What is NMR Spectroscopy (Nuclear Magnetic Resonance Spectroscopy)?
NMR spectroscopy, commonly known as Magnetic Resonance Spectroscopy (MRS), is a strong analytical method used to analyze the local magnetic fields around atomic nuclei. It is based on the absorption of electromagnetic radiation by atom nuclei in the radiofrequency area, which generally ranges from 4 to 900 MHz.
Over the last several decades, NMR spectroscopy has emerged as a major tool for identifying the structure of organic molecules. It is uncommon among spectroscopic approaches in that it is normally required to analyze and interpret the full spectrum.
The NMR spectroscopy concept consists of three main steps:
- Magnetic nuclear spin alignment: The sample is immersed in a constant magnetic field (B0), which aligns the magnetic nuclear spins.
- Spin alignment perturbation: A weak oscillating magnetic field, known as a radio-frequency (RF) pulse, is used to disturb the nuclear spin alignment.
- Detection and analysis of released electromagnetic waves: As a result of the disturbance, the sample produces electromagnetic waves, which are detected and evaluated.
The electrical structure, kinetics, reaction state, and chemical environment of molecules are all revealed by NMR spectroscopy. It is frequently employed in a variety of domains such as organic chemistry, biochemistry, and materials science.
NMR spectroscopy is a reliable approach for detecting monomolecular organic molecules in organic science. It provides distinct and tractable spectra capable of distinguishing different functional groups and providing vital insights into molecule structure and interactions. NMR spectroscopy of protons and carbon-13 is among the most regularly used methods, however NMR may be applied to any substance containing nuclei with spin.
NMR spectra are well-resolved, predictable, and provide useful information for even tiny compounds. For chemical identification, they have virtually supplanted traditional wet chemistry experiments. NMR spectroscopy, on the other hand, takes a rather substantial amount of purified chemical, often 2-50 mg, however the sample is frequently recovered. Because solid-state NMR analysis needs specialist equipment, it is better to dissolve the sample in a solvent.
Correlation spectroscopy, such as two-dimensional (2D) NMR, allows for the study of correlated resonances and makes it easier to identify nearby substituents. More advanced 3D and 4D NMR technologies are also available to enhance or suppress certain resonances. NOE spectroscopy, which observes the relaxation of resonances, permits the creation of three-dimensional models of molecules based on nuclei closeness.
NMR spectrometers are relatively costly, ranging from hundreds of thousands to millions of dollars. To attain great resolution, they use strong liquid helium-cooled superconducting magnets. However, for specific applications, less priced devices with permanent magnets are available. There are even tabletop NMR spectrometers with lower resolution but enough performance for specialized needs.
In summary, NMR spectroscopy is a flexible and strong analytical method utilized for structural characterization, chemical identification, and molecular interaction study. Its capacity to offer extensive information on the structure and behavior of molecules makes it a valuable tool in a wide range of scientific domains.
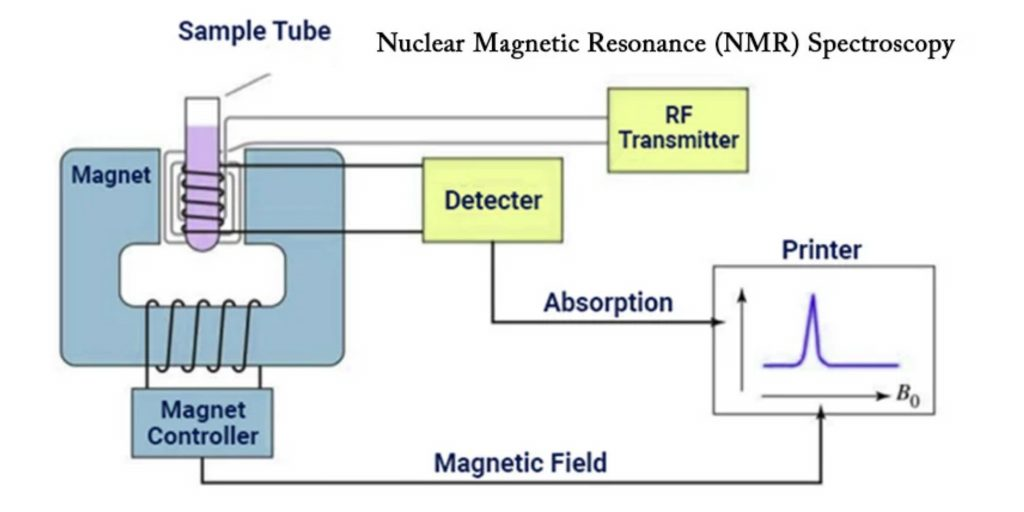
Basis of NMR Spectroscopy
The detection and study of the behavior of atomic nuclei in a magnetic field is the foundation of Nuclear Magnetic Resonance (NMR) spectroscopy. NMR was initially seen experimentally by Felix Bloch at Stanford University and Edward Purcell at Harvard University in 1945. Their pioneering work paved the way for the advancement of NMR spectroscopy as a potent analytical method.
NMR spectroscopy is extremely useful in organic chemistry. It has transformed organic lab research by delivering critical information on the structure, composition, and purity of molecules. One of the most frequent NMR methods in organic chemistry is proton (1H) NMR.
