxin isolated from Chlamydomonas has been purified and sequenced, showing typical characteristics of chloroplast ferredoxins found in other species. Although a crystal structure of this particular ferredoxin has not been determined, homology modeling has provided insights into its probable structure.
- Flavodoxin as an Alternative Electron Acceptor: In some species of cyanobacteria, a flavoprotein named flavodoxin can act as an alternative electron acceptor under conditions of iron limitation. Flavodoxin, like ferredoxin, plays a role in transferring electrons but is employed when iron availability is low, demonstrating the adaptability of the photosynthetic apparatus to varying environmental conditions.
- Chlamydomonas and Flavodoxin: Within the Chlamydomonas genome, there are potentially four flavodoxin-like genes. However, evidence to date does not indicate that flavodoxin acts as an electron acceptor for PSI in Chlamydomonas. This points to a specificity in the electron transfer mechanisms in different species, with Chlamydomonas relying primarily on ferredoxin.
The Electron Transfer Chain of PS1
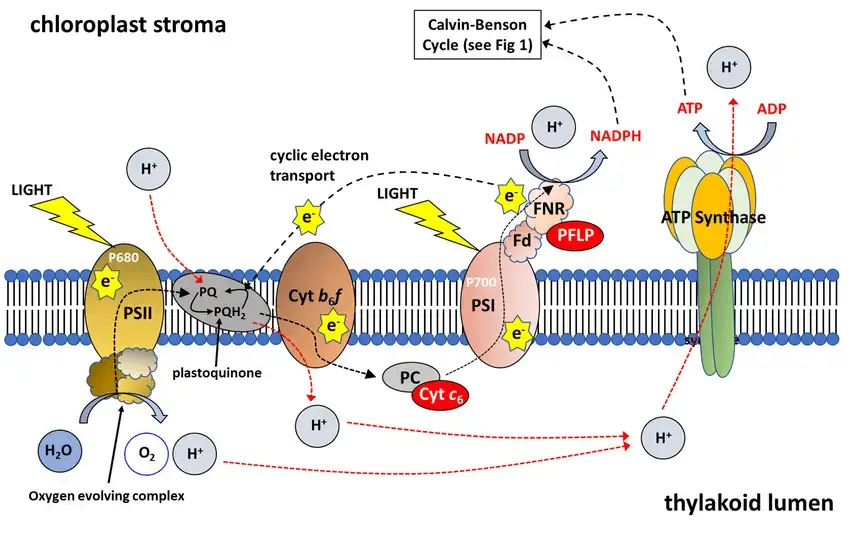
The electron transfer chain of Photosystem I (PSI) is a highly sophisticated and conserved mechanism in plants and cyanobacteria, consisting of a series of cofactors and subunits that facilitate electron transport. This process can be detailed as follows:
- Composition and Cofactor Arrangement: The electron transport chain of PSI includes six chlorophylls, two phylloquinone molecules, and three [4Fe-4S] clusters. These organic cofactors are arranged into two branches, namely the A- and B-branches. This bifurcation is based on the coordination of the cofactors by the two large subunits, PsaA and PsaB, with a twofold axis running through the first FeS cluster FX.
- Primary Charge Separation and P700: The electron transfer begins with primary charge separation, initiated when the antenna chlorophylls transfer excitation energy to P700, exciting it to P700. P700 likely comprises the first pair of chlorophylls, with one chlorophyll a molecule in the B-branch and a C13 epimer of chlorophyll a in the A-branch. However, there is evidence suggesting that P700 may be delocalized over more chlorophylls, and charge separation could potentially start from one of the accessory chlorophylls.
- Electron Transfer Pathways: Electrons are transferred along either the A- or B-branch through a chain of electron carriers, including the second and third pairs of chlorophylls, phylloquinones, and the FeS cluster FX. This electron transfer concludes at the terminal FeS clusters FA and FB, coordinated by PsaC, and the electron is then transferred to ferredoxin.
