What is Neuron?
- Neurons are specialized cells that form the basic working unit of the nervous system. Their primary role is to transmit signals throughout the body, using both electrical and chemical means to communicate. Neurons are responsible for receiving, processing, and passing on information to other cells, whether they be neurons, muscles, or glands.
- A typical neuron is made up of three main components: the soma (or cell body), the axon, and the dendrites. The soma contains the nucleus and is responsible for maintaining the health of the cell. Dendrites extend from the cell body and act as receivers, gathering incoming signals from other neurons or sensory stimuli. These incoming signals are called afferent signals. The axon, on the other hand, transmits outgoing (efferent) signals to other neurons or target cells, such as muscle fibers or organs.
- Neurons are connected to other neurons at synapses, which are specialized junctions that allow for the transfer of information. When a signal reaches the synapse, it can trigger the release of chemicals called neurotransmitters, which pass the message to the next neuron or target cell. In this way, neurons ensure that signals are effectively communicated across long distances within the body, which is essential for controlling actions, reflexes, and cognitive functions like memory and learning.
Definition of Neuron
A neuron is a specialized cell in the nervous system that receives, processes, and transmits information through electrical and chemical signals.
How Do Neurons Work?
Neurons communicate through a combination of electrical impulses and chemical signals, ensuring efficient information transfer throughout the body.
- Neurons are positioned adjacent to each other, separated by a tiny gap known as the synapse.
- The primary function of a neuron is to transmit nerve impulses along its length and across the synapse to the next neuron.
- Neurons generate electrical signals called action potentials. These impulses travel down the axon toward the synaptic terminal.
- Upon reaching the synapse, the action potential triggers synaptic vesicles within the pre-synaptic neuron to release neurotransmitters.
- Neurotransmitters are chemical messengers that diffuse across the synaptic gap, bridging the gap between the pre-synaptic and post-synaptic neurons.
- Once neurotransmitters reach the post-synaptic neuron, they bind to specific receptor sites, which can initiate a new electrical impulse in the receiving cell.
- This process of synaptic transmission ensures the continuation of the nerve signal, allowing for communication between neurons.
- The central nervous system (CNS), which includes the brain and spinal cord, along with the peripheral nervous system (PNS), composed of sensory and motor neurons, utilizes this mechanism for information processing.
- Together, these systems enable a wide range of functions, from reflex actions to complex cognitive tasks.
By facilitating the rapid transmission of signals, neurons play a crucial role in how organisms perceive, react to, and interact with their environment. This intricate process underscores the essential nature of neurons in both sensory and motor functions.
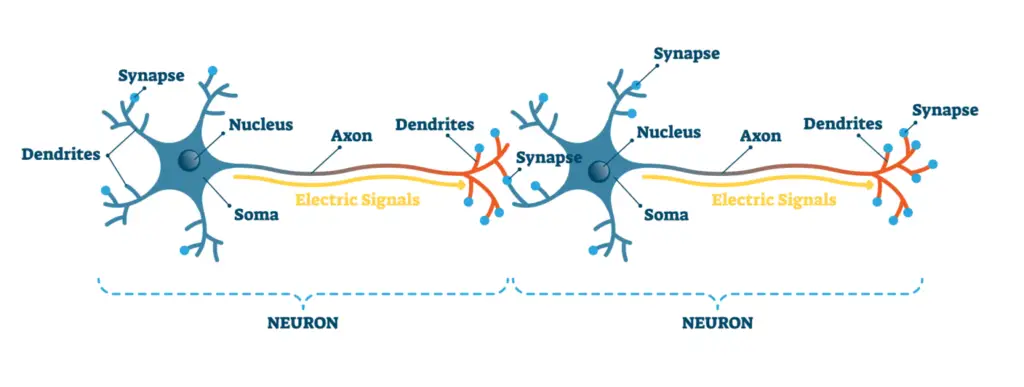
The neuron doctrine
- The neuron doctrine is a foundational concept in neuroscience that articulates the structure and function of neurons as distinct, individual cells. This idea emerged from historical advancements in histology and neuroanatomy, which allowed scientists to observe and understand the complexities of neuronal organization.
- The initial challenge in studying neurons was their minuscule size, typically ranging from 0.01 to 0.05 millimeters in diameter. The invention of the compound microscope in the late seventeenth century marked a pivotal moment, enabling researchers to visualize these tiny cells. However, the soft and jelly-like consistency of brain tissue necessitated further technological innovations, such as the use of formaldehyde for tissue fixation and the development of microtomes for creating ultra-thin slices of tissue.
- The introduction of selective stains, particularly the Nissl stain developed by Franz Nissl in the late nineteenth century, allowed for the differentiation between neurons and glial cells. This staining method highlighted the cell nuclei and surrounding Nissl bodies, revealing insights into the cytoarchitecture of the brain. The study of cytoarchitecture demonstrated that different regions of the brain are specialized for various functions.
- Despite the advances made with the Nissl stain, it was Camillo Golgi’s development of the Golgi stain in 1873 that provided a more comprehensive view of neuronal structure. This stain allowed for the visualization of entire neurons, not just their cell bodies. Golgi’s observations revealed that neurons consist of a central soma and distinct neurites, which include axons and dendrites. The axon serves as the output pathway for the neuron, while dendrites function as input receptors, gathering signals from other neurons.
- Santiago Ramón y Cajal, a contemporary of Golgi, utilized the Golgi stain to explore neuronal circuits and asserted that neurons are not continuous but instead communicate at junctions. This concept opposed Golgi’s reticular theory, which posited that neurites formed a fused network. Cajal’s perspective led to the formulation of the neuron doctrine, asserting that neurons are individual cells that transmit signals via contact rather than continuity.
- The neuron doctrine gained substantial support through subsequent research, particularly with the advent of the electron microscope in the 1950s. This technology provided definitive evidence that neurites from different neurons do not physically connect, reinforcing the notion that each neuron operates as a separate entity. Consequently, the neuron doctrine established the individual neuron as the fundamental unit of nervous system function, laying the groundwork for modern neuroscience.
The neuron doctrine is a fundamental principle in neuroscience, establishing that neurons are the basic structural and functional units of the nervous system. This concept emerged through the efforts of early histologists and their discoveries regarding neuronal structure.
- Historical Context:
- Early Challenges:
- Investigating brain cells presented numerous difficulties due to their microscopic size, typically ranging from 0.01 to 0.05 mm in diameter.
- The invention of the compound microscope in the late 17th century was a crucial advancement that enabled cellular neuroscience to progress.
- Histological Techniques:
- Early neuroscientists faced the challenge of preparing brain tissue for microscopic examination, which required the development of techniques to “fix” tissue and create thin slices using a microtome.
- The introduction of staining methods was pivotal, allowing for the selective coloring of cell components and revealing cellular structures.
- Early Challenges:
- Key Staining Techniques:
- Nissl Stain:
- Developed by Franz Nissl, this stain selectively colors the nuclei of cells and the rough endoplasmic reticulum (Nissl bodies) in neurons.
- The Nissl stain distinguishes between neurons and glial cells, aiding in the study of cytoarchitecture across different brain regions.
- Golgi Stain:
- Camillo Golgi’s silver chromate staining method allowed for the visualization of entire neurons, showing their complex morphology.
- The Golgi stain demonstrated that neurons consist of a cell body (soma) and neurites (axons and dendrites), thus revealing their intricate structure.
- Nissl Stain:
- Contributions of Key Figures:
- Santiago Ramón y Cajal:
- Cajal utilized the Golgi stain to elucidate the organization of neural circuits, arguing against Golgi’s reticular theory.
- He proposed that neurites from different neurons do not fuse but communicate through contact, reinforcing the idea of distinct cellular entities.
- The Rivalry and Collaboration:
- Cajal and Golgi shared the Nobel Prize in 1906, yet they maintained opposing views on neuronal structure, with Cajal’s neuron doctrine gaining prominence over time.
- Santiago Ramón y Cajal:
- Validation of the Neuron Doctrine:
- Scientific Evidence:
- For decades, accumulating evidence supported the neuron doctrine, asserting that neurons are discrete entities.
- The introduction of the electron microscope in the 1950s provided definitive proof by revealing the non-continuous nature of neuronal connections.
- Significance in Neuroscience:
- The neuron doctrine emphasizes that neurons are the fundamental units of communication in the nervous system, responsible for processing and transmitting information.
- This principle laid the groundwork for understanding more complex neural networks and functions in both health and disease.
- Scientific Evidence:
In summary, the neuron doctrine, solidified by the contributions of early scientists, underscores the importance of neurons as distinct entities within the nervous system. This doctrine continues to shape our understanding of neurobiology and the intricate workings of the brain.
Structure of Neuron
The structure of a neuron is essential for its function in the nervous system. Neurons are composed of several key components that work together to facilitate the transmission of electrical impulses and chemical signals. Understanding these parts provides insight into how neurons communicate and process information.