The behavior of protons (nuclei) in a molecule is investigated using NMR spectroscopy. Protons behave differently depending on their chemical surroundings, such as the existence of nearby atoms or functional groups. This behavioral variance enables the understanding of the molecular structure.
When a magnetic field is applied to a sample, the protons in the molecule align with the field. The protons can be disturbed and identified by using radiofrequency pulses. Protons’ absorption and emission of energy at certain frequencies, known as resonance frequencies, give important information about their chemical environment and interactions.
Researchers may discover the structural properties of the molecule by analyzing the NMR signals collected from the sample, including the connectivity of atoms and the types of functional groups present. Furthermore, NMR spectroscopy allows for the measurement of component concentrations as well as the evaluation of sample quality.
Felix Bloch and Edward Purcell were awarded the Nobel Prize in Physics in 1952 for their pioneering contributions to Nuclear Magnetic Resonance Spectroscopy. Since then, NMR spectroscopy has become an indispensable technique for organic chemists, allowing them to get in-depth knowledge of molecule structures and characteristics.
Principle of NMR Spectroscopy
The behavior of atomic nuclei with spin and electric charge in the presence of an external magnetic field is the basis for Nuclear Magnetic Resonance (NMR) spectroscopy. Energy transfers between lower energy states (base energy) and higher energy levels can occur when a sample is put in a magnetic field.
The sample’s nuclei can absorb energy from the external magnetic field and move to higher energy levels. This energy transfer occurs at a certain wavelength associated with radio frequencies. When the nuclei return to their base energy level, they radiate energy at the same frequency that was absorbed.
The radiated energy, or signal, is measured and processed to provide an NMR spectrum tailored to the nucleus under investigation. The spectrum reveals information about the chemical environment and nuclear interactions in the sample.
In summary, the absorption and emission of energy by atomic nuclei with spin and electric charge when exposed to an external magnetic field is the basis of NMR spectroscopy. NMR spectroscopy gives significant information on the structure, dynamics, and chemical characteristics of molecules by studying the resultant signals.
Working of Nuclear Magnetic Resonance (NMR) Spectroscopy
Nuclear Magnetic Resonance (NMR) spectroscopy is based on magnetic field concepts and the behavior of atomic nuclei. The operation of NMR spectroscopy entails many critical steps:
- Sample Preparation: The sample of interest is prepared, which is commonly an organic substance dissolved in a suitable solvent. Atomic nuclei having spin, such as protons (1H) or carbon-13 (13C), should be present in the sample.
- Magnetic Field Application: A strong magnetic field created by a superconducting magnet is applied to the prepared sample. The magnetic field in the sample aligns the atomic nuclei.
- Radiofrequency Excitation: The sample is excited using radiofrequency (RF) pulses. These RF pulses have frequencies that correspond to the resonance frequencies of atomic nuclei in a magnetic field. The radiofrequency pulses are generally in the hundreds of megahertz (MHz) to gigahertz (GHz) range.
- Nuclear Magnetic Resonance and Signal Detection: Nuclear magnetic resonance occurs when RF pulses induce atomic nuclei to move from a lower energy state to a higher energy state. When the RF pulse is terminated, the nuclei revert to their original condition and release energy at the same resonance frequency. This released energy is recognized as an NMR signal by sensitive radio receivers.
- Signal Analysis: The obtained NMR signals are processed and analyzed to retrieve useful sample information. The signal’s resonance frequencies and intensities reveal information on the electronic structure of the molecules and their distinct functional groups. Chemical changes caused by intramolecular magnetic field effects are utilized to detect and differentiate distinct molecules. NMR spectroscopy may also offer extensive information on a molecule’s structure, dynamics, reaction state, and chemical environment.
- Types of NMR: Proton (1H) NMR and carbon-13 (13C) NMR spectroscopy are the two most frequent forms of NMR spectroscopy. These procedures are aimed at analyzing hydrogen and carbon nuclei, respectively. NMR spectroscopy, on the other hand, is applicable to any sample containing nuclei with spin, allowing the investigation of elements such as nitrogen, phosphorus, and fluorine.
In brief, NMR spectroscopy works by exposing the sample to a strong magnetic field, activating the atomic nuclei with radiofrequency pulses, and measuring the energy signals released. The resultant NMR spectra include useful information on the structure, composition, dynamics, and chemical characteristics of the molecules under investigation.
