What is gene expression, and how does it relate to cell specialization?
Gene expression is a fundamental biological process through which the information encoded in a gene is utilized to produce functional products, primarily proteins. This mechanism plays a crucial role in cell specialization, often referred to as cell differentiation, wherein generic cells evolve into distinct types, each with unique functions and characteristics.
- Identical Genomes: All cells within a multicellular organism contain the same set of genes, constituting an identical genome. However, the expression of these genes varies across different cell types, meaning that not all genes are activated in every cell.
- Role of Differential Gene Expression: The phenomenon of cell specialization is driven by differential gene expression. Specific genes are activated in particular cells, leading to the production of proteins that determine the cell’s structure and function. For instance, muscle cells express genes that code for proteins involved in muscle contraction, while nerve cells express genes responsible for nerve impulse transmission.
- Stem Cells and Differentiation: Stem cells exemplify the concept of gene expression in specialization. These undifferentiated cells possess the potential to transform into any cell type within the body. As they differentiate, they selectively activate different genes, resulting in the formation of specialized cell types.
- Regulatory Mechanisms of Gene Expression: Several mechanisms regulate gene expression, ensuring precise control over which genes are activated in response to various signals:
- Transcription Factors: These proteins bind to specific DNA sequences, influencing the transcription process—the initial step of gene expression. Transcription factors can function as activators or repressors, modulating the expression of target genes.
- DNA Packaging: DNA is organized into chromatin, a complex of DNA and proteins. The structural arrangement of chromatin can significantly impact gene expression. Tightly packed chromatin can hinder the binding of transcription factors, thereby repressing gene activity.
- Environmental Influences: External factors, such as temperature, nutrient availability, and salinity, can also affect gene expression. An illustrative case is seen in Himalayan rabbits, where fur color is determined by temperature. At warmer temperatures, the genes for black fur remain inactive, resulting in white fur, while colder conditions activate these genes, leading to the expression of black fur.
- Importance for Multicellular Organisms: The interplay between gene expression and cell specialization is vital for the development and functionality of multicellular organisms. These processes enable the formation of diverse cell types, each tailored to perform specific roles necessary for the organism’s survival. Without the intricacies of gene expression and specialization, the existence of complex life forms would be unattainable.
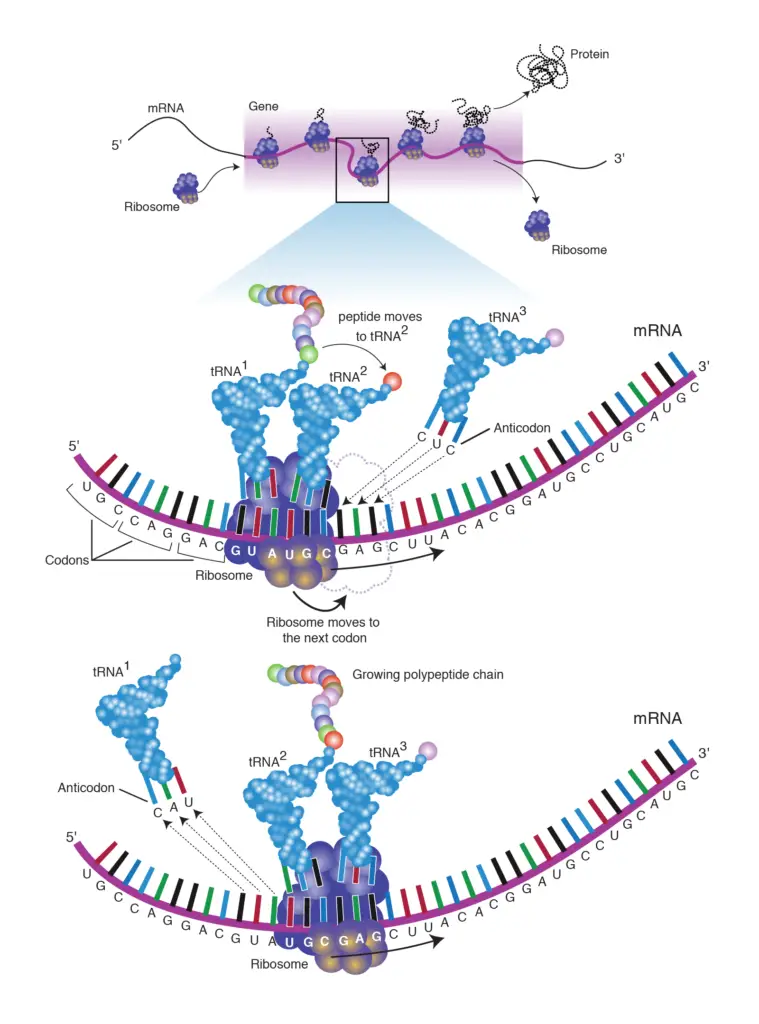
What are the main steps involved in gene expression?