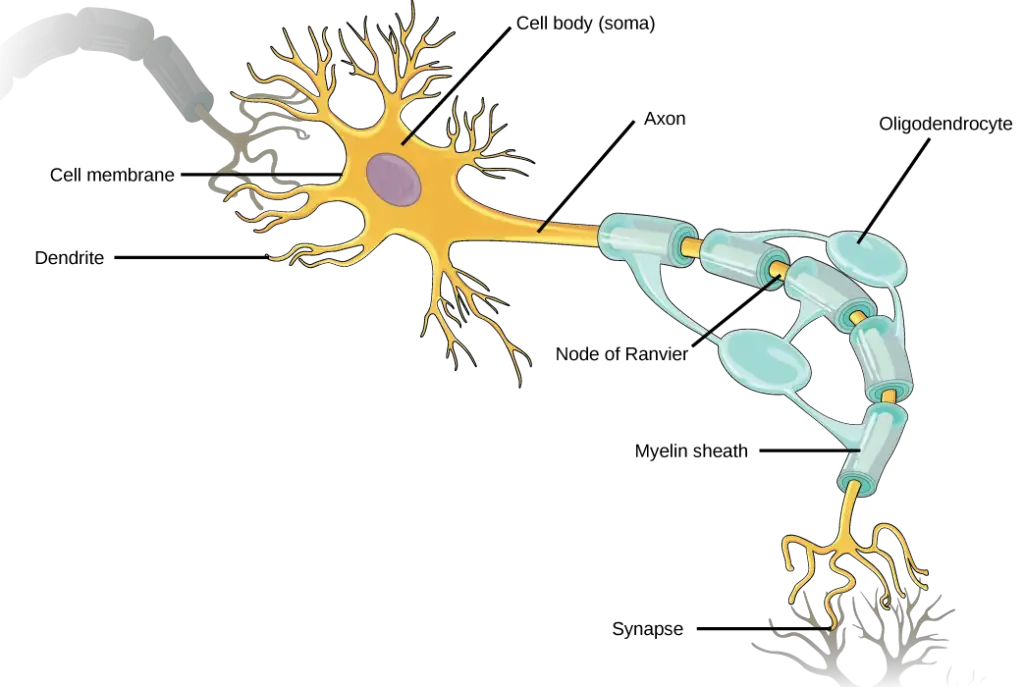
- Soma (Cell Body): The soma is the core of the neuron, housing the nucleus, which contains genetic material. It is responsible for maintaining the neuron’s health and supporting its metabolic functions. The soma also contains rough endoplasmic reticulum, known as Nissl substance, which is involved in protein synthesis vital for neuronal function.
- Dendrites: Dendrites are branched, tree-like structures that extend from the soma. Their primary function is to receive signals from other neurons and the environment. They are usually shorter and more numerous than axons, allowing neurons to form connections with multiple other neurons. In some types of neurons, like Purkinje cells in the cerebellum, dendrites are highly developed to facilitate extensive signal reception.
- Axon: The axon is a long, thin extension that conducts electrical impulses away from the cell body. It joins the soma at a region called the axon hillock. Most neurons possess a single axon, which can vary significantly in length. The axon serves as a conduit, transmitting action potentials to other neurons or target cells such as muscles and glands.
- Myelin Sheath: Many axons are covered with a fatty insulating layer called the myelin sheath, which is formed by glial cells—oligodendrocytes in the central nervous system and Schwann cells in the peripheral nervous system. This sheath enhances the speed of signal transmission and protects the axon. The myelin sheath has gaps known as nodes of Ranvier, which facilitate rapid electrical conduction through a process called saltatory conduction.
- Axon Terminals: Located at the distal end of the axon, axon terminals (or terminal buttons) are responsible for transmitting signals to other neurons. These terminals contain synaptic vesicles filled with neurotransmitters. Upon reaching the axon terminals, the electrical impulse triggers the release of neurotransmitters into the synaptic gap, allowing communication with adjacent neurons.
- Synapse: The synapse is the junction where communication occurs between two neurons. Although neurons do not physically touch, neurotransmitters released from the axon terminals of one neuron cross the synaptic gap and bind to receptors on the dendrites of another neuron, thereby transmitting signals.
- Coverings in the Peripheral Nervous System: In the PNS, axons are bundled to form nerves, surrounded by connective tissue layers. The endoneurium encases individual axons, the perineurium surrounds groups of axons (fascicles), and the epineurium encloses the entire nerve, which includes blood vessels that supply the nerve fibers.
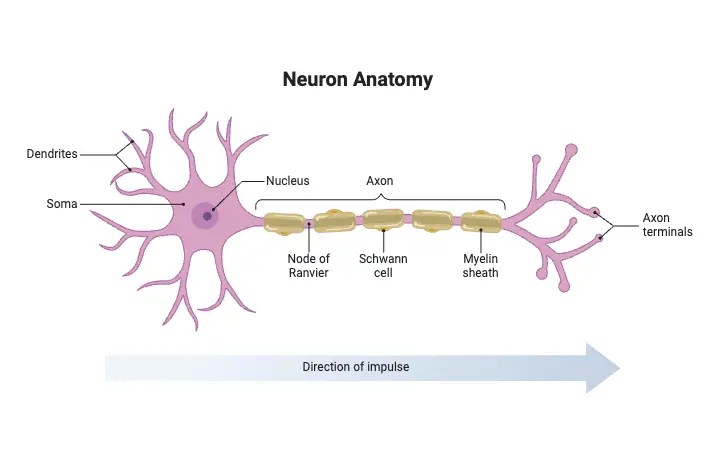
Part of Neuron | Description |
---|---|
Cell Body (Soma) | The core of the neuron containing the nucleus, Golgi body, endoplasmic reticulum, mitochondria, and other cellular components. It maintains the cell’s functions, synthesizes proteins, and provides energy. |
Dendrites | Branch-like structures that receive messages from other neurons and transmit them to the cell body. They are covered in synapses and increase the surface area for connections with other neurons. |
Axon | A tube-like structure responsible for carrying electrical impulses away from the cell body to axon terminals. It can be covered by a myelin sheath, which insulates and speeds up the transmission of nerve impulses. |
Axon Terminals | Found at the end of the axon, these specialized structures contain synapses and release neurotransmitter chemicals to communicate with other neurons. |
Synapse | The junction between the axon terminal of one neuron and the dendrites of another neuron, where neurotransmitters are released and signals are transmitted from one neuron to another. |
Myelin Sheath | A layer of fatty material that covers the axons of neurons, providing insulation between nerve cells and speeding up the conduction of nerve impulses. |
Axon Hillock | A specialized structure located at the junction between the cell body and the axon. It integrates signals from multiple synapses and acts as a threshold detector, determining whether an action potential should be generated. |
Parts of Neuron with their functions
The Soma
The soma, also known as the cell body, forms the core of a neuron, containing essential structures for cellular function. The size of a typical neuron’s soma is about 20 µm in diameter. The fluid inside the soma, called cytosol, is rich in potassium and separated from the external environment by the neuronal membrane. Within this soma are multiple membrane-bound organelles, each playing a critical role in maintaining the cell’s functionality.
- Cytoplasm and Organelles:
- The cytoplasm encompasses everything within the cell membrane, excluding the nucleus.
- Organelles within the soma include the nucleus, rough endoplasmic reticulum (ER), smooth ER, the Golgi apparatus, and mitochondria.
- Nucleus:
- The nucleus, around 5–10 µm in size, is encased in a double membrane known as the nuclear envelope, perforated by small pores.
- Inside the nucleus are chromosomes containing DNA, the genetic material passed from parents, coding the unique characteristics of each cell.
- DNA houses genes, segments of genetic code responsible for assembling specific proteins.
- Protein synthesis is initiated by gene expression, where DNA is transcribed into mRNA and then translated into proteins in the cytoplasm.
- Gene Expression:
- DNA transcription into mRNA occurs within the nucleus, with mRNA exiting through nuclear pores to guide protein synthesis.
- This process is tightly regulated by promoters, terminators, and transcription factors that control where RNA polymerase binds and stops transcription.
- RNA splicing removes non-coding regions (introns) from the mRNA transcript, leaving only coding sequences (exons).
- Rough Endoplasmic Reticulum (Rough ER):
- Rough ER is abundant in neurons and is the primary site of protein synthesis, especially for membrane-bound proteins.
- Free ribosomes synthesize cytosolic proteins, while rough ER synthesizes proteins destined for membranes or organelles.
- Smooth Endoplasmic Reticulum (Smooth ER):
- Smooth ER helps in folding membrane proteins and regulating intracellular concentrations of substances, such as calcium.
- In some cells, it is involved in critical processes like muscle contraction by controlling calcium levels.
- Golgi Apparatus:
- The Golgi apparatus modifies and sorts proteins for delivery to specific regions of the neuron, such as dendrites or axons.
- Mitochondria:
- Mitochondria, 1 µm in length, are responsible for cellular respiration and energy production.
- Through the Krebs cycle and electron transport chain, mitochondria generate ATP, the cell’s energy currency.
- ATP powers many neuronal processes, including maintaining concentration gradients across the cell membrane.
The Neuronal Membrane
The neuronal membrane plays a fundamental role in maintaining the neuron’s internal environment and controlling interactions with the external environment. This membrane, approximately 5 nanometers thick, acts as a selective barrier that regulates the movement of substances in and out of the neuron, ensuring the proper function of the cell.
- Structure and Composition:
- The neuronal membrane encloses the cytoplasm, separating the internal contents of the neuron from the surrounding extracellular fluid.
- It consists of a lipid bilayer embedded with various proteins that are crucial for its function.
- Membrane Proteins:
- The membrane is embedded with specialized proteins that serve different functions.
- Some of these proteins act as pumps, actively moving substances such as ions from the inside to the outside of the neuron.
- Others form channels or pores that allow specific ions or molecules to passively diffuse into or out of the neuron.
- Regional Variation in Protein Composition:
- The protein composition of the neuronal membrane varies depending on its location—whether in the soma, dendrites, or axon.
- This variation in membrane proteins allows different regions of the neuron to perform distinct functions, such as receiving signals in the dendrites and transmitting them through the axon.
- Role in Signal Transmission:
- The neuronal membrane is essential for the generation and propagation of electrical signals, known as action potentials, which allow neurons to communicate.
- Voltage-gated ion channels in the membrane open and close in response to changes in membrane potential, enabling the flow of ions that drives the electrical signals.
- Selective Permeability:
- The membrane is selectively permeable, meaning it allows certain substances to pass while restricting others.