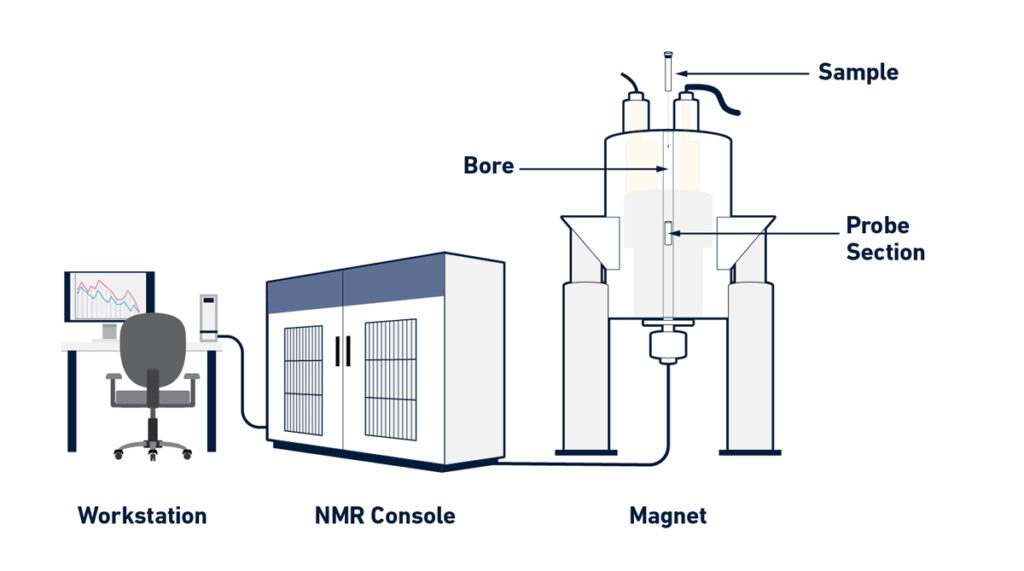
Instrumentation of Nuclear Magnetic Resonance (NMR) Spectroscopy
Nuclear Magnetic Resonance (NMR) spectroscopy apparatus consists of many components that work together to create and detect NMR signals. The following are the main components of an NMR instrument:
- Sample Holder: The sample under analysis is held in a glass tube that is generally 8.5 cm long and 0.3 cm in diameter.
- Magnetic Coils: When an electric current flows through these coils, it creates a magnetic field. The coils’ magnetic field interacts with the atomic nuclei in the sample.
- Permanent Magnet: A permanent magnet creates a uniform magnetic field within the NMR instrument. The magnetic field strength is normally in the 60-100 MHz range.
- Sweep Generator: The sweep generator changes the intensity of the magnetic field supplied to the sample. It enables fine-tuning and modifications to guarantee that the necessary magnetic field strength is achieved.
- Radiofrequency Transmitter: This component is made up of a coil that generates a brief yet strong radio wave pulse. To excite the atomic nuclei, a radiofrequency pulse is delivered to the sample.
- Radiofrequency Receiver: The radiofrequency receiver coil detects the radio frequencies released by the atomic nuclei as they relax to a lower energy level. It records the NMR signals produced by the material.
- RF Detector: The RF detector aids in determining the unabsorbed radio frequencies after the sample has been excited. It gives information about the NMR signals’ resonance frequencies and intensities.
- Recorder: The NMR signals received by the RF detector are recorded by a recorder. It collects and saves data for further study.
- Readout System: The NMR data is analyzed, processed, and interpreted using a computer-based readout system. It includes tools for spectral analysis, data management, and NMR spectrum display.
These components constitute the foundation of NMR spectroscopy apparatus, allowing for the creation, detection, and analysis of NMR signals from samples. The NMR data gives essential information on the structure, composition, and characteristics of the molecules under study.
NMR Spectroscopy Techniques
- Resonant Frequency: The exact frequency at which NMR active nuclei absorb electromagnetic radiation when put in a magnetic field is referred to as the resonant frequency in NMR spectroscopy. The resonance frequency is directly connected to the energy of absorption and is unique to the isotope under investigation. The strength of the magnetic field is related to the intensity of the NMR signal.
- Spectra Acquisition: Acquiring NMR spectra involves igniting the sample with a radiofrequency pulse. The nuclei in the sample undergo nuclear magnetic resonance and generate a response signal as a result of this pulse. The NMR signal, on the other hand, is often quite faint, necessitating the use of sensitive radio receivers to identify and amplify the signal for further investigation.
NMR spectroscopy uses these techniques to examine the behavior, structure, and characteristics of molecules at the atomic and molecular levels. The resonance frequency gives useful information about the nuclei and their chemical environment, whereas spectral collection allows for the detection and analysis of NMR signals for use in chemistry, biology, and materials research.
Chemical Shift in NMR Spectroscopy
- In NMR spectroscopy, a spinning charge generates a magnetic field that results in a magnetic moment proportional to the spin. When placed in an external magnetic field, two spin states exist: one aligned with the magnetic field and one opposed to it.
- Chemical shift refers to the difference in resonant frequency between the spinning protons (or other nuclei) in a molecule and a reference molecule. It is a crucial property used for determining the molecular structure in nuclear magnetic resonance. NMR spectroscopy can detect various nuclei, including 1H (proton), 13C (carbon-13), 15N (nitrogen-15), 19F (fluorine-19), and many more. Among them, 1H and 13C are the most commonly used nuclei.
- The concept of chemical shift is particularly applicable to 1H NMR spectroscopy. Both protons in the nucleus have a positive charge and are in constant motion, resembling a cloud. According to the principles of quantum mechanics, a charge in motion generates a magnetic field. In NMR, when the nucleus is exposed to radio frequency (RF) radiation, it causes the nucleus and its magnetic field to rotate or “pulse,” hence the term NMR.
- By analyzing the chemical shifts in NMR spectra, scientists can gain valuable insights into the chemical environment and molecular structure of a compound. The chemical shift values are typically reported in parts per million (ppm) and provide information about the electron distribution and chemical bonding within the molecule. This information is essential for various applications in organic chemistry, medicinal chemistry, structural biology, and materials science.