Gene expression is a vital biological process that involves the conversion of genetic information into functional products, mainly proteins. This intricate process consists of two primary steps: transcription and translation. Each step plays a crucial role in ensuring that the correct proteins are synthesized, which are essential for various cellular functions.
- Transcription:
- Definition: Transcription is the initial step in gene expression where the DNA sequence of a gene is copied into a messenger RNA (mRNA) molecule.
- Process:
- Initiation: The process begins when RNA polymerase, an enzyme responsible for synthesizing RNA, binds to a specific region of the gene known as the promoter. This binding marks the starting point for transcription.
- Elongation: Once RNA polymerase is attached, it unwinds the DNA strands and synthesizes a single strand of mRNA by adding complementary RNA nucleotides to the growing chain. For example, if the DNA template has an adenine (A), the RNA will have a uracil (U) instead of thymine (T).
- Termination: Transcription continues until RNA polymerase reaches a terminator sequence, signaling the end of the gene. The newly synthesized mRNA strand detaches from the DNA, completing the transcription process.
- Translation:
- Definition: Translation is the subsequent step in gene expression that involves using the mRNA molecule as a template to synthesize a protein.
- Process:
- Initiation: The mRNA strand binds to a ribosome, the cellular machinery responsible for protein synthesis. The ribosome identifies the start codon on the mRNA, which signals the beginning of the protein coding sequence.
- Elongation: Transfer RNA (tRNA) molecules, each carrying a specific amino acid, enter the ribosome. The anticodon of each tRNA matches with the corresponding codon on the mRNA, facilitating the addition of the amino acid to the growing polypeptide chain. This process continues as the ribosome moves along the mRNA, adding amino acids in the correct sequence.
- Termination: Translation concludes when the ribosome encounters a stop codon on the mRNA. At this point, the completed polypeptide chain is released, and the ribosomal subunits detach from the mRNA.
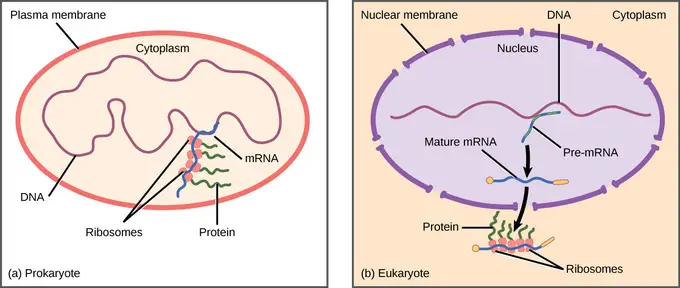
Why is gene regulation crucial in gene expression?
Gene regulation is a fundamental aspect of gene expression, essential for ensuring that the appropriate genes are activated at the right times and within the correct cell types. This regulatory mechanism is crucial in maintaining cellular integrity and functionality, as it prevents unnecessary gene expression that could lead to inefficiencies or cellular damage.
- Uniformity of Genetic Material: Every cell in a multicellular organism possesses the same DNA and genes. However, not all genes are activated simultaneously; this selective expression is vital for the proper functioning of different cell types.
- Role in Cell Differentiation: The specific genes expressed in a cell dictate its structure and function. For instance, muscle cells activate genes that code for proteins associated with muscle contraction, while nerve cells express genes necessary for nerve impulse transmission. Therefore, gene regulation enables cells to acquire specialized roles, contributing to the organism’s overall complexity.
- Mechanisms of Gene Regulation: Various mechanisms govern gene regulation, ensuring precise control over gene expression:
- Transcription Factors: These proteins play a pivotal role in regulating transcription, the initial step of gene expression where DNA is transcribed into RNA. Transcription factors can bind to specific DNA sequences, influencing whether a gene is activated or repressed. This binding can enhance or inhibit the rate of transcription, thereby modulating gene expression.
- DNA Packaging: The structure of DNA within the cell, known as chromatin, can affect gene expression. DNA is packaged into chromatin, and when this chromatin is tightly packed, it can restrict access for transcription factors. This physical arrangement can prevent gene expression by blocking the transcription machinery from binding to the DNA.
- Operons in Prokaryotes: In prokaryotic organisms, genes can be organized into operons, which are clusters of genes controlled by a single promoter. This arrangement allows for the coordinated expression of genes involved in related metabolic pathways. For example, the lac operon regulates the metabolism of lactose in bacteria; it is activated in the presence of lactose and repressed when lactose is absent, thus optimizing resource use.
- Environmental Influences: Gene expression can also be affected by environmental factors such as temperature, nutrient availability, and salinity. An illustrative example is seen in Himalayan rabbits, where fur color changes based on temperature. This adaptability highlights the importance of gene regulation in responding to external conditions.