- This selective permeability is critical for maintaining the proper ionic gradients that are necessary for the electrical signaling capabilities of neurons.
- Membrane Thickness and Function:
- At approximately 5 nanometers thick, the membrane is structurally delicate but functionally robust, providing a protective layer while allowing complex regulatory mechanisms to occur.
- Its thinness facilitates rapid changes in membrane potential, which is vital for fast signal transmission in the nervous system.
The Cytoskeleton
The cytoskeleton is a dynamic network of structural proteins that provides the neuron with its shape and mechanical support. This internal framework is composed of three primary types of fibers: microtubules, microfilaments, and neurofilaments, all of which contribute to the neuron’s structure and function.
- Microtubules:
- Microtubules are the largest cytoskeletal elements, measuring approximately 20 nm in diameter.
- They run longitudinally down the neurites and are essential for maintaining the structure of the neuron.
- Each microtubule is a hollow tube formed by polymerized strands of tubulin protein, arranged in a braided pattern around a central core.
- The process of polymerization (assembly) and depolymerization (disassembly) of tubulin proteins regulates the shape and flexibility of the neuron.
- Microtubule-associated proteins (MAPs) aid in the assembly and stabilization of microtubules, anchoring them to other parts of the neuron.
- Pathological changes in the MAP protein tau are linked to Alzheimer’s disease, where abnormal tau accumulation disrupts axonal function.
- Microfilaments:
- Microfilaments are the thinnest cytoskeletal elements, measuring about 5 nm in diameter.
- They are composed of two intertwined strands of the actin protein, one of the most abundant proteins in all cell types.
- Actin filaments are involved in maintaining and altering cell shape, allowing neurons to adapt and change structurally.
- Like microtubules, microfilaments constantly undergo assembly and disassembly, which is regulated by cellular signals.
- Microfilaments are particularly dense in neurites and closely associate with the neuronal membrane.
- These filaments anchor to the membrane via a network of fibrous proteins that line the inside of the membrane, stabilizing the structure.
- Neurofilaments:
- Neurofilaments have a diameter of about 10 nm, making them intermediate in size between microtubules and microfilaments.
- These are specialized intermediate filaments unique to neurons, though similar structures exist in other cells under different names (e.g., keratin in hair).
- Neurofilaments are highly durable, providing mechanical strength to neurons, much like bones and ligaments do in the human body.
- They are composed of multiple protein subunits twisted together into a ropelike structure, making them particularly resilient and strong.
The Axon
The axon is a specialized structure found exclusively in neurons, designed for the efficient transmission of electrical signals over varying distances in the nervous system. Its unique features and functions distinguish it from other cellular components, making it essential for neuronal communication.
- Axon Structure:
- The axon originates from the axon hillock, a tapered region that extends from the soma, leading to the axon proper.
- Key structural differences include:
- The absence of rough endoplasmic reticulum (ER) and a lack of free ribosomes in the axon, which prevents protein synthesis within the axon itself.
- A distinct protein composition in the axonal membrane compared to the soma, enabling specialized functions related to signal transmission.
- Functionality:
- Because protein synthesis does not occur in the axon, all necessary proteins are synthesized in the soma and transported down the axon.
- Axons can vary in length, extending from less than a millimeter to over a meter, with diameters ranging from under 1 µm to approximately 25 µm in humans.
- In some species, like squids, axons can reach diameters of up to 1 mm.
- The diameter of the axon influences the speed of the electrical impulses that travel through it; larger diameters facilitate faster impulse conduction.
- Axon Terminals:
- The axon concludes at the axon terminal, or terminal bouton, where it forms synapses with other neurons or cells.
- Each axon terminal is often bulbous in appearance and contains synaptic vesicles filled with neurotransmitters.
- The terminal cytoplasm differs from the axon, as it lacks microtubules and has a high concentration of mitochondria to meet its energy demands.
- The synapse represents the junction between the presynaptic axon terminal and the postsynaptic membrane of another neuron, facilitating the transmission of information through chemical signals.
- The axon concludes at the axon terminal, or terminal bouton, where it forms synapses with other neurons or cells.
- Synaptic Transmission:
- Electrical impulses traveling down the axon are converted into chemical signals at the axon terminal.
- Neurotransmitters are released from synaptic vesicles into the synaptic cleft and bind to receptors on the postsynaptic membrane, transforming the signal back into an electrical impulse.
- This process is critical for neuronal communication, underlining the foundation for learning, memory, and various neural functions.
- Axoplasmic Transport:
- Axonal transport is vital for delivering proteins and other materials from the soma to the axon terminal, known as anterograde transport.
- This process utilizes kinesin proteins that “walk” along microtubules, moving materials at rates up to 1,000 mm per day.
- Retrograde transport moves materials back to the soma, providing feedback on the metabolic state of the axon terminal and using dynein proteins for transport.
- Both transport mechanisms are essential for maintaining neuronal health and function and are utilized by researchers to trace neural connections.
- Axonal transport is vital for delivering proteins and other materials from the soma to the axon terminal, known as anterograde transport.
Dendrites
Dendrites, derived from the Greek word for “tree,” are essential structures in neurons that resemble the branching patterns of a tree. Collectively known as the dendritic tree, these extensions play a critical role in receiving and integrating signals from other neurons.
- Structure and Function:
- Dendrites are characterized by their extensive branching, allowing them to form a complex network with multiple synapses.
- Each branch of the dendritic tree, called a dendritic branch, contributes to the neuron’s ability to receive inputs from various sources.
- The dendritic membrane at the synapses is embedded with specialized protein molecules known as receptors. These receptors are essential for detecting neurotransmitters released into the synaptic cleft.
- Dendritic Spines:
- Some dendrites possess specialized structures called dendritic spines, which are small protrusions that enhance the surface area for synaptic connections.
- These spines have a unique morphology and are believed to compartmentalize biochemical reactions resulting from synaptic activation.
- The structure and density of dendritic spines can change in response to synaptic activity, and alterations in spine morphology are associated with cognitive impairments.
- Cytoplasmic Composition:
- The cytoplasm of dendrites shares similarities with that of axons, containing cytoskeletal elements and mitochondria, which support their structural integrity and energy needs.
- A distinguishing feature of dendrites is the presence of polyribosomes, especially beneath dendritic spines. These polyribosomes facilitate local protein synthesis in response to synaptic transmission.
- Local Protein Synthesis:
- Research indicates that synaptic activity can influence protein synthesis locally within dendrites. This capacity is critical for synaptic plasticity and memory formation.
- The regulation of local protein synthesis is essential for the brain’s ability to store and process information, highlighting the functional importance of dendritic structures in neuronal communication.
Types of Neuron
Neurons can be classified based on their structure and function, with each type playing a distinct role in the nervous system.
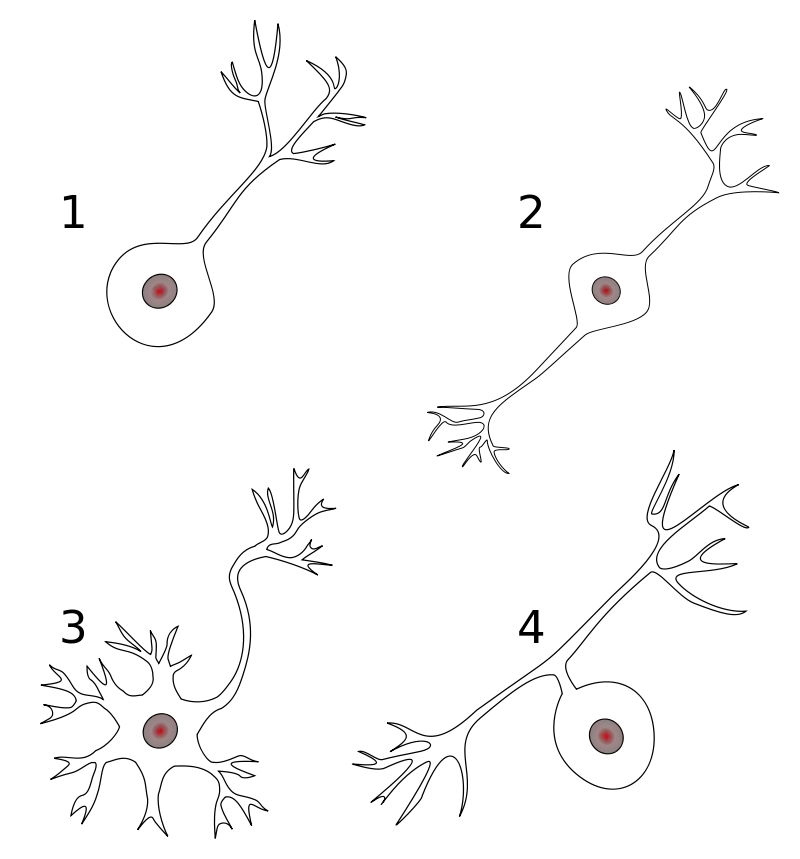
- Structural Classification:
- Unipolar Neurons: These neurons have a single, unbranched axon extending from one end of the cell body. Notably, they lack dendrites. Unipolar neurons are typically found in the cochlear nucleus of the brain and are involved in sensory functions.
- Pseudounipolar Neurons: Characterized by a single axon that bifurcates into two branches, these neurons possess a cell body located along the axon. They are primarily sensory neurons, gathering information from the environment and relaying it to the central nervous system (CNS).
- Bipolar Neurons: Bipolar neurons feature two processes extending from a central cell body—one axon and one dendrite. These neurons are primarily located in specialized sensory areas, such as the retina, where they facilitate vision.
- Multipolar Neurons: The most common type, multipolar neurons, have one axon and multiple dendrites. The cell body is typically positioned to one side of the axon. This structure enables them to integrate a significant amount of information, and they are commonly found in motor functions.