Background
In the last fifty years, the nuclear magnetic resonance technique also known as nmr has grown to become the most reliable method for determining the organic structure. Of all the methods for spectroscopy that exist, it is the only one that allows for a full study and analysis of the complete spectrum is usually required. While larger quantities of sample are required than mass spectroscopy however, nmr is not destructive and, with the latest instruments, good results can be obtained from specimens weighing less than 1 milligram. To make the most of nmr for analysis it is essential to know the physical concepts upon which the methods are founded.
The nuclei in many elemental isotopes exhibit a distinct spin (I). Certain nuclei have an integral spin (e.g. I = 1,2 3, ….), others contain fractional spins (e.g. I = 1/2 3/2 5/2, etc.) ….), and some do not have a spin I = 0. (e.g. 12C, 16O, 32S, ….). Isotopes that can be of great significance and importance for organic chemists include 1H 13C, 13C and 19F. 31P. All of them contain I = 1/2. Because the study of the spin state is quite easy, our discussion on the nmr is limited to these as well as other I = 1/2 nuclei.
The following features lead to the nmr phenomenon:
- Spinning Charge and Magnetic Moments: A spinning charge generates an electric field, resulting in magnetic moments (m) that are equal to its spin.
- Two Spin States: In the presence of an external magnetic field (B0), two spin states exist: +1/2 and -1/2. The +1/2 state aligns with the external field, while the -1/2 state opposes it.
- Energy Difference: The energy difference between the two spin states depends on the strength of the external magnetic field. As the field increases, the energy levels diverge.
- Magnetic Field Strength: Strong magnetic fields are required for NMR spectroscopy. The unit for magnetic flux is Tesla (T), and modern NMR spectrometers use magnets with fields ranging from 1 to 20 T.
- Tiny Energy Difference: The energy gap between the two spin states is relatively small, typically around 0.1 Cal/mole. This difference is expressed as a frequency in MHz units and varies based on the magnetic field strength and the specific nucleus being examined.
- Excitation by Radiofrequency (RF) Energy: When a sample is exposed to radiofrequency energy at the frequency corresponding to the spin-state separation of certain nuclei, the nuclei can undergo excitation from the +1/2 spin state to the higher-energy -1/2 spin state.
- Proton (1H) Nucleus: The nucleus of the hydrogen atom (proton) has a well-studied magnetic moment (m = 2.7927). It serves as a common example in NMR spectroscopy.
- Energy Differences and Magnetic Moments: For spin-1/2 nuclei, the energy differences between the spin states are proportional to their magnetic moments. The magnetic moments for nuclei such as 1H, 19F, 31P, and 13C are measured in nuclear magnetons, which are units of magnetic moment.
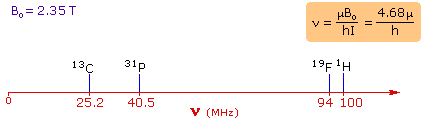
- Relationship Between Frequency and Magnetic Moments: The frequencies corresponding to the energy differences for the mentioned nuclei under a specific magnetic field (2.35 T) can be calculated using a formula that relates frequency (energy variance) to magnetic moments and Planck’s constant (h).
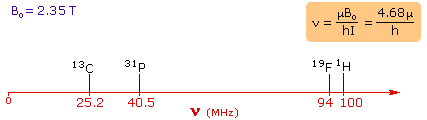
These features collectively contribute to the NMR phenomenon, allowing for the analysis of molecular structures and properties through NMR spectroscopy.
How does NMR work?
Nuclear Magnetic Resonance (NMR) spectroscopy is founded on quantum mechanics concepts and the behavior of atomic nuclei in a magnetic field. This is how NMR works:
- Magnet Generation: NMR spectrometers create a strong and homogenous magnetic field using a superconducting magnet. To produce superconductivity, these magnets are often cooled to very low temperatures (about 4 K) using liquid helium and liquid nitrogen.
- The Probe: The sample to be studied is put within the superconducting magnet’s cylindrical chamber known as the probe. Magnetic coils surround the sample in the probe. These coils perform a variety of functions, including irradiating the sample with radiofrequency (RF) pulses and detecting the NMR signals released by the sample.
- Sample Alignment: When a sample is put in a magnetic field, the nuclear spins of the atoms inside the sample align with the magnetic field. Nuclear spin alignment is critical for NMR analysis.
- Excitation by RF Pulse: To acquire NMR signals, the sample is exposed to a brief and intense RF pulse. This pulse causes the aligned nuclear spins to precess around the magnetic field.
- Detection of NMR Signals: The magnetic coils in the probe also serve as radio receivers. After the RF pulse stimulation, they detect the NMR signals released by the precessing nuclear spins. These signals are extremely faint, necessitating the use of sensitive detecting devices.
- Electronic System and Data Analysis: The NMR spectrometer’s electronic system controls the experimental settings and allows the operator to set up and alter numerous NMR experiment parameters. It also makes data collecting and mathematical processing of obtained data into an NMR spectrum easier.
- NMR Spectrum: A graphical depiction of the NMR signals acquired from the sample is the NMR spectrum. It is made up of a succession of peaks with varying strengths that correspond to distinct atomic nuclei in the sample. The chemical shift, which is related to the Larmor frequency of the nuclei in the magnetic field, determines the position of these peaks.
Scientists may learn about the chemical structure, composition, and dynamics of molecules in a sample by examining the NMR spectrum. NMR spectroscopy is a strong analytical method that is used extensively in chemistry, biology, materials science, and other disciplines.