- Significance for Multicellular Organisms: The ability to regulate gene expression is essential for the development and function of multicellular organisms. This regulation allows for the creation of diverse cell types, each capable of performing specific tasks required for the organism’s survival. Without effective gene regulation, the complexity and functionality of multicellular life would be significantly compromised.
How does gene expression differ between prokaryotes and eukaryotes?
Gene expression is a fundamental biological process that differs significantly between prokaryotes and eukaryotes, primarily due to differences in their cellular structures and complexities. While both groups share the core processes of transcription and translation, the regulation and execution of these processes vary in several key aspects.
- Location and Timing:
- In prokaryotes, which lack a membrane-bound nucleus, transcription and translation occur simultaneously in the cytoplasm. This arrangement allows for rapid protein synthesis, enabling prokaryotic cells to quickly respond to environmental changes.
- In contrast, eukaryotic cells possess a nucleus where transcription occurs. The mRNA must then be transported from the nucleus to the cytoplasm, where translation takes place. This separation of processes introduces additional regulatory levels and results in a delay between transcription and the production of proteins.
- Regulatory Mechanisms:
- Transcription Regulation: In prokaryotes, transcription regulation serves as the primary control mechanism for gene expression. The simplicity of their structure allows for straightforward regulation, often through the use of operons.
- Eukaryotic Complexity: Eukaryotes exhibit more elaborate regulatory mechanisms at multiple stages:
- Epigenetic Regulation: This involves modifications to DNA and histones, affecting chromatin structure and gene accessibility, thereby influencing transcription rates.
- Transcriptional Regulation: Transcription factors play a pivotal role in initiating transcription by binding to specific DNA sequences. Activators enhance transcription, while repressors inhibit it, providing a sophisticated level of control.
- Post-Transcriptional Regulation: This includes processes such as RNA splicing and editing, which can modify the mRNA sequence, impacting subsequent translation and protein production.
- Translational Regulation: Factors that influence ribosome binding and protein synthesis can modulate the rate of translation, allowing cells to respond dynamically to changes in conditions.
- Post-Translational Regulation: After proteins are synthesized, various modifications, such as phosphorylation or glycosylation, can alter their activity and stability, further regulating their function.
- Operons:
- Prokaryotes often utilize operons, which are clusters of genes transcribed together under a single promoter, to control gene expression efficiently. A classic example is the lac operon, which governs lactose metabolism in bacteria. It is activated in the presence of lactose, allowing the bacteria to utilize it as an energy source, while repression occurs in its absence to prevent unnecessary enzyme production.
- Eukaryotes, on the other hand, generally do not utilize operons. Instead, they rely on more complex regulatory mechanisms that facilitate coordinated gene expression across various cell types.
Where do transcription and translation occur in prokaryotic cells, and what is the primary control mechanism?
In prokaryotic cells, transcription and translation occur in the cytoplasm, highlighting a fundamental difference between prokaryotic and eukaryotic organisms. Prokaryotes lack a membrane-bound nucleus, which allows for these processes to happen almost simultaneously.
- Location of Transcription and Translation:
- Both transcription, the process of copying DNA into RNA, and translation, which uses the RNA template to synthesize proteins, take place in the cytoplasm of prokaryotic cells. This proximity enables a rapid response to environmental changes, as the newly synthesized RNA can be immediately translated into protein without the delays associated with nuclear transport.
- Primary Control Mechanism:
- The main regulatory mechanism of gene expression in prokaryotes is the control of transcription. This regulation dictates when and how often a particular gene is transcribed, thereby influencing the production levels of specific proteins. When a protein is no longer required by the cell, transcription is halted, effectively controlling protein synthesis.
- Role of Operons:
- Prokaryotic cells frequently utilize operons as a means to regulate transcription. An operon is a cluster of genes that are transcribed together under the control of a single promoter, allowing for coordinated expression of genes involved in related metabolic pathways.
- A classic example of an operon is the lac operon, which regulates the production of lactase, an enzyme essential for breaking down the sugar lactose. The lac operon is activated in the presence of lactose, ensuring that lactase is produced only when needed. This efficient regulatory mechanism conserves energy and resources, preventing the synthesis of unnecessary enzymes when lactose is not available.
How are these processes spatially separated in eukaryotic cells, and how does this impact gene regulation?
In prokaryotic cells, both transcription and translation occur within the cytoplasm due to the absence of a membrane-bound nucleus. This unique cellular structure enables transcription—the process of copying DNA into messenger RNA (mRNA)—and translation—the synthesis of proteins from mRNA templates—to happen nearly simultaneously.
- Transcription and Translation in the Cytoplasm:
- In prokaryotes, transcription begins in the cytoplasm, where the cell’s genetic material is freely accessible. As mRNA is produced, ribosomes can immediately attach to it, initiating translation without delay. This spatial overlap allows for a rapid response in protein synthesis, particularly useful for adapting to environmental changes swiftly.