- Functional Classification:
- Sensory Neurons (Afferent Neurons): These neurons detect and transmit signals from the external environment to the CNS. They gather information via sensory receptors and convert stimuli (such as touch, taste, and pain) into electrical impulses that travel toward the CNS. Sensory neurons usually exhibit a pseudounipolar structure, characterized by small axons.
- Motor Neurons (Efferent Neurons): Motor neurons carry signals away from the CNS to muscles or glands, initiating voluntary and involuntary movements. They can be further divided into upper and lower motor neurons. Upper motor neurons send signals to interneurons and lower motor neurons, while lower motor neurons receive inputs and translate them into muscle actions. These neurons typically have a multipolar structure, allowing for extensive connectivity.
- Interneurons: Predominantly located within the CNS, interneurons serve as connectors between sensory and motor neurons. They are involved in processing and integrating information, receiving signals from sensory neurons or other interneurons and transmitting outputs to motor neurons. Interneurons possess a complex structure with multiple dendrites, facilitating intricate networks of communication.
Classification of neuron based on Neuronal Structure and Gene Expression
Classifying neurons is a crucial endeavor in neuroscience that aims to simplify the complexity of the nervous system’s 85 billion neurons by organizing them into categories based on shared characteristics. This classification can help in understanding how different types of neurons contribute to overall brain function.
- Classification Based on Neuronal Structure:
- Number of Neurites:
- Neurons can be classified according to the number of neurites (axons and dendrites) that extend from the soma.
- Unipolar neurons have one neurite, bipolar neurons possess two, and multipolar neurons contain three or more. Most neurons in the brain fall into the multipolar category.
- Dendritic Morphology:
- The shapes and structures of dendritic trees vary significantly across neuron types, leading to distinct naming conventions, such as “double bouquet cells” and “chandelier cells.”
- In specific brain regions, like the cerebral cortex, neurons can be classified into stellate cells (star-shaped) and pyramidal cells (pyramid-shaped).
- Spiny vs. Aspinous Dendrites:
- Neurons with spiny dendrites are termed spiny, while those without are called aspinous.
- For instance, all pyramidal cells are spiny, whereas stellate cells can be either spiny or aspinous.
- Connections:
- Neurons can be categorized by their connections: primary sensory neurons carry information from sensory surfaces (e.g., skin, retina), motor neurons connect to muscles to facilitate movement, and interneurons connect only with other neurons.
- Axon Length:
- Neurons can also be classified based on axon length: Golgi type I neurons (projection neurons) have long axons that connect different brain regions, while Golgi type II neurons (local circuit neurons) have short axons confined to their local area.
- For example, pyramidal cells in the cerebral cortex are typically Golgi type I, while stellate cells are Golgi type II.
- Number of Neurites:
- Classification Based on Gene Expression:
- Genetic Differences:
- Variations in gene expression contribute to the morphological differences between neuron types. For instance, genetic mechanisms dictate the shapes of pyramidal and stellate cells.
- Techniques such as transgenic mice, utilizing fluorescent proteins like GFP, allow researchers to visualize specific neuron types and study their functions.
- Neurotransmitter Systems:
- Neurons can also be classified by the neurotransmitter they utilize, which results from differences in the expression of proteins involved in neurotransmitter synthesis, storage, and release.
- For example, motor neurons that release acetylcholine are classified as cholinergic, indicating the presence of genes for this neurotransmitter.
- Genetic Differences:
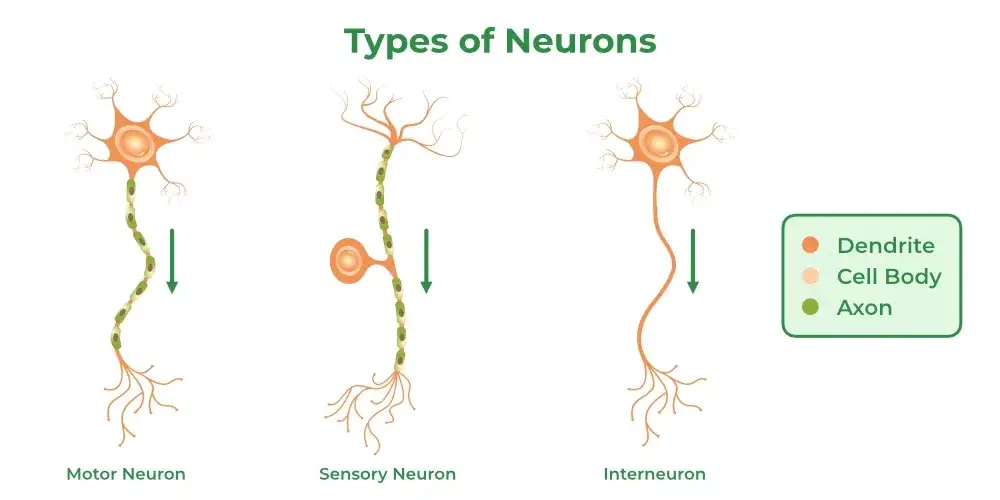
What is Glia?
Glia, often considered the “sleeping giants” of neuroscience, play crucial roles in supporting neuronal function and maintaining brain health. While traditionally viewed as subordinate to neurons, recent research highlights their significant contributions to information processing in the brain.
- Astrocytes:
- Prevalence and Structure:
- Astrocytes are the most abundant type of glial cell in the brain, occupying the spaces between neurons.
- The narrow gap between neurons and astrocytes is about 20 nanometers, indicating their close association with neuronal processes.
- Regulation of Extracellular Environment:
- A primary function of astrocytes is to regulate the chemical composition of the extracellular space.
- They envelop synaptic junctions, limiting the diffusion of neurotransmitter molecules and enhancing synaptic efficiency.
- Neurotransmitter Management:
- Astrocytes possess membrane proteins that actively remove excess neurotransmitters from the synaptic cleft.
- They contain receptors for neurotransmitters, allowing them to respond to neuronal activity and trigger internal electrical and biochemical responses.
- Ionic Homeostasis:
- Astrocytes are vital in maintaining the extracellular concentration of ions, such as potassium, which are essential for proper neuronal function.
- Prevalence and Structure:
- Myelinating Glia:
- Oligodendroglia and Schwann Cells:
- Oligodendrocytes and Schwann cells are specialized glial cells responsible for forming myelin sheaths around axons, which insulate and protect them.
- Oligodendrocytes are located in the central nervous system (CNS), while Schwann cells are found in the peripheral nervous system (PNS).
- Myelin Structure and Function:
- Myelin wraps around axons in a spiral manner, creating a sheath that is critical for the rapid transmission of nerve impulses.
- The myelin sheath is interrupted at intervals by nodes of Ranvier, which facilitate the saltatory conduction of action potentials along the axon.
- Differences in Myelination:
- Each oligodendrocyte can myelinate multiple axons, whereas a single Schwann cell myelinates only one axon.
- Oligodendroglia and Schwann Cells:
- Other Non-Neuronal Cells:
- Ependymal Cells:
- Ependymal cells line the ventricles of the brain and are involved in the production and circulation of cerebrospinal fluid, as well as guiding cell migration during brain development.
- Microglia:
- Microglia act as the brain’s immune cells, functioning as phagocytes to eliminate debris from dead or degenerating neurons and glia.
- They play a role in remodeling synaptic connections, with their activity influencing overall brain plasticity and health.
- Vasculature:
- The brain contains a network of blood vessels, including arteries, veins, and capillaries, which supply essential nutrients and oxygen to neurons, underscoring the interdependence between glia and the vascular system.
- Ependymal Cells:
What is Neurotransmitter?
Neurotransmitters are chemical messengers that facilitate communication between neurons or between neurons and target cells such as muscle cells or gland cells.
Here are some examples of neurotransmitters and their functions:
- Acetylcholine (ACh): Released by cholinergic neurons, acetylcholine acts as a ligand for both ligand-gated ion channels and metabotropic muscarinic receptors. It is involved in various processes such as muscle contraction, learning, memory, and attention. Acetylcholine is synthesized from choline and acetyl coenzyme A.
- Noradrenaline (norepinephrine): Released by adrenergic neurons, noradrenaline binds to alpha adrenoceptors and beta adrenoceptors. It plays a role in the sympathetic nervous system, regulating functions such as the stress response, heart rate, and blood pressure. Noradrenaline is synthesized from the amino acid tyrosine.
- Gamma-aminobutyric acid (GABA): GABA is an inhibitory neurotransmitter in the central nervous system (CNS). It opens anion channels that allow chloride ions to enter the post-synaptic neuron, leading to hyperpolarization and decreased probability of an action potential firing. GABA helps regulate neuronal excitability and is synthesized from glutamate neurotransmitters.
- Glutamate: Glutamate is the primary excitatory neurotransmitter in the central nervous system. It binds to various receptors, including AMPA, kainate, NMDA, and metabotropic receptors. Glutamate is involved in synaptic transmission, learning, and memory. Excessive release of glutamate can lead to excitotoxicity and neuronal damage.
- Dopamine: Dopamine acts on D1 and D2 type receptors, modulating both pre- and post-synaptic neurotransmission. It plays a crucial role in reward-motivated behavior, mood regulation, and movement coordination. Dysfunction of dopamine neurons is associated with disorders like Parkinson’s disease and schizophrenia.
- Serotonin (5-HT): Serotonin can act as both an excitatory and inhibitory neurotransmitter. It binds to various receptor classes, including GPCRs and ligand-gated ion channels. Serotonin is involved in mood regulation, sleep, appetite, and pain modulation. Altered serotonin levels have been linked to depression and anxiety disorders.
- Adenosine triphosphate (ATP): ATP acts as a neurotransmitter at both ligand-gated ion channels (P2X receptors) and GPCRs (P2Y receptors). It is known as a cotransmitter and plays a role in purinergic signaling, which regulates processes such as synaptic transmission, inflammation, and pain perception.