How to read an NMR spectrum and what it tells you?
The following processes and considerations are necessary to interpret an NMR spectrum and understand the information it provides:
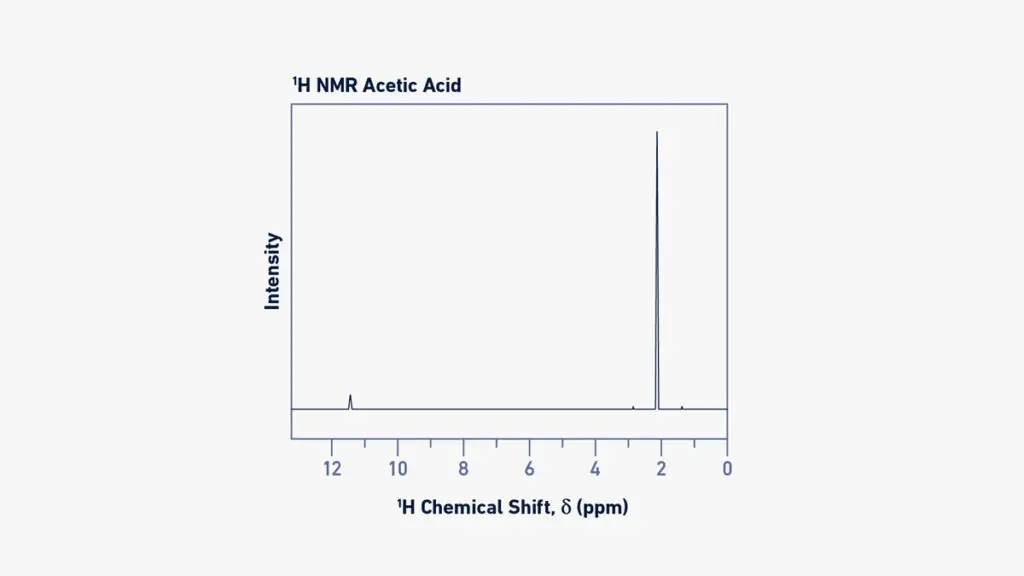
- Chemical Shift: The spectrometer’s measured NMR signal, known as the Free Induction Decay (FID), is processed to yield a relative magnitude known as chemical shift (). This magnitude is independent of the magnetic field and may be compared between equipment. Chemical shift is measured in parts per million (ppm) and is calculated by the observed Larmor frequency (vL) in relation to the Larmor frequency (L0) of a reference nucleus.
- Reference Compound: To set the zero value of the chemical shift scale, a reference substance, such as tetramethylsilane (TMS) or sodium trimethylsilylpropanesulfonate (DSS) for 1H NMR, is utilized.
- Spectral Interpretation: An NMR spectrum may tell you a lot about the chemicals in a sample. The chemical shift values of chemical groups inside a molecule can be used to identify them. In a proton (1H) NMR spectrum, for example, various protons in the molecule will produce unique signals at different chemical shifts.
- Signal Intensity: The area beneath a signal in 1H-NMR spectra is proportional to the number of protons (atomic nuclei) creating that signal. As a result, the signal regions may be utilized to calculate the relative abundance of various protons inside the molecule. This intensity connection, however, does not apply to 13C-NMR spectra.
- Signal Splitting (Multiplicity): When two nuclei are joined by a few chemical bonds, their spins might interact, resulting in signal splitting or multiplicity. The pattern of splitting is governed by the number of connected nuclei and the coupling constant (J), which is dictated by the kind of nuclei and the number of chemical bonds that separate them. The N+1 rule and Pascal’s triangle can be used to anticipate the number of split peaks and their respective intensities.
- Scalar Coupling: Scalar Coupling happens when the number of chemical bonds separating two nuclei is less than four. It gives extra information on the structure and connectivity of the molecule. Scalar coupling can induce signal splitting and peak intensity decrease.
- Nuclear Overhauser Effect (NOE): The nuclear Overhauser effect (NOE) is found in NMR spectra and is caused by the interaction of nuclear spins between atoms that are spatially close yet distant in the molecular sequence. It is required for macromolecule structural determination.
- Spectral Assignment: When interpreting an NMR spectrum, each observable signal is assigned to the relevant atomic nucleus in the molecule(s) present in the sample. This technique, known as spectrum assignment, can be difficult for complicated compounds. To properly describe a material, many types of NMR investigations with diverse and complimentary information are frequently performed.