- Primary Control of Gene Expression:
- Prokaryotic cells primarily regulate gene expression at the level of transcription. By controlling the initiation and rate of transcription, the cell can effectively manage the synthesis of specific proteins according to its needs. When certain proteins are unnecessary, transcription of the corresponding genes is simply repressed, conserving resources.
- Operons as a Control Mechanism:
- A distinctive regulatory feature in prokaryotes is the operon system, which clusters genes related to a particular function under the control of a single promoter. This arrangement allows coordinated expression of genes that participate in shared metabolic pathways.
- For instance, the lac operon, a well-known operon in bacteria, regulates genes necessary for lactose metabolism. When lactose is available in the environment, the operon is activated to produce enzymes that help in lactose breakdown. In the absence of lactose, the operon is repressed, preventing unnecessary enzyme production and conserving energy.
Why is cell specialization essential in multicellular organisms?
Cell specialization is a critical feature of multicellular organisms, allowing different cell types to perform distinct, necessary functions that contribute to the organism’s overall health and survival.
- Efficient Division of Labor:
- In multicellular organisms, cell specialization means that each cell type has a specific role, unlike a situation where all cells perform identical tasks. Without specialization, every cell would need to carry out all essential life processes, an approach that is both inefficient and limits the size and complexity of organisms.
- Enabling Complexity and Adaptation:
- By having specialized cells, multicellular organisms can grow larger, develop more complex structures, and adapt to various environments. This specialization allows each cell to function optimally in its role, supporting the broader needs of the organism, such as movement, nutrient absorption, and signal transmission.
- Driven by Cell Differentiation:
- Specialization occurs through cell differentiation, a process controlled by gene expression. Although every cell contains the same DNA, specific genes are activated in each cell type, directing it to develop a unique structure and function. For example, transcription factors and DNA packaging help regulate which genes are “turned on” or “off,” ensuring that muscle cells and nerve cells each produce proteins suited to their functions.
- Examples of Specialized Cells:
- In animals, cells such as red blood cells and muscle cells highlight the importance of specialization. Red blood cells are designed to transport oxygen, while muscle cells are built for contraction and movement. In plants, root cells, leaf cells, and stem cells each have distinct roles, from nutrient uptake to photosynthesis, which supports the plant’s survival and growth.
- Essential for Development and Function:
- Cell specialization is fundamental to multicellular life, enabling organisms to grow, adapt, and perform a variety of complex functions. This process of differentiation and specialization ensures that organisms can survive, respond to environmental changes, and carry out a range of life-sustaining activities effectively.
What are stem cells, and how do they contribute to cell specialization?
Stem cells are versatile, unspecialized cells with two main abilities: they can self-renew to produce more stem cells and differentiate into various specialized cell types. This flexibility makes stem cells fundamental for growth, development, and tissue repair in multicellular organisms.
- Unique Potential for Differentiation:
- Unlike mature cells, stem cells start as unspecialized and have the potential to develop into a wide range of cell types. This quality, known as “potency,” varies across different types of stem cells.
- Types of Stem Cells:
- Embryonic Stem Cells:
- Found in early embryonic stages, these stem cells are pluripotent, meaning they can differentiate into nearly any cell type in the body.
- Adult Stem Cells:
- Present in tissues throughout life, adult stem cells are typically multipotent, meaning they can only develop into cell types within a particular tissue or organ. For instance, hematopoietic stem cells in the bone marrow differentiate into various types of blood cells, supporting immune function and oxygen transport.
- Embryonic Stem Cells:
- Mechanisms Driving Cell Specialization:
- Stem cells contribute to the formation of specialized cells through differentiation, a process controlled by two major factors:
- Differential Gene Expression:
- Stem cells contain the same genetic material as all cells in an organism. However, only specific genes are activated during differentiation, leading to the production of unique proteins that shape the cell’s structure and function. This selective gene expression is key to determining a cell’s specialized role.
- Environmental Cues:
- The environment surrounding a stem cell significantly influences its path of specialization. Various factors, such as signaling molecules, physical contact with other cells, and mechanical cues, interact with the cell’s internal pathways. These cues activate specific gene expression patterns, guiding stem cells to develop into specialized cell types.
- Differential Gene Expression:
- Stem cells contribute to the formation of specialized cells through differentiation, a process controlled by two major factors:
- Crucial Roles in Development and Repair:
- Stem cells are essential in embryonic development, giving rise to the diverse cell types required to build complex body structures. In adulthood, they remain vital for tissue repair and regeneration. For instance, stem cells in the skin contribute to wound healing, while bone marrow stem cells continually produce new blood cells to maintain immune health and oxygen transport.
How are stem cells defined, and what is their unique potential?