- Histamine: Histamine functions as a monoamine neurotransmitter and neuromodulator. It is involved in regulating arousal, sleep/wake behaviors, and allergic responses. Histamine-producing neurons are located in the tuberomammillary nucleus of the hypothalamus.
These neurotransmitters play crucial roles in transmitting signals and modulating various physiological and cognitive functions in the nervous system. Imbalances or dysfunctions in neurotransmitter systems can contribute to neurological and psychiatric disorders.
Communication Between Neurons
Communication between neurons is essential for the functioning of the nervous system. Neurons utilize electrical and chemical signals to transmit information to one another. Similar to how humans communicate using language and gestures, neurons rely on these specialized signals to exchange information and carry out various functions.
Neurons receive input from multiple other neurons, and this information is integrated and processed before a decision is made to transmit the message further. Communication between neurons occurs through two main mechanisms: electrical signals and chemical signals.
Electrical signals within a neuron are known as action potentials. An action potential is a brief depolarization, or reduction in the charge across the neuron’s membrane, that travels along the neuron’s axon. This electrical impulse allows for the efficient transmission of signals within a single neuron, from the dendrites (receiving end) to the axon terminal (transmitting end). Action potentials are all-or-nothing events, meaning they either occur fully or not at all, without degrees of magnitude.
Chemical signals, on the other hand, are involved in the communication between adjacent neurons. When an action potential reaches the axon terminal of a neuron, it triggers the release of chemical messengers called neurotransmitters. Neurotransmitters are specialized molecules that can depolarize or hyperpolarize the adjacent neuron, influencing the likelihood of an action potential occurring in the receiving neuron.
The communication between neurons relies on three fundamental phenomena:
- Resting Potential: The resting potential refers to the electrical charge of a neuron when it is not actively transmitting a signal. It is characterized by a voltage difference across the neuron’s membrane, with a negative charge on the inside and a positive charge on the outside. This resting potential provides the baseline state from which changes in electrical charge can occur.
- Action Potential: An action potential is a rapid and transient depolarization of the neuron’s membrane. It occurs when the membrane potential reaches a threshold level, triggering a cascade of events that result in the propagation of the action potential along the axon. The action potential allows for the efficient transmission of signals over long distances within a neuron.
- Neurotransmitters: Neurotransmitters are chemical messengers released from one neuron that bind to receptors on the adjacent neuron. They can either depolarize the receiving neuron, making it more likely to generate an action potential (excitatory neurotransmitters), or hyperpolarize the receiving neuron, making it less likely to generate an action potential (inhibitory neurotransmitters). The specific neurotransmitters released and their interactions determine the overall excitatory or inhibitory effect on the receiving neuron.
Through the coordinated interplay of electrical and chemical signaling, neurons establish complex communication networks within the nervous system. This communication enables the transmission and integration of information, supporting various functions such as sensory perception, motor control, memory formation, and decision-making processes.
1. The Resting Potential
- The resting potential of a neuron refers to its membrane potential when it is not actively transmitting a signal. The lipid bilayer membrane surrounding the neuron is impermeable to charged molecules or ions. In order for ions to enter or exit the neuron, they must pass through specialized proteins called ion channels that span the membrane and regulate the ion concentrations inside and outside the cell.
- The resting potential is established by two main processes: the sodium-potassium pump and the potassium leak channels. The sodium-potassium pump, also known as Na+/K+ ATPase, actively transports sodium ions out of the cell and potassium ions into the cell. This pump utilizes ATP energy to maintain an electrical gradient across the cell membrane. It moves three sodium ions out of the cell and two potassium ions into the cell for each ATP molecule consumed. This pump is particularly abundant in nerve cells and plays a crucial role in maintaining the electrical gradient.
- In addition to the sodium-potassium pump, neurons also possess potassium leak channels and sodium leak channels. These channels allow the diffusion of potassium and sodium ions down their concentration gradients. However, neurons have a greater number of potassium leak channels compared to sodium leak channels. As a result, potassium ions diffuse out of the cell at a faster rate than sodium ions leak in. This causes more positive charges to leave the cell than enter, leading to a net negative charge inside the cell relative to the outside.
- The combined effects of the sodium-potassium pump and potassium leak channels contribute to the resting membrane potential. The inside of the cell is negatively charged, with a resting potential of approximately -70 millivolts (-70 mV). This negative charge is due to the differences in ion concentrations inside and outside the cell.
- It is worth noting that chloride ions (Cl-) tend to accumulate outside the cell as they are repelled by negatively charged proteins within the cytoplasm. The concentrations of ions inside and outside neurons can vary, but typical values include a higher concentration of sodium ions outside the cell (around 145 mM) compared to inside (12 mM), a higher concentration of potassium ions inside the cell (155 mM) compared to outside (4 mM), and a higher concentration of chloride ions outside the cell (120 mM) compared to inside (4 mM).
- The resting potential is crucial for the functioning of neurons as it serves as a baseline electrical state from which changes in electrical charge can occur. It provides the necessary conditions for the generation and propagation of action potentials, which are essential for neuronal communication and information processing within the nervous system.
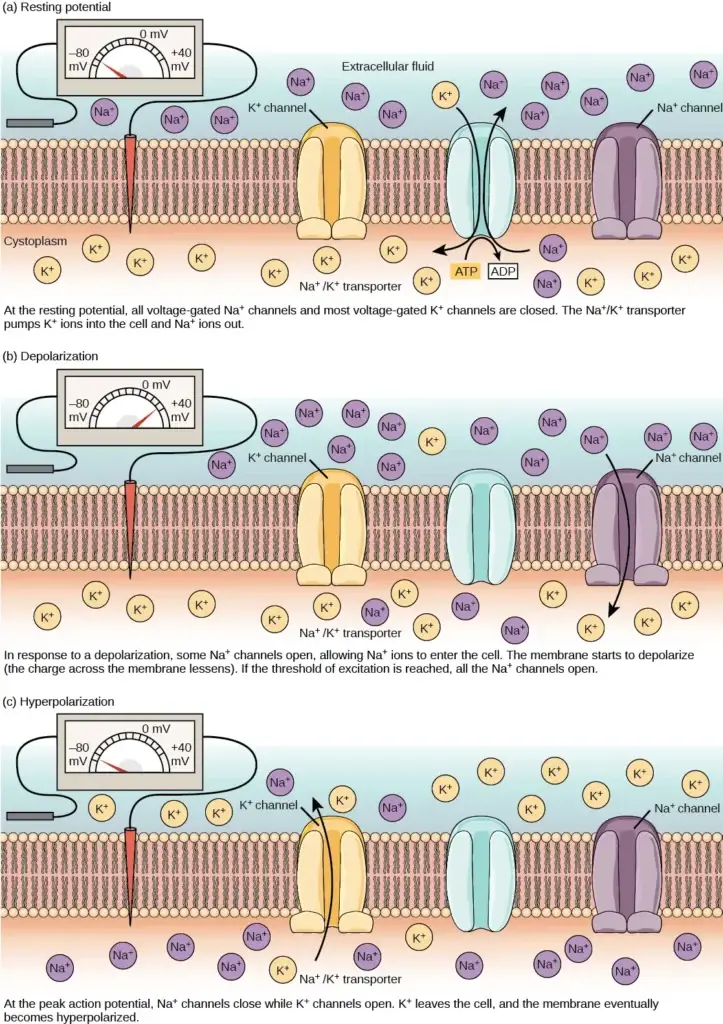
Ion Concentration Inside and Outside Neurons | |||
---|---|---|---|
Ion | Extracellular concentration (mM) | Intracellular concentration (mM) | Ratio outside/inside |
Na+ | 145 | 12 | 12 |
K+ | 4 | 155 | 0.026 |
Cl– | 120 | 4 | 30 |
Organic anions (A-) | — | 100 |
2. The Action Potential
- The resting potential of a neuron refers to its membrane potential when it is not actively transmitting a signal. Neurons communicate through action potentials, which are brief, positive changes in the membrane potential along the neuron’s axon. When a neuron receives a signal from another neuron in the form of neurotransmitters, it causes a change in the membrane potential. This change is mediated by the opening or closing of voltage-gated ion channels, which are channels that respond to changes in membrane voltage.
- The depolarization of the membrane can lead to an action potential if it reaches a certain threshold potential, typically around -55 mV. If the threshold potential is reached, an action potential is initiated at the axon hillock. The stages of an action potential include depolarization, where voltage-gated sodium channels open, allowing sodium ions to enter the axon and making the inside of the cell more positive. This is followed by repolarization, where sodium channels close and voltage-gated potassium channels open, allowing potassium ions to leave the axon and causing the membrane to repolarize. The process continues with hyperpolarization, where the membrane potential dips below the resting potential, and then the resting potential is reset through the activity of the sodium-potassium pump and potassium leak channels.
- Action potentials travel down the axon as a wave of depolarization, and they always proceed in one direction, from the cell body to the synapse. This is due to the refractory period of voltage-gated ion channels, where the channels cannot reopen immediately after closing. Action potentials are all-or-nothing events, meaning they do not vary in magnitude or speed. However, the frequency of action potentials can vary, representing how many action potentials occur in a given time.
- The speed of action potentials can vary between neurons. In invertebrates, the difference is often due to axon diameter, where larger axons have faster conduction. In vertebrates, myelination of the axon plays a significant role. Myelin acts as an insulator and increases the speed of action potential conduction. The nodes of Ranvier, which are gaps in the myelin sheath, allow the action potential to regenerate along the axon. This saltatory conduction, jumping from one node to the next, saves energy for the neuron and speeds up the conduction of the action potential.