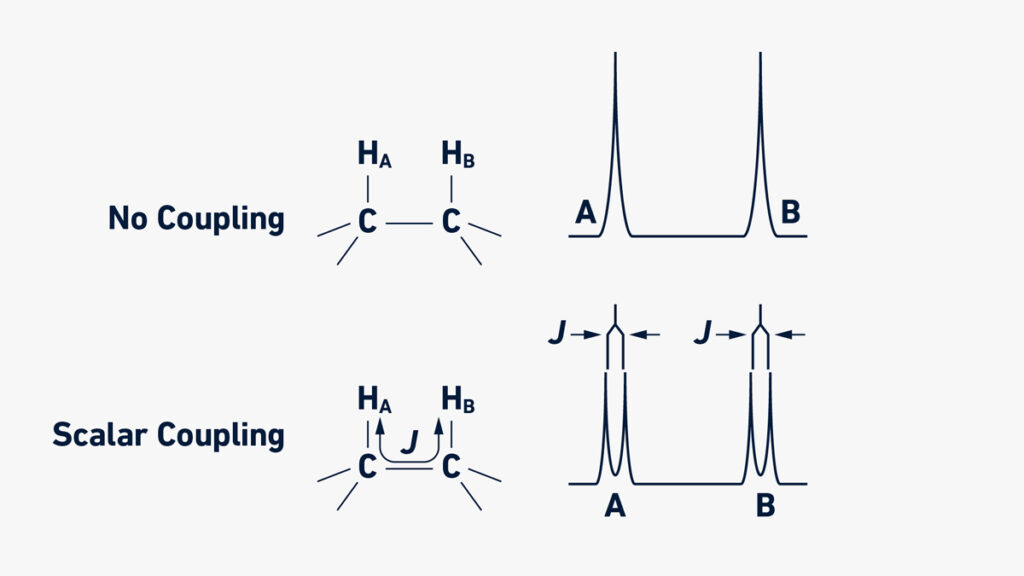
Scientists may learn a lot about the chemical structure, connectivity, and dynamics of molecules in an NMR sample by examining chemical shifts, signal intensities, signal splitting patterns, and other characteristics.
Applications of NMR Spectroscopy
- Quality Control: NMR spectroscopy is widely used as an analytical technique in chemistry for quality control purposes. It helps in determining the composition and purity of a substance, ensuring its adherence to specific standards.
- Research and Structural Analysis: NMR spectroscopy plays a crucial role in research for determining the content, purity, and molecular structure of a sample. It is used to quantitatively analyze mixtures containing known compounds. Chemists rely on one-dimensional NMR techniques for studying chemical structures, while two-dimensional techniques are employed for more complex molecules. NMR methods have also become a substitute for X-ray crystallography in determining protein structures.
- Molecular Dynamics: Time domain NMR spectroscopy techniques are employed to study molecular dynamics in solution. These techniques provide insights into the behavior, conformational changes, phase transitions, solubility, and diffusion of molecules at the molecular level.
- Solid-State Analysis: Solid-state NMR spectroscopy is utilized to investigate the molecular structure of solids. It provides valuable information about the arrangement, interactions, and dynamics of molecules in solid materials.
- Diffusion Measurements: NMR spectroscopy offers methods for measuring diffusion coefficients, which are important in understanding the movement and distribution of molecules in various systems.
NMR spectroscopy finds applications not only in chemistry but also in biochemistry, providing valuable insights into the properties and behavior of organic molecules. It is a versatile technique applicable to any sample containing nuclei with spin, making it a powerful tool for scientific investigations.
Upfield vs downfield NMR
- In NMR spectroscopy, the terms “upfield” and “downfield” are used to describe the positions of signals on the chemical shift scale. The chemical shift values vary for different nuclei based on their local chemical environment and the electronic density surrounding them.
- When a nucleus experiences a high electronic density around it, the shielding effect is high. This means that the circulating electrons create magnetic fields that oppose the applied external field, resulting in a lower effective magnetic field acting on the nucleus. As a result, the Larmor frequency decreases, and the chemical shift moves towards lower values on the scale, which is referred to as “upfield”.
- Conversely, when there is low electronic density in the vicinity of a nucleus, the shielding effect is low, and the effective magnetic field acting on the nucleus is higher. This leads to higher Larmor frequencies and chemical shifts that are located towards higher values on the scale, which is known as “downfield”.
- For example, hydrogen nuclei (protons) in methyl groups or aliphatic molecules are strongly shielded due to high electron density around them. Therefore, their typical chemical shift values are located upfield on the NMR spectrum. On the other hand, hydrogen nuclei attached to electronegative atoms (such as oxygen or nitrogen) or located close to electronegative functional groups (such as carboxylic acids or aldehydes) are deshielded. Consequently, their chemical shift values are located downfield on the NMR spectrum.
- Understanding the upfield and downfield regions of the chemical shift scale helps in interpreting NMR spectra and identifying the chemical environments and functional groups present in a molecule.
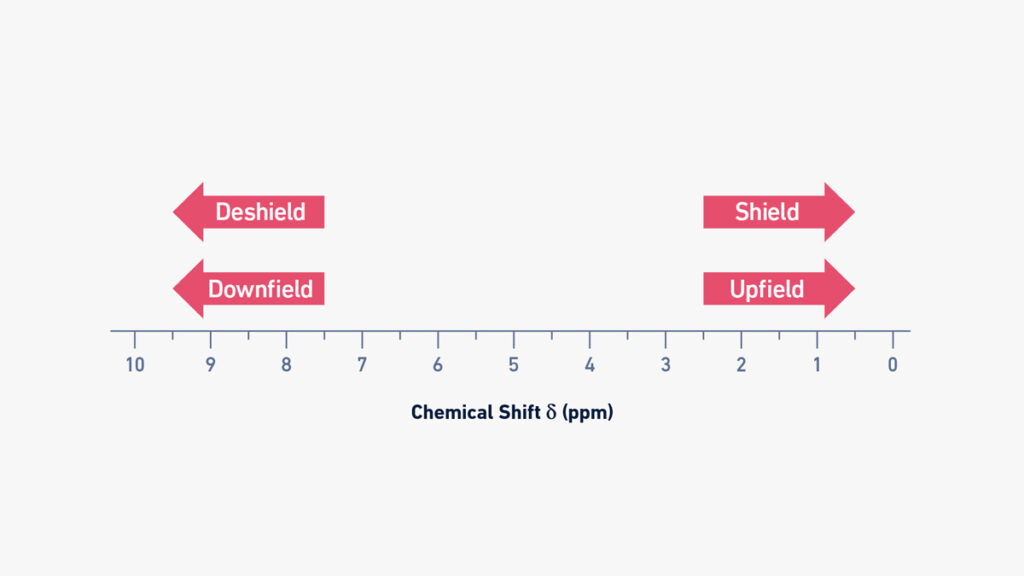
Proton NMR vs carbon NMR
- Proton NMR (1H-NMR) and carbon NMR (13C-NMR) are two extensively used NMR spectroscopy methods with unique properties and applications.