Stem cells are unique, unspecialized cells distinguished by two fundamental properties: self-renewal and potency.
- Self-Renewal:
- Stem cells have the ability to divide and continuously generate new stem cells, maintaining their population over an organism’s lifetime.
- Potency:
- Potency refers to a stem cell’s potential to differentiate into various specialized cell types, allowing for the creation of diverse cell types essential for an organism’s function.
This dual capability of self-renewal and potency makes stem cells invaluable for growth, development, and tissue repair in multicellular organisms. Stem cells are categorized based on origin and potency:
- Embryonic Stem Cells:
- Found in early developmental stages, embryonic stem cells are pluripotent, meaning they can become any cell type in the body. This flexibility supports their critical role during the early stages of development when the organism’s fundamental body plan is formed.
- Adult Stem Cells:
- Found in specific tissues throughout life, adult stem cells are typically multipotent. They can only differentiate into a limited range of cell types within their tissue of origin. For instance, hematopoietic stem cells in the bone marrow can differentiate into various blood cells necessary for transporting oxygen, supporting immunity, and enabling clotting.
Stem cells contribute to cell specialization, where they transform into specific cell types needed for different functions within an organism:
- Role of Differential Gene Expression:
- Cell specialization relies on differential gene expression, where only a select subset of genes in a cell’s DNA is activated, controlling which proteins are synthesized. This selective activation of genes shapes each cell’s structure and function.
Stem cell differentiation, the process by which stem cells become specialized, is directed by several regulatory mechanisms:
- Transcription Factors:
- Transcription factors are proteins that regulate gene expression by acting as molecular switches. By binding to specific DNA sequences, they either promote or inhibit transcription, the initial stage of gene expression. These factors guide stem cells along particular pathways, ultimately determining their specialization.
- Environmental Cues:
- The external environment of a stem cell, including signals from surrounding cells and physical cues, can significantly influence its fate. External signaling molecules or interactions with neighboring cells can initiate signaling pathways within the stem cell that lead to specific gene activation, guiding it toward a certain specialization.
Stem cells’ unique capabilities position them as central players in:
- Embryonic Development:
- Stem cells are foundational during early development, creating all the diverse cell types required to build the organism.
- Tissue Maintenance and Repair:
- Throughout an organism’s life, stem cells are essential for replenishing and repairing tissues. In bone marrow, they continuously produce new blood cells, contribute to wound healing in the skin, and support regeneration in other tissues as needed.
What are the different types of stem cells, and how does their differentiation potential vary?
Stem cells are categorized based on their source and differentiation potential, which refers to their ability to develop into various specialized cell types. This differentiation potential varies significantly among different types of stem cells, impacting their roles in development and tissue maintenance.
- Embryonic Stem Cells:
- Found in early embryos, specifically within the inner cell mass of a blastocyst, embryonic stem cells are classified as pluripotent. This means they have the remarkable ability to differentiate into any cell type in the body, including muscle cells, nerve cells, and blood cells. Their pluripotency arises during the early stages of development, when the organism’s body plan is being established. Therefore, these stem cells play a critical role in forming all the various cell types necessary for a functioning organism.
- Adult Stem Cells:
- Adult stem cells, also known as somatic stem cells, reside in various tissues throughout the body and are typically multipotent. Unlike embryonic stem cells, multipotent stem cells can differentiate into a more limited range of cell types specific to their tissue of origin. For instance, hematopoietic stem cells located in the bone marrow can give rise to different types of blood cells, including red blood cells, white blood cells, and platelets. The ability of adult stem cells to regenerate and repair tissues is essential for maintaining the body’s health throughout life.
The differentiation potential of stem cells is largely determined by the specific genes that are expressed within each cell type. Although all cells in an organism share the same DNA, the activation or repression of different genes leads to the specialization of cells.
- Control Mechanisms of Differentiation:
- Transcription Factors:
- Transcription factors are crucial proteins that bind to specific DNA sequences to regulate gene expression. They can either activate or repress the transcription of genes, effectively determining which proteins are produced. The presence or absence of certain transcription factors guides stem cells down specific differentiation pathways, influencing their ultimate cell fate.
- Environmental Cues:
- The external environment surrounding a stem cell significantly impacts its differentiation potential. Factors such as signaling molecules, physical interactions with neighboring cells, and various physical cues can modulate gene expression. For example, changes in temperature can influence gene expression patterns in organisms, as seen in Himalayan rabbits, where temperature affects fur color by altering the expression of pigmentation genes. These environmental signals can trigger specific signaling pathways that nudge stem cells toward particular specialized forms.
- Transcription Factors:
What roles do DNA and RNA play in cell specialization?
DNA and RNA are fundamental to the process of cell specialization, transforming unspecialized stem cells into the diverse cell types that form a multicellular organism. Together, they coordinate the complex steps of gene expression that ultimately define a cell’s identity and function.