- Overall, the resting potential is an essential aspect of neuronal function, providing the baseline electrical state from which action potentials can be generated and propagated, allowing for communication between neurons in the nervous system.
Initiation of Action potential in axon hillock
The initiation steps of an action potential at the axon hillock involve several stages:
- Depolarization: When the membrane potential of the neuron reaches the threshold potential of around -55 mV, voltage-gated sodium channels open rapidly. This allows an influx of sodium ions into the axon, causing the interior of the cell to become relatively electrically positive compared to the initial resting potential of approximately -70 mV.
- Repolarization: Shortly after the depolarization phase, the voltage-gated sodium channels close and remain closed for about 1-2 milliseconds. During this time, voltage-gated potassium channels open, allowing potassium ions to rush out of the axon (efflux). This outflow of potassium ions causes the membrane to repolarize, returning it to a more negative state.
- Hyperpolarization: The efflux of potassium ions during repolarization can cause the membrane potential to dip below the normal resting potential. This hyperpolarization occurs as potassium continues to leave the axon, making the inside of the cell even more negative than the resting potential. Meanwhile, the sodium channels return to their resting state, ready to be opened again if the membrane potential exceeds the threshold in the future.
- Resetting the resting potential: Following hyperpolarization, the sodium-potassium pump and potassium leak channels work together to reset the locations of sodium and potassium ions. The sodium-potassium pump actively transports sodium ions out of the cell while bringing potassium ions back in. This process reestablishes the resting potential of the membrane, allowing it to be ready for another action potential to occur.
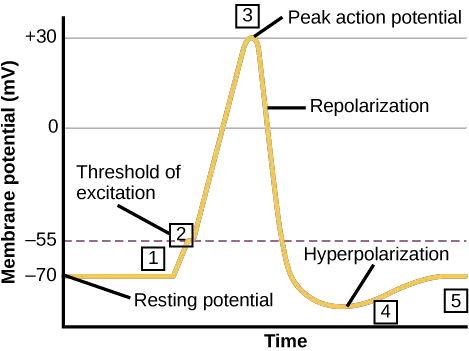
These initiation steps of the action potential at the axon hillock are crucial for the transmission of electrical signals along the neuron’s axon, enabling communication between neurons in the nervous system.
Features of action potentials
Action potentials, or nerve impulses, possess several distinct features:
- Unidirectional propagation: Action potentials travel along the axon in a one-way direction, from the cell body (soma) to the synapse(s) located at the end of the axon. They do not reverse or travel backward. This unidirectional flow is due to the refractory period of voltage-gated ion channels. After an action potential is generated, these channels enter a state where they cannot be reopened for a brief period of 1-2 milliseconds. This refractory period ensures that the action potential proceeds in a forward direction.
- All-or-nothing principle: Action potentials follow the all-or-nothing principle, meaning that they either occur fully or do not occur at all. Once the membrane potential of a neuron reaches the threshold level, an action potential is triggered with a consistent magnitude and speed. The magnitude of an action potential remains the same from initiation to termination, and it always depolarizes the membrane to the same extent. Furthermore, the speed at which the action potential travels along the axon is constant and does not vary.
- Frequency modulation: While the magnitude and speed of an individual action potential remain constant, the frequency of action potentials can vary. Neurons can generate action potentials at different rates, determining the frequency of their firing. The frequency of action potentials encodes information, and the varying frequency can convey different levels or types of signals.
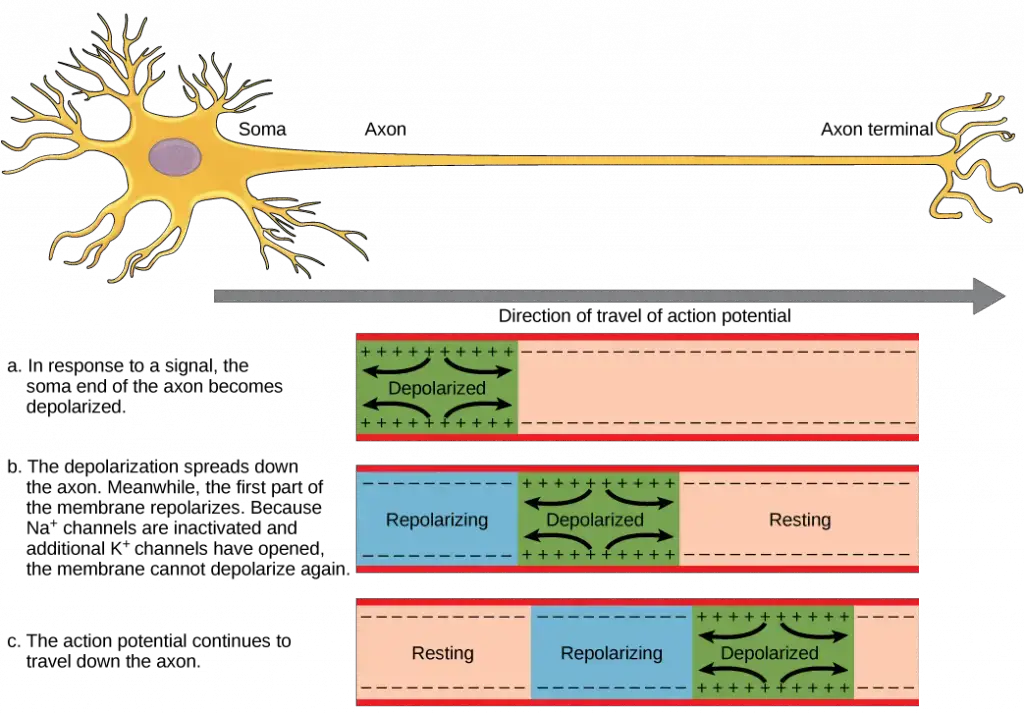
In summary, action potentials are characterized by their unidirectional propagation, all-or-nothing nature, and the ability to modulate their frequency. These features allow for the efficient and reliable transmission of electrical signals along the axon, facilitating communication between neurons in the nervous system.
3. The Chemical Synapse and Neurotransmitters
The chemical synapse is a crucial component in the transmission of signals between neurons. Neurons are not physically connected to each other but instead communicate at specialized structures known as synapses. Within a synapse, there are two key players: the presynaptic neuron, responsible for sending the signal, and the postsynaptic neuron, which receives the signal.
The synaptic cleft, a small gap between the presynaptic and postsynaptic neurons, plays a vital role in the transmission process. It is within this space that neurotransmitters, chemical messengers, are released by the presynaptic neuron to transmit the signal to the postsynaptic neuron.
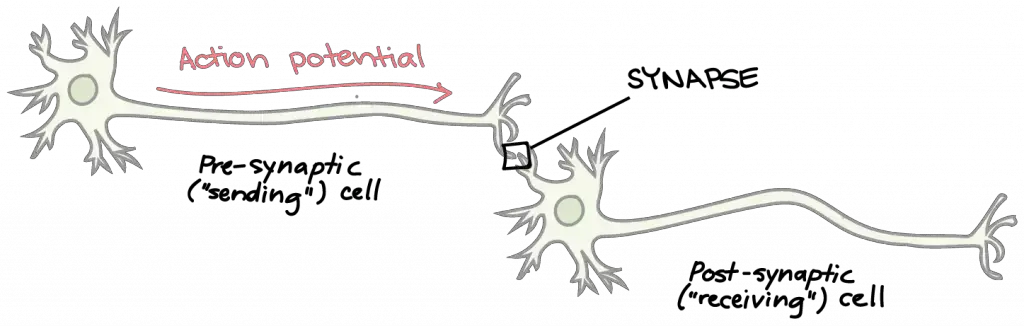
So, how does synaptic transmission occur? When an action potential, an electrical signal, reaches the end of the presynaptic neuron’s axon, a series of events take place:
- The action potential depolarizes the presynaptic membrane by opening voltage-gated Na+ channels. Sodium ions (Na+) flow into the cell, further depolarizing the membrane.
- The depolarization of the presynaptic membrane triggers the opening of voltage-gated Ca2+ (calcium) channels. Calcium ions (Ca2+) enter the presynaptic neuron at the synapse.
- The entry of calcium ions initiates a signaling cascade that leads to the fusion of synaptic vesicles, small membrane-bound vesicles containing neurotransmitter molecules, with the presynaptic membrane.
- Fusion of a vesicle with the presynaptic membrane releases neurotransmitters into the synaptic cleft, the extracellular space between the presynaptic and postsynaptic membranes. The neurotransmitters then diffuse across the synaptic cleft and bind to receptor proteins on the postsynaptic membrane.
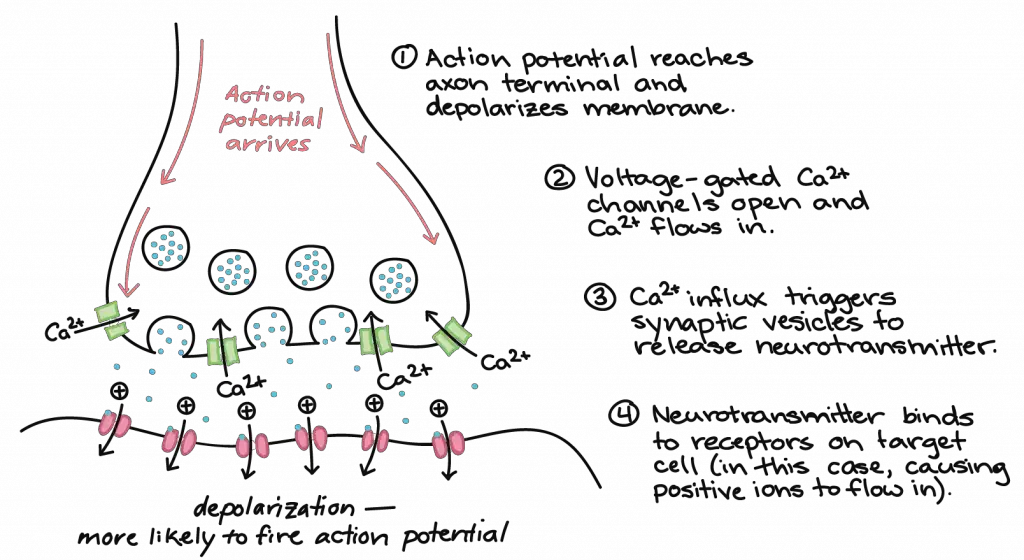
The binding of neurotransmitters to receptors on the postsynaptic membrane can have different effects on the postsynaptic neuron. Excitatory postsynaptic potentials (EPSPs) occur when the binding of neurotransmitters makes the postsynaptic neuron more likely to generate an action potential. For example, acetylcholine released at the neuromuscular junction between a nerve and muscle causes the opening of Na+ channels, leading to depolarization of the postsynaptic membrane.