- Branch Activity and Electron Transfer Rates: Both the A- and B-branches are active in electron transfer, but their usage varies between organisms. The transfer from phylloquinones to FX is considered the rate-limiting step in PSI. The B-branch electron transfer from phylloquinone to FX is notably faster than the A-branch. The differential rates are hypothesized to be influenced by the presence of different lipids near the electron transport chain.
- FX Cluster and Its Dual Coordination: The first FeS cluster FX is unique as it is a 4Fe-4S cluster coordinated by two different proteins, PsaA and PsaB. Besides its role in electron transfer, FX also significantly contributes to the assembly of PSI.
- Structural Asymmetry and Electron Gating: The pair of chlorophylls assigned to P700 exhibits chemical and environmental asymmetry, possibly influencing the redox potential and distribution of the unpaired electron. This asymmetry might play a role in gating electrons along the two branches, although this has not been experimentally proven.
- Unusual Coordination of Chlorophylls: The coordination of chlorophylls in PSI, particularly “A” and “A0”, is noteworthy. They are not coordinated by histidine but involve unique interactions with water molecules and methionine, respectively. This unusual coordination is crucial for the function and efficiency of electron transport within PSI.
- Differences in Activation Energy Barrier: The difference in activation energy barriers between the A- and B-branches is attributed to the presence of different lipids. A negatively charged phospholipid on the A-branch and a neutral galactolipid on the B-branch may explain the variance in electron transfer rates.
Steps of The Electron Transfer Chain of PS1
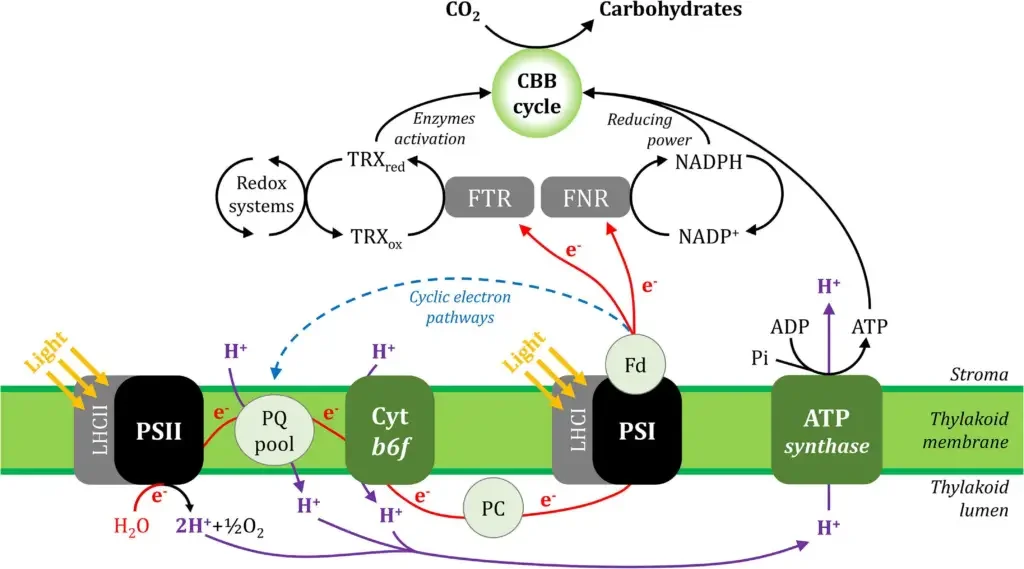
- Electron Donation to PSI: Electrons are donated to PSI by plastocyanin (PC), a small copper-containing protein that transfers electrons from the cytochrome b6f complex (Cyt b6f) to PSI.
- Absorption of Light: PSI captures photons of light through its light-harvesting complex I (LHCI), which boosts the energy level of the electrons within the PSI reaction center.
- Primary Charge Separation: The energized electrons are transferred to the reaction center chlorophyll of PSI, known as P700, leading to its primary charge separation and the formation of P700+.
- Electron Transport within PSI: The excited electrons are then passed through a series of internal electron carriers within PSI. These carriers include chlorophyll molecules, phylloquinones, and an iron-sulfur cluster called FX.
- Transfer to Ferredoxin: The electrons are eventually transferred to ferredoxin (Fd), a small iron-sulfur protein, which is the final electron acceptor of PSI.