- DNA:
- DNA, or deoxyribonucleic acid, is often referred to as the blueprint of life, containing the entire genetic code needed to build and maintain an organism. This code is stored in the nucleus of each cell and organized into functional units called genes, each encoding instructions for a particular protein or functional RNA molecule. Therefore, DNA provides a comprehensive set of instructions for cell specialization, as it holds the potential to direct cells into various specialized forms.
- Differential Gene Expression:
- A central principle of cell specialization is not the presence of different DNA in each cell, but rather the selective expression of different genes from this common DNA pool. Known as differential gene expression, this process involves the activation (or repression) of only a subset of genes within a cell’s DNA, enabling each cell to adopt a unique structure and function. For example, muscle cells activate genes coding for proteins responsible for contraction, while nerve cells express genes for proteins involved in signal transmission. This selective gene activation drives each cell’s specialization by producing the specific proteins necessary for its unique role.
- RNA:
- RNA serves as the intermediary between DNA and protein synthesis, enabling the translation of genetic instructions into the proteins that define a cell’s structure and function. The process begins with transcription, during which a DNA sequence of a specific gene is copied into a messenger RNA (mRNA) molecule. This mRNA molecule then exits the nucleus and travels to the cytoplasm, where it is translated into protein by ribosomes. These proteins ultimately serve as the building blocks of the cell, providing the structure and functionality specific to each cell type.
- Regulation of Gene Expression:
- The regulation of gene expression is essential to ensure that the right genes are activated at the right time and in the right cells. This regulation is achieved through a coordinated network of factors that guide cell specialization:
- Transcription Factors:
- Transcription factors are specialized proteins that bind to specific DNA sequences, acting as molecular switches. They either activate (as activators) or suppress (as repressors) the transcription of a gene, determining whether the gene’s instructions are read and translated into a protein.
- Environmental Cues:
- The surrounding environment also plays a crucial role in regulating gene expression. Signals from neighboring cells, interactions within tissues, and other physical cues can influence the gene expression patterns within a cell. These external factors can trigger signaling pathways that activate or repress specific genes, thereby directing cells along specialized pathways.
- Transcription Factors:
- The regulation of gene expression is essential to ensure that the right genes are activated at the right time and in the right cells. This regulation is achieved through a coordinated network of factors that guide cell specialization:
Through the combined action of DNA as the genetic blueprint, RNA as the translator, and intricate regulatory mechanisms, cells are able to achieve specialization. DNA provides the complete set of instructions, while RNA enables the production of proteins that establish each cell’s unique structure and function. This precise regulation of gene expression lies at the heart of cellular specialization, defining the role and identity of each cell within the organism.
What are the mechanisms that regulate gene expression?
Regulating gene expression is a complex, multifaceted process essential for guiding the development of specialized cells from unspecialized stem cells. Through various mechanisms, cells orchestrate gene activation and suppression to ensure precise cellular functions and adaptability.
- Transcription Factors: The Master Regulators
- Transcription factors are proteins that function as molecular switches, determining which genes are transcribed by binding to specific DNA sequences. They can act as activators, promoting gene transcription, or as repressors, blocking transcription.
- The presence or absence of particular transcription factors is crucial in steering a stem cell along specific differentiation pathways, leading to specialization.
- Approximately 10% of genes in eukaryotes code for transcription factors, underscoring their essential role in gene regulation and cellular development.
- Environmental Cues: Influencing Gene Expression
- The surrounding environment significantly impacts gene expression, with factors such as signaling molecules, intercellular interactions, and physical environmental cues triggering internal signaling pathways. These pathways can either enhance or suppress specific gene expressions, contributing to cell specialization.
- Examples include:
- Temperature Changes: In Himalayan rabbits, fur color is temperature-dependent; cooler body areas activate genes for black fur, while warmer regions remain white.
- Metamorphosis in Tadpoles: Tadpoles undergo significant changes during metamorphosis, driven by environmental factors like temperature and resource availability. These cues stimulate hormone release, which in turn triggers gene expression changes necessary for developing specialized cells for the transition to adult frogs.
- Operons: Coordinated Gene Control in Prokaryotes
- In prokaryotes, gene regulation frequently involves operons—clusters of genes under a single promoter, allowing for coordinated expression.
- The lac Operon:
- The lac operon in bacteria regulates lactase production, an enzyme required for lactose breakdown. It includes structural genes coding for lactase and other associated proteins, alongside regulatory elements like a promoter and operator.
- Regulatory Mechanisms: The lac operon is controlled by a repressor protein that binds to the operator region, inhibiting transcription when lactose is absent. When lactose is present, it binds to the repressor, altering its shape and preventing it from binding to the operator, thereby enabling RNA polymerase to bind to the promoter and initiate gene transcription, leading to lactase production.