- The identification of hydrogen nuclei, which are abundant in organic and biological molecules, is the basis of 1H-NMR. With a spin of 12, the most prevalent isotope of hydrogen, 1H, is NMR-active. Carbon nuclei (12C), on the other hand, are not NMR-active, and only the isotope 13C, which has a lower natural abundance of 1.11%, can be identified. Furthermore, because 13C has a lower magnetogyric ratio than 1H, 13C-NMR is less sensitive than 1H-NMR. As a result, 13C-NMR experiments often require longer acquisition periods than 1H-NMR experiments.
- Chemical shifts in 1H-NMR generally occur in the range of 0 to 14 ppm, but chemical shifts in 13C-NMR encompass a greater range of 10 to 220 ppm. This disparity in chemical shift range is caused by the nuclei’s differing Larmor frequencies, which are determined by their magnetogyric ratios. When compared to 1H-NMR, the greater range of chemical shifts in 13C-NMR provides for improved signal resolution and dispersion.
- Due to the low natural abundance of 13C, scalar coupling between 13C atoms is seldom detected in 13C-NMR, making close proximity of two 13C atoms uncommon. However, interaction between 13C and other nuclei is conceivable, and this might impair the technique’s sensitivity. In 13C-NMR investigations, specific pulse sequences are utilized to reduce scalar coupling between 13C and 1H.
- Although 13C-NMR has lesser sensitivity and limits than 1H-NMR, it provides vital information that 1H-NMR does not. It, for example, enables the identification of several sorts of carbon atoms (primary, secondary, tertiary, and quaternary) in a molecule. As a result, 13C-NMR and 1H-NMR are frequently utilized in NMR laboratories as a complimentary strategy for determining molecular structure.
- Several strategies may be used to improve the sensitivity of 13C-NMR, such as enriching the sample with 13C, increasing the number of collected spectra to improve the signal-to-noise ratio, and employing NMR pulses to raise the population difference between nuclear spin energy levels.
- To summarize, 1H-NMR is more sensitive and produces more signals, but 13C-NMR gives finer resolution and useful information on the carbon atoms in a molecule. In NMR laboratories, these methods are routinely employed in tandem for complete molecular structure research.
Advanatges and Limitations of NMR and common problems
Advanatges of NMR
- Amenable to many sample types: NMR spectroscopy is applicable to a wide range of sample types, including liquids, solids, tissues, and gases, making it flexible in its use.
- Provides a range of information: NMR can give useful insights into molecule structure, dynamics, interactions, physical characteristics, and quantification. It can provide a thorough grasp of molecules’ chemical and physical characteristics.
- Molecules can be measured in their native state: NMR allows for the measurement of molecules in their natural environment, retaining their original state and minimizing the need for substantial sample processing.
- NMR libraries facilitate chemical identification: NMR spectra of known compounds may be kept in libraries, making it comparatively simple to identify novel compounds by comparing their spectra to the database.
- A high level of automation is possible: NMR equipment may be highly automated, allowing for efficient data collecting and processing. This automation increases productivity while decreasing manual involvement.
- Non-destructive method: NMR spectroscopy is a non-destructive technique that does not compromise the sample’s integrity. This is especially useful when working with valuable or scarce samples.
- High reproducibility: NMR experiments are highly reproducible, providing for consistent and dependable findings.
- Simple and economical sample preparation: Sample preparation for NMR analysis is often simple and inexpensive, involving only minor chemical changes.
Limitations of NMR’s
- Only nuclei with I ≠ 0 can be measured: NMR spectroscopy can only be used on NMR-active nuclei, which have a spin other than zero (I). This constraint limits the number of nuclei that may be investigated with NMR.
- Low sensitivity: NMR is often regarded as a low-sensitivity technology, needing larger sample concentrations or longer collection periods to achieve adequate signal-to-noise ratios, particularly for specific nuclei such as carbon-13.
- Expensive equipment and upkeep: NMR instruments are sophisticated and costly, both in terms of original purchase price and continuous maintenance. These issues may limit access to NMR facilities.
- Some experiments are time-consuming: Some NMR studies can be time-consuming, needing hours or even days to collect enough data for analysis. When speedy findings are required, this might be a practical hindrance.
- Magnetic field inhomogeneities must be rectified: Magnetic field inhomogeneities within an NMR instrument must be addressed using shimming, which is a procedure that optimizes field uniformity. Inadequate shimming might result in poor spectral quality.