- Reduction of NADP+: Ferredoxin then interacts with ferredoxin:NADP+ oxidoreductase (FNR) to facilitate the transfer of electrons to NADP+, reducing it to NADPH. This reaction provides reducing power for the Calvin-Benson-Bassham (CBB) cycle for the synthesis of carbohydrates.
- Cyclic Electron Transport: A portion of the electrons can be cycled back from ferredoxin to the cytochrome b6f complex, represented by the blue dashed line. This cyclic electron flow allows the generation of additional ATP without the production of NADPH.
- Proton Flux: Concurrently, the movement of electrons through the electron transport chain, particularly through the cytochrome b6f complex, contributes to a proton gradient across the thylakoid membrane, illustrated by purple lines. The protons accumulate within the thylakoid lumen.
- ATP Synthesis: The proton gradient drives the synthesis of ATP from ADP and inorganic phosphate (Pi) by ATP synthase. The ATP produced is then utilized in the CBB cycle and other cellular processes.
- CO2 Assimilation in the CBB Cycle: The ATP and NADPH generated by the light-dependent reactions are used to assimilate CO2 in the CBB cycle, leading to the production of carbohydrates.
The Antenna System
The antenna system of Photosystem I (PSI) is a complex and highly efficient structure, crucial for capturing light energy and transferring it to the reaction center of PSI. This system can be detailed as follows:
- Core-Antenna System in Cyanobacteria: Comprised of 90 chlorophyll a molecules and 22 carotenoids per monomer, the core-antenna system of PSI in cyanobacteria forms a tightly interconnected network across the three monomers in the PSI trimer. This interconnectedness facilitates efficient transfer of excitation energy not only within a single monomer but also between monomers. The chlorophylls are arranged in a clustered network, with each chlorophyll located within 15 Å of its neighbors, ensuring high efficiency in excitation energy transfer.
- Role of Carotenoids: Carotenoids serve dual functions in the antenna system. They act as additional antenna pigments, aiding in light capture, and play a crucial role in photoprotection. By quenching dangerous triplet states of chlorophylls and dissipating excess energy as heat, carotenoids prevent the formation of reactive oxygen species, thereby protecting the photosynthetic apparatus from damage.
- Quantum Efficiency: The excitation energy transfer from the antenna to P700 is extraordinarily efficient, with a quantum efficiency of greater than 99.9% at room temperature. This efficiency is a testament to the highly conserved nature of the core-antenna system over evolutionary time.
- Structure and Evolution: Most chlorophylls of the core-antenna system are coordinated by the large subunits PsaA and PsaB. It is believed that these subunits evolved from a fusion of an antenna protein with a protein coordinating the electron transfer chain. This fusion has resulted in a highly efficient antenna system in PSI, where the reaction center (RC) and the antenna system are jointly organized and functionally intertwined.
- Stabilization by Small Subunits: Small subunits like PsaI, PsaJ, PsaK, PsaL, and PsaX play a pivotal role in stabilizing the core-antenna system and facilitating interactions with peripheral antenna systems. Additionally, specific phospholipids in PSI also coordinate an antenna chlorophyll.
- Antenna System in Plants: In plants, PSI typically forms a monomeric complex with four tightly bound peripheral antenna proteins: Lhca1, Lhca2, Lhca3, and Lhca4. The PSI–LHCI complex contains a total of 173 chlorophylls, distributed among the core of PSI, the LHCI proteins, and the interface between the LHCI antenna proteins and the core-antenna system of PSI.
- Peripheral Antenna Systems in Cyanobacteria: Most cyanobacteria possess genes for two different peripheral antenna systems. Phycobilisomes serve as peripheral antenna systems under nutrient-rich conditions, while a membrane intrinsic antenna system consisting of rings of the IsiA protein surrounds the PSI trimer under iron deficiency, dramatically increasing the antenna size.
- Functional Architecture of the Antenna System: The antenna chlorophylls in PSI are uniquely arranged to capture light and transfer excitation energy efficiently to the center of the complex, where electron transfer occurs. Unlike symmetric arrangements found in other light-harvesting systems, the chlorophylls in PSI form a clustered network, allowing multiple pathways for efficient energy transfer.