- DNA Packaging: Impact on Accessibility
- In eukaryotic cells, DNA is compacted by wrapping around histone proteins, forming chromatin structures. The level of chromatin compaction influences gene expression by affecting DNA accessibility.
- Tightly packed chromatin limits access to the DNA, inhibiting gene transcription.
- Looser chromatin provides greater accessibility to regulatory proteins like transcription factors and RNA polymerase, facilitating gene expression.
- In eukaryotic cells, DNA is compacted by wrapping around histone proteins, forming chromatin structures. The level of chromatin compaction influences gene expression by affecting DNA accessibility.
Each of these mechanisms collaboratively fine-tunes gene expression, enabling cells to develop the distinct structures and functions required for specialized roles across an organism.
What is the overall significance of gene expression and cell specialization?
Gene expression and cell specialization are vital processes driving the formation, function, and adaptability of multicellular organisms. Together, they ensure that cells develop specific roles, enabling the organism to thrive in a complex environment.
- From Single Cell to Complex Organism
- All multicellular organisms originate from a single fertilized egg cell. Through successive divisions and regulated gene expression, this single cell gives rise to a vast array of specialized cells that form tissues, organs, and organ systems, each serving distinct purposes.
- This transformation hinges on differential gene expression, where selected genes are activated, or “turned on,” while others remain inactive. By expressing unique gene combinations, cells produce the necessary proteins and molecular components essential for their specialized roles.
- Division of Labor for Efficiency
- Cell specialization creates an internal division of labor, optimizing function and efficiency. Similar to the specialization seen in a structured society, a multicellular organism relies on cells dedicated to specific tasks, improving its capacity to manage diverse biological processes.
- Examples:
- Muscle cells are specialized for contraction, which facilitates movement.
- Nerve cells are tailored for transmitting electrical impulses, allowing communication and coordination.
- Red blood cells transport oxygen throughout the body, supporting cellular respiration.
- In plants, root cells are designed to absorb water and nutrients, while leaf cells carry out photosynthesis.
- Adaptation and Survival
- Specialization enhances an organism’s ability to adapt to environmental challenges, promoting survival. With specialized cells and tissues, organisms are better equipped to respond to changes and external threats.
- Examples:
- Skin cells form a defensive barrier against pathogens and environmental hazards.
- Immune cells actively combat infections.
- In plants, specific cells adapt to manage drought, salinity, and temperature variations, aiding survival in diverse habitats.
- Regeneration and Repair
- Adult stem cells, present in various tissues, are crucial for tissue repair and regeneration. These multipotent stem cells have the potential to differentiate into several cell types within their tissue origin, enabling wound healing and the replacement of damaged cells.
- Medical Advancements
- Knowledge of gene expression and specialization holds substantial promise for medicine and biotechnology.
- Regenerative Medicine: Scientists are investigating the use of stem cells to restore damaged tissues and organs, a potential solution for treating conditions like heart disease, spinal cord injuries, and neurodegenerative disorders.
- Disease Treatment: By understanding gene expression patterns, researchers can develop therapies targeting specific diseases, particularly cancer, where abnormal gene expression and unchecked cell proliferation are defining characteristics.
- Knowledge of gene expression and specialization holds substantial promise for medicine and biotechnology.
Epigenetic Control and Chromatin Remodeling
Epigenetic control and chromatin remodeling are essential mechanisms that regulate gene expression without altering the DNA sequence itself. These processes influence cellular identity, function, and developmental pathways by modulating the accessibility of genes to the transcriptional machinery. Here’s an overview of each and their roles:
1. Epigenetic Control
Epigenetics refers to heritable changes in gene expression that do not involve alterations to the underlying DNA sequence. This regulation is achieved through modifications that affect DNA directly or alter histone proteins around which DNA is wrapped. Key mechanisms of epigenetic control include:
- DNA Methylation:
- In this process, methyl groups are added to cytosine bases, typically at CpG islands (regions with a high frequency of CpG sites) within or near gene promoters.
- DNA methylation generally represses gene expression by preventing transcription factors from binding or by recruiting proteins that compact the chromatin structure, making genes inaccessible for transcription.
- DNA methylation patterns are crucial for cell differentiation and maintaining cellular identity. For instance, genes specific to other cell types are often methylated in differentiated cells to prevent expression of irrelevant functions.
- Histone Modification:
- Histones, the proteins around which DNA is coiled, can be chemically modified to affect chromatin structure and gene accessibility.
- Common modifications include acetylation, methylation, phosphorylation, and ubiquitination, each contributing differently to transcriptional activation or repression.
- Histone Acetylation (often associated with gene activation) adds acetyl groups to lysine residues on histones, reducing the histones’ positive charge and loosening the chromatin structure to allow transcription.