In contrast, inhibitory postsynaptic potentials (IPSPs) make the postsynaptic neuron less likely to generate an action potential. When the neurotransmitter GABA is released from a presynaptic neuron, it binds to and opens Cl– channels, allowing chloride ions (Cl–) to enter the cell and hyperpolarize the membrane.
After neurotransmission has occurred, the neurotransmitter must be cleared from the synaptic cleft to allow the postsynaptic membrane to reset and be prepared for another signal. This removal can happen in three ways: diffusion of the neurotransmitter away from the synaptic cleft, degradation of the neurotransmitter by enzymes present in the synaptic cleft, or recycling (reuptake) of the neurotransmitter by the presynaptic neuron.
It is important to note that while action potentials are “all-or-nothing,” EPSPs and IPSPs are graded in nature, meaning they can vary in the magnitude of depolarization or hyperpolarization they induce. Often, a single EPSP may not be sufficient to trigger an action potential in the postsynaptic neuron, and multiple presynaptic inputs must produce EPSPs around the same time to achieve sufficient depolarization for firing an action potential. This phenomenon is known as summation and takes place at the axon hillock, the region where the axon connects to the cell body. Additionally, since a neuron receives inputs from multiple presynaptic neurons, some excitatory and some inhibitory, IPSPs can counteract EPSPs, and vice versa. The net change in postsynaptic membrane voltage determines whether the threshold for excitation is reached, ultimately deciding if the postsynaptic cell will generate an action potential.
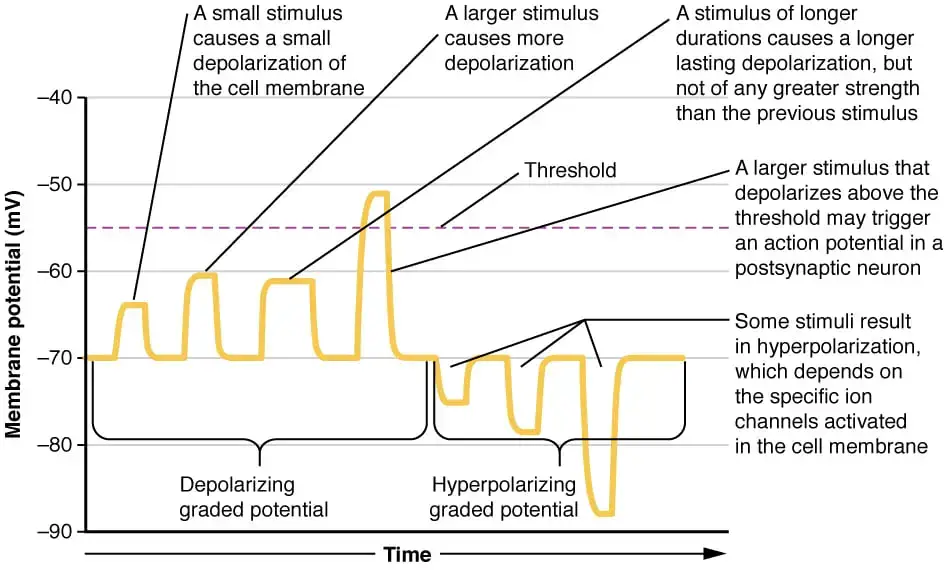
Together, synaptic summation and the threshold for excitation act as a filter, ensuring that only relevant and important information is transmitted while preventing random “noise” in the system from affecting the overall output of the neuron.
What is Reflex Arc?
A reflex arc is a rapid and involuntary response to an external stimulus that occurs through the spinal cord rather than the brain. It is a mechanism that allows the body to respond quickly to potentially harmful or dangerous situations without the need for conscious thought. A classic example of a reflex arc is the withdrawal of a hand from a hot object.
The reflex arc involves several key components:
- Receptor: The receptor is a specialized sensory structure that detects the stimulus. It could be a sensory nerve ending in the skin, for example, that senses heat from the hot object.
- Afferent pathway (sensory neuron): The sensory neuron carries the nerve impulses from the receptor to the central nervous system (CNS), which consists of the brain and spinal cord. In the case of the reflex arc, the sensory neuron transmits the information about the hot object to the spinal cord.
- Interneuron: The interneuron, located within the spinal cord, serves as a connector between the sensory neuron and the motor neuron. It receives the sensory input and relays it to the appropriate motor neuron, which then initiates the response.
- Efferent pathway (motor neuron): The motor neuron carries the nerve impulses from the CNS to the effector, which is usually a muscle or gland. In the case of the reflex arc, the motor neuron transmits the signal from the spinal cord to the muscle fibers responsible for the withdrawal reflex.
- Effector: The effector is the target organ that carries out the response. In the context of the reflex arc, the effector is typically a muscle fiber. When the motor neuron sends the signal, the muscle fibers contract, causing the hand to be pulled away from the hot object.
It’s important to note that spinal reflexes, like the reflex arc, can occur without the involvement of higher brain centers. The entire reflex response is coordinated within the spinal cord itself, allowing for a rapid and automatic reaction to the stimulus.
Reflex arcs play a vital role in protecting the body from harm. They allow for swift responses to potential dangers without the need for conscious thought or decision-making processes, ensuring the safety and survival of an individual.
Glia
Glia, often referred to as the supporting cells of the nervous system, play a crucial role in its proper functioning. In fact, the number of glial cells in the brain is approximately ten times greater than the number of neurons. These glial cells perform essential functions that enable neurons to function effectively. They contribute to neuronal development, protect neurons from harmful substances, provide insulation for axons, and regulate communication between nerve cells. When glia malfunction, it can have severe consequences, as many brain tumors are caused by glial mutations.
There are several distinct types of glia, each with its own specific functions:
- Astrocytes: Astrocytes provide essential support to neurons by supplying them with nutrients and other substances. They also help regulate the concentration of ions and chemicals in the extracellular fluid surrounding neurons. Additionally, astrocytes play a crucial role in synapses by providing structural support. They are responsible for forming the blood-brain barrier, a protective mechanism that prevents the entry of toxic substances into the brain.
- Satellite glia: Satellite glia primarily exist in the peripheral nervous system (PNS). They provide support and nutrients to neurons in the PNS, ensuring their proper functioning.
- Microglia: Microglia are the immune cells of the central nervous system (CNS). Their primary role is to scavenge and degrade dead cells and protect the brain from invading microorganisms. They act as the first line of defense against pathogens or damage within the CNS.
- Oligodendrocytes: Oligodendrocytes are responsible for the formation of myelin sheaths around axons in the CNS. A single oligodendrocyte can provide myelin for multiple neurons, and one axon can be myelinated by several oligodendrocytes. The myelin sheath serves as insulation for the axon, allowing for faster and more efficient transmission of electrical signals.
- Schwann cells: Schwann cells, like oligodendrocytes, play a role in myelination. However, they are found in the peripheral nervous system (PNS). Unlike oligodendrocytes, a single Schwann cell provides myelin for only one axon. The entire Schwann cell wraps around the axon, creating the myelin sheath.
- Ependymal cells: Ependymal cells line the fluid-filled ventricles of the brain and the central canal of the spinal cord. They play a role in the circulation of cerebrospinal fluid, which acts as a cushion for the brain and spinal cord. Ependymal cells help maintain the appropriate balance and flow of cerebrospinal fluid within the central nervous system.
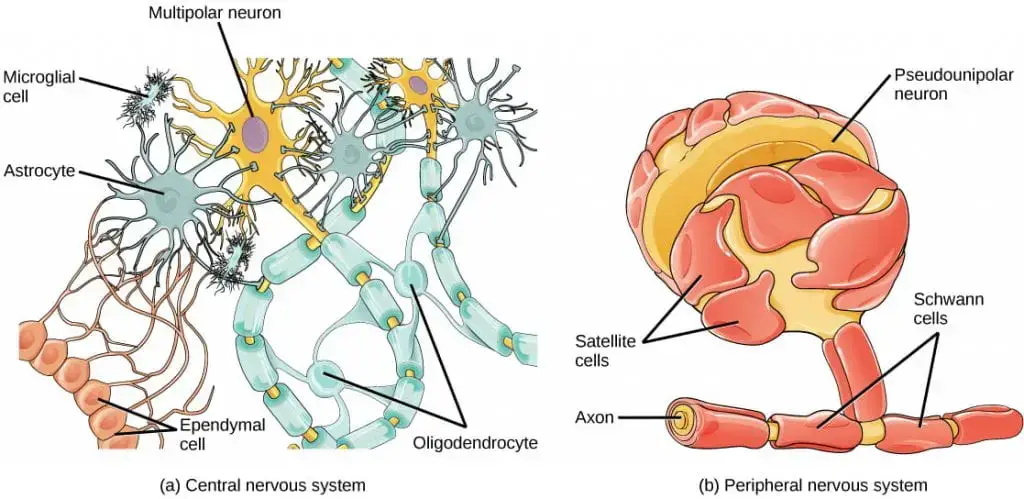
Overall, glia are essential for the proper functioning and protection of neurons in the nervous system. Their diverse functions contribute to the development, maintenance, and overall health of the nervous system, highlighting their critical role in supporting neural processes.