- Carotenoids’ Protective Role: Carotenoids in PSI perform essential structural roles and function as additional antenna pigments. Their critical role in preventing damage from overexcitation, particularly in high-light conditions, is achieved by quenching chlorophyll triplet states and dissipating energy as heat.
Functions of Photosystem I (PS1)
Photosystem I (PSI) plays several vital roles in the process of photosynthesis, particularly in the light-dependent reactions. Its key functions include:
- Light Absorption and Charge Separation: PSI contains a complex array of pigments, primarily chlorophyll molecules, which absorb light energy. This energy is used to excite electrons within the chlorophyll molecules, leading to primary charge separation. This step is crucial in converting light energy into a form that can be used in the biochemical processes of the cell.
- Electron Transport: PSI is an essential component of the photosynthetic electron transport chain. It accepts electrons from the cytochrome b6f complex through plastocyanin or cytochrome c6 and then transfers these electrons, via a series of internal redox reactions, to its primary electron acceptor, ferredoxin.
- NADP+ Reduction: One of the most critical roles of PSI is facilitating the reduction of NADP+ to NADPH. This is achieved through the ferredoxin-NADP+ reductase enzyme, which uses electrons transferred from PSI to reduce NADP+. NADPH is a key reducing agent used in the Calvin cycle for carbon fixation, leading to the synthesis of sugars.
- Formation of Proton Gradient: The movement of electrons through PSI and the associated electron transport chain contributes to the formation of a proton gradient across the thylakoid membrane. This gradient is crucial for ATP synthesis.
- ATP Synthesis: The proton gradient created partly by the activity of PSI is utilized by ATP synthase to produce ATP. This process, known as photophosphorylation, is essential for providing the energy required for various cellular processes, including the Calvin cycle.
- Protection Against Photodamage: PSI plays a role in protecting the photosynthetic apparatus from photodamage, especially under conditions of excess light. It can participate in alternative electron flow pathways, such as cyclic electron flow, which helps in dissipating excess energy and protecting the chloroplast from oxidative stress.
- Regulation and Signaling: PSI is involved in various regulatory and signaling pathways within the cell. Its activity can be modulated based on environmental conditions, and it can influence the expression of genes related to photosynthesis and stress responses.
References
- Jensen PE, Bassi R, Boekema EJ, Dekker JP, Jansson S, Leister D, Robinson C, Scheller HV. Structure, function and regulation of plant photosystem I. Biochim Biophys Acta. 2007 May;1767(5):335-52. doi: 10.1016/j.bbabio.2007.03.004. Epub 2007 Mar 15. PMID: 17442259.
- Hilary Evans, E. Photosystem 1 preparations from dark-and light-grown cells of the cyanobacterium, Chlorogloea fritschii . Photosynth Res 1, 259–264 (1981). https://doi.org/10.1007/BF00034269
- Yordanov, Ivan & Velikova, Violeta. (2000). Photoinhibition of photosystem I. Bulg J Plant Physiol. 26.
- Redding, Kevin E. (2009). The Chlamydomonas Sourcebook || Photosystem I. , (), 541–572. doi:10.1016/b978-0-12-370873-1.00023-x
- Fromme, Petra (2004). Encyclopedia of Biological Chemistry || Photosystem I, Structure and Function. , (), 342–347. doi:10.1016/b0-12-443710-9/00493-2
- Antonkine, Mikhail L. (2004). Encyclopedia of Biological Chemistry || Photosystem I: FX, FA, and FB Iron–Sulfur Clusters. , (), 348–356. doi:10.1016/b0-12-443710-9/00489-0
- Leegood, R.C. (2013). Encyclopedia of Biological Chemistry || Photosynthesis. , (), 492–496. doi:10.1016/B978-0-12-378630-2.00049-9
- Author(s). (Year). Title of the chapter. In Editor(s) (Eds.), Title of the book (pp. pages of the chapter). Publisher. https://doi.org/10.1016/B978-0-12-378630-2.00287-5