- Histone Methylation can either activate or repress transcription depending on the specific residues modified. For example, methylation of histone H3 at lysine 4 (H3K4me3) is often associated with active gene promoters, while methylation at H3K9 or H3K27 correlates with gene repression.
- Non-coding RNAs:
- Small non-coding RNAs, like microRNAs (miRNAs) and long non-coding RNAs (lncRNAs), can silence genes post-transcriptionally. Some non-coding RNAs also interact with chromatin-modifying enzymes, directly influencing epigenetic states.
- For instance, lncRNAs may recruit chromatin modifiers to specific genomic loci, influencing the expression of targeted genes.
2. Chromatin Remodeling
Chromatin remodeling involves the dynamic restructuring of chromatin, making it more or less accessible to transcriptional machinery. This process is mediated by chromatin remodeling complexes—protein machines that reposition, eject, or restructure nucleosomes (units of DNA wrapped around histones). Major chromatin remodeling activities include:
- ATP-Dependent Chromatin Remodeling Complexes:
- These complexes use energy from ATP hydrolysis to slide, evict, or rearrange nucleosomes. This process can expose DNA regions, such as promoters and enhancers, allowing transcription factors to access and activate specific genes.
- Examples of chromatin remodeling complexes include the SWI/SNF, ISWI, CHD, and INO80 families, each involved in specific developmental processes and cell type-specific gene expression.
- The SWI/SNF complex, for example, is known for its role in gene activation and plays a role in tissue-specific differentiation and cell lineage commitment.
- Histone Variant Incorporation:
- Histone variants, such as H2A.Z and H3.3, replace standard histones in nucleosomes, altering the chromatin structure and its transcriptional properties. For instance, H2A.Z is often found at active promoters and enhancers, making those regions more transcriptionally permissive.
- Histone variants influence cell cycle control, DNA repair, and developmental processes by stabilizing active or repressive chromatin states.
3. Epigenetic Regulation in Development and Disease
- During development, epigenetic modifications and chromatin remodeling ensure proper activation and silencing of genes in a temporally controlled manner. This regulation enables stem cells to differentiate into various cell types, each with a unique gene expression pattern.
- Aberrant epigenetic changes can contribute to diseases, particularly cancer, where genes that normally suppress cell growth may become silenced through hypermethylation, or oncogenes may become active due to hypomethylation and chromatin restructuring.
- Epigenetic therapies, like drugs that inhibit DNA methyltransferases or histone deacetylases, are being explored to reprogram cancer cells or reverse undesired epigenetic states in various diseases.
Role of Gene Expression in Differentiation
Gene expression plays a critical role in cellular differentiation, the process by which cells become specialized in structure and function. This specialization is key to forming the various cell types in multicellular organisms. Here’s a breakdown of how gene expression contributes to this process:
1. Selective Gene Activation and Inactivation
- During differentiation, specific sets of genes are turned on or off in a regulated manner, enabling each cell to develop unique structures and perform specialized functions. This selective expression determines a cell’s phenotype, such as muscle, nerve, or blood cells, which all originate from a common precursor cell.
- Transcription factors (proteins that control which genes are transcribed) are essential in this process, guiding which genes to activate and which to suppress. Each cell type has a unique combination of these transcription factors, leading to a specialized pattern of gene expression.
2. Epigenetic Modifications
- Epigenetic changes, including DNA methylation and histone modification, influence gene expression by making certain genomic regions more or less accessible for transcription. These modifications are stable through cell divisions and ensure that differentiated cells maintain their identity over time.
- For example, a muscle cell will continue expressing muscle-specific genes, while silencing non-muscle genes, due to these stable epigenetic marks.
3. Signaling Pathways and External Cues
- Differentiation often relies on external signals from the cell’s environment, such as growth factors and cytokines. These signals activate intracellular signaling pathways that, in turn, activate or inhibit transcription factors.
- The presence of specific signals guides stem cells or progenitor cells toward particular lineages by promoting the expression of lineage-specific genes.
4. Feedback Mechanisms
- As differentiation proceeds, cells often use feedback mechanisms to reinforce gene expression changes. Positive feedback can solidify a cell’s fate, while negative feedback can prevent the activation of alternative cell fates.
- For instance, once a cell commits to becoming a neuron, it will activate neural-specific genes and often suppress genes associated with other lineages, such as glial cells.
5. miRNAs and Non-coding RNAs
- MicroRNAs (miRNAs) and other non-coding RNAs play regulatory roles by silencing specific genes post-transcriptionally. They help fine-tune gene expression patterns needed for the gradual shift from a multipotent state to a specialized cell type.
- By targeting messenger RNAs of certain genes, miRNAs ensure that only the necessary proteins are translated, contributing to the stability of the differentiated state.
6. Stem Cell Pluripotency and