Functions of Neuron
Neurons play a pivotal role in the nervous system by facilitating communication and processing information through electrical signals. This function is essential for various bodily activities and responses to environmental stimuli.
- Information Reception: Neurons receive stimuli from the external environment, which is primarily mediated by sensory neurons. These cells detect changes such as light, sound, temperature, and pressure. By converting these stimuli into electrical signals, sensory neurons transmit this information to the central nervous system (CNS).
- Signal Transmission: The transmission of electrical impulses, known as action potentials, occurs along the axon of the neuron. This process is crucial for communicating information quickly and efficiently across the nervous system. The ability to generate and propagate these impulses is fundamental to all neural activities.
- Motor Control: Motor neurons are responsible for transmitting signals from the CNS to muscles and glands. They play a critical role in initiating and regulating voluntary movements, such as walking and running, as well as involuntary actions like reflexes. By converting neural signals into mechanical actions, motor neurons enable the body to respond to sensory input effectively.
- Interneuron Mediation: Interneurons function as connectors within the CNS, facilitating communication between sensory and motor neurons. They process information and relay signals, thereby integrating inputs from multiple sources. This mediation is vital for complex reflexes and cognitive functions.
- Ionic Balance Maintenance: Neurons are also involved in maintaining the concentrations of ions inside and outside their membranes. This ionic balance is crucial for the generation of action potentials. Specifically, the movement of ions such as sodium and potassium across the neuronal membrane underlies the excitability of neurons and their ability to transmit signals.
- Neurotransmitter Release: Upon reaching the axon terminals, action potentials trigger the release of neurotransmitters into the synapse. This chemical signaling allows communication between neurons, enabling the propagation of signals across synaptic gaps.
Disorders of the Nerve Cells
Disorders of nerve cells encompass a range of conditions that can significantly impact neurological function. These disorders often lead to progressive damage or dysfunction of neurons, affecting various bodily systems. Understanding these disorders is crucial for recognizing symptoms, diagnosing conditions, and providing appropriate treatment.
- Neurodegenerative Disorders: These diseases are characterized by the progressive degeneration of nerve cells and are often associated with aging. Currently, there is no cure, and treatment focuses on symptom management to enhance quality of life.
- Alzheimer’s Disease: This disorder results in a gradual decline in memory and cognitive abilities. Individuals may face challenges with communication, decision-making, and completing daily tasks as the disease progresses.
- Parkinson’s Disease: Characterized by movement difficulties, Parkinson’s leads to symptoms such as tremors, muscle stiffness, and impaired balance. Cognitive decline often follows. Both genetic predispositions and environmental factors contribute to its onset, with aging being a significant risk factor.
- Huntington’s Disease: A genetic condition that leads to the progressive loss of nerve cells, particularly affecting brain regions responsible for voluntary movement. Symptoms include involuntary movements (chorea), atypical postures, and cognitive impairments.
- Motor Neuron Diseases: These disorders specifically affect motor neurons, leading to muscle weakness and, eventually, paralysis. This category includes:
- Amyotrophic Lateral Sclerosis (ALS): A severe form of motor neuron disease that results in the degeneration of motor neurons in the brain and spinal cord.
- Primary Lateral Sclerosis: A rare disorder that affects the upper motor neurons.
- Spinal Muscular Atrophy: A genetic disorder leading to the degeneration of motor neurons, primarily in infants and children.
- Peripheral Neuropathy: This condition affects the peripheral nerves and can result from various causes, including diabetes, alcohol abuse, infections, and genetic disorders. Symptoms often include:
- Muscle weakness
- Uncontrolled muscle movements
- Numbness or tingling
- Imbalance and changes in blood pressure
- Demyelinating Diseases: These disorders involve damage to the myelin sheath that insulates nerve fibers. Common demyelinating diseases include:
- Multiple Sclerosis (MS): Characterized by immune-mediated damage to myelin, leading to symptoms such as vision changes, muscle weakness, and coordination difficulties.
- Guillain-Barré Syndrome (GBS): An acute condition that causes the immune system to attack the peripheral nerves, often leading to rapid-onset muscle weakness.
- Chronic Inflammatory Demyelinating Polyneuropathy (CIDP): A progressive form of neuropathy that results in muscle weakness and sensory loss.
Facts
- Did you know that neurons can transmit signals at speeds of up to 120 meters per second, making them some of the fastest cells in the body?
- Did you know that there are different types of neurons, including unipolar, bipolar, and multipolar, each designed for specific functions?
- Did you know that neurons communicate using electrical impulses called action potentials, which are generated by the movement of ions across their membranes?
- Did you know that the human brain contains approximately 86 billion neurons, forming a complex network that processes all our thoughts and actions?
- Did you know that neurons consume about 20% of the body’s total energy, even though they make up only about 2% of body weight?
- Did you know that new neurons can be generated throughout a person’s life, particularly in areas like the hippocampus, which is important for memory?
- Did you know that neurons communicate chemically through neurotransmitters, which are released into synapses to transmit signals between cells?
- Did you know that neuroplasticity allows neurons to adapt and change their connections based on experiences, playing a key role in learning and memory?
- Did you know that sensory neurons carry information from sensory receptors to the brain, while motor neurons transmit signals to muscles for movement?
- Did you know that glial cells support neurons by providing insulation, nutrients, and maintenance, playing a vital role in overall brain health?
FAQ
What is a neuron?
A neuron is a specialized cell in the nervous system that processes and transmits information through electrical and chemical signals.
What is the structure of a neuron?
A typical neuron consists of three main parts: the cell body (soma), dendrites, and an axon. The dendrites receive signals from other neurons, the cell body integrates these signals, and the axon transmits signals to other neurons.
How does a neuron transmit information?
Neurons transmit information through electrical impulses called action potentials. When a neuron receives a strong enough signal, it generates an action potential that travels down the axon and allows communication with other neurons.
What is the role of neurotransmitters?
Neurotransmitters are chemical messengers that help transmit signals between neurons. They are released from the axon terminals of one neuron and bind to receptors on the dendrites or cell body of another neuron, transmitting the signal across the synapse.
How many neurons are in the human brain?
While the exact number is difficult to determine, it is estimated that the human brain contains around 86 billion neurons.
What are the different types of neurons?
There are several types of neurons, including sensory neurons that transmit information from sensory organs to the brain, motor neurons that carry signals from the brain to muscles, and interneurons that connect and communicate between other neurons.
Can neurons regenerate or repair themselves?
In general, neurons have limited ability to regenerate or repair themselves. However, there is ongoing research in the field of neuroregeneration that explores ways to enhance the regenerative capabilities of neurons.
How do neurons form connections?
Neurons form connections through specialized structures called synapses. When an action potential reaches the axon terminal of one neuron, it triggers the release of neurotransmitters, which bind to receptors on the neighboring neuron, allowing communication and information transfer.
What is the role of neurons in learning and memory?
Neurons play a crucial role in learning and memory processes. When we learn something new, connections between neurons are strengthened, forming neural circuits. These circuits facilitate the storage and retrieval of information, contributing to memory formation.
What happens when neurons malfunction?
When neurons malfunction, it can lead to various neurological disorders and conditions. Examples include Alzheimer’s disease, Parkinson’s disease, epilepsy, and stroke. Understanding how neurons function and interact is essential for studying and treating these conditions.
- Chambers D, Huang C, Matthews G. Neuronal Structure and Function. In: Basic Physiology for Anaesthetists. Cambridge University Press; 2019:189-191.
- Ludwig PE, Reddy V, Varacallo M. Neuroanatomy, Neurons. [Updated 2023 Jul 24]. In: StatPearls [Internet]. Treasure Island (FL): StatPearls Publishing; 2024 Jan-. Available from:
- https://www.ncbi.nlm.nih.gov/books/NBK441977/
- https://qbi.uq.edu.au/brain/brain-anatomy/types-neurons
- https://app.jove.com/science-education/v/10842/concepts/neuron-structure
- https://qbi.uq.edu.au/brain/brain-anatomy/what-neuron
- https://www.simplypsychology.org/neuron.html
- https://www.khanacademy.org/science/biology/human-biology/neuron-nervous-system/a/overview-of-neuron-structure-and-function
- https://sites.duke.edu/apep/module-2-the-abcs-of-intoxication/biology-and-chemistry-connections/neuron-structure-and-function/
- https://content.byui.edu/file/a236934c-3c60-4fe9-90aa-d343b3e3a640/1/module6/readings/neuron_structure.html
- https://testbook.com/biology/neurons-structure-types-diagram
- https://organismalbio.biosci.gatech.edu/chemical-and-electrical-signals/neurons/
- https://www.yaclass.in/p/science-cbse/class-10/control-and-coordination-10298/nervous-system-in-animals-and-humans-10290/re-988a9df9-12c1-4417-a916-1705ec889bff
- https://www.brainfacts.org/brain-anatomy-and-function/anatomy/2012/the-neuron
- https://www.geeksforgeeks.org/neuron/
- https://opentextbc.ca/biology/chapter/16-1-neurons-and-glial-cells/
- https://collegedunia.com/exams/neuron-definition-structure-diagram-parts-functions-biology-articleid-1131
- https://www.tutoroot.com/blog/what-is-neuron-structure-definition-function/
- https://www.getbodysmart.com/nerve-cells/
- https://onlinesciencenotes.com/structure-types-neuron-nervous-tissue/