- Spectral assignment and data analysis can be difficult: Spectral assignment and data analysis in NMR can be difficult in some situations, especially when working with complicated samples or overlapping signals. Accurate findings require knowledge of NMR interpretation and data analysis.
- spectrum interferences caused by impurities and solvents: Impurities and solvents in the sample might generate spectrum interferences or obfuscate the signals of interest, necessitating careful purification and solvent selection.
- Instrumental optimization is necessary prior to measurements: Before conducting NMR experiments, the instrument must be tuned, matched, and shimming. This optimization method assures optimal instrument performance and spectral purity, but it adds a step before measurements can be taken.
Despite these limits, NMR’s benefits, such as its flexibility, information-rich nature, non-destructiveness, and repeatability, make it a strong tool in a variety of scientific domains, including chemistry, biology, materials science, and medicine.
FAQ
What is NMR spectroscopy?
NMR (Nuclear Magnetic Resonance) spectroscopy is a technique used to study the interaction of atomic nuclei with a strong magnetic field and electromagnetic radiation. It provides information about the chemical structure, dynamics, and interactions of molecules.
How does NMR spectroscopy work?
NMR spectroscopy involves placing a sample in a strong magnetic field and subjecting it to radiofrequency pulses. The atomic nuclei in the sample absorb and re-emit energy at specific frequencies, which can be detected and analyzed to obtain a spectrum.
What information can NMR spectroscopy provide?
NMR spectroscopy can provide information about the molecular structure, chemical environment, connectivity of atoms, molecular dynamics, and interactions between molecules. It can also quantify the amounts of different components in a sample.
What are the common nuclei studied in NMR spectroscopy?
The most commonly studied nuclei in NMR spectroscopy are hydrogen-1 (1H) and carbon-13 (13C). Other nuclei such as nitrogen-15 (15N), oxygen-17 (17O), and phosphorus-31 (31P) can also be studied, depending on the specific application.
What is chemical shift in NMR spectroscopy?
Chemical shift refers to the displacement of NMR signals from a reference compound and is expressed in parts per million (ppm). It provides information about the electronic environment and chemical nature of the atoms in a molecule.
What is coupling in NMR spectroscopy?
Coupling refers to the splitting of NMR signals into multiple peaks due to interactions between neighboring nuclei. It provides information about the connectivity and bonding patterns of atoms in a molecule.
How is NMR spectroscopy used in structural determination?
NMR spectroscopy is widely used to determine the structures of organic molecules, including small organic compounds and complex biomolecules such as proteins and nucleic acids. It can provide information about bond distances, angles, and torsion angles, aiding in the elucidation of molecular structures.
What are the advantages of NMR spectroscopy?
NMR spectroscopy has several advantages, including its non-destructive nature, ability to study samples in various states (solution, solid, tissue), versatility in sample types, high reproducibility, and the range of information it provides about molecules.
What are the limitations of NMR spectroscopy?
Some limitations of NMR spectroscopy include its relatively low sensitivity, especially for certain nuclei, the need for expensive equipment and maintenance, long experimental times for some experiments, spectral interferences from impurities and solvents, and the complexity of spectral analysis for complex samples.
What are common applications of NMR spectroscopy?
NMR spectroscopy finds applications in various fields, including chemistry, biochemistry, pharmaceuticals, materials science, environmental analysis, and forensics. It is used for compound identification, structural determination, monitoring chemical reactions, studying protein-ligand interactions, quality control, and metabolomics, among others.
References
- Rabi II, Millman S, Kusch P, and Zacharias JR. The molecular beam resonance method for measuring nuclear magnetic moments. the magnetic moments of 3Li6, 3Li7 and 9F19. Phys Rev. 1939. 55(6): 526. doi: 10.1103/PhysRev.55.526
- Bloch F, Hansen W, and Packard M. Nuclear induction. Phys Rev. 69: 127L. doi: 10.1103/PhysRev.70.460
- Purcell EM, Torrey HC, and Pound RV. Resonance absorption by nuclear magnetic moments in a solid. 1946. Phys Rev. 69: 37L. doi: 10.1103/PhysRev.69.37
- Emsley JW, and Feeney J. Milestones in the first fifty years of NMR. 1995. Progr Nucl Mag Res Sp. 28(1): 1-9. doi: 10.1016/0079-6565(95)01023-8
- Marion D. An introduction to biological NMR spectroscopy. Mol Cell Proteomics. 2013. 12(11): 3006-3025. doi: 10.1074/mcp.O113.030239
- Friebolin H & Becconsall JK. (2005). Basic one-and two-dimensional NMR spectroscopy (Vol. 7). Weinheim: Wiley-vch. 2005.
- Hore PJ. Nuclear magnetic resonance. USA: Oxford University Press. 2015.
- Derome AE. Modern NMR techniques for chemistry research. Elsevier. 2013.
- Wüthrich K. Protein structure determination in solution by NMR spectroscopy. 1990. J Biol Chem. 1990. 265(36): 22059-22062. doi: 10.1016/S0021-9258(18)45665-7
- Jacobsen NE. NMR data interpretation explained: understanding 1D and 2D NMR spectra of organic compounds and natural products. John Wiley & Sons. 2016.
- Bible RH. Interpretation of NMR spectra: an empirical approach. Springer Science & Business Media. 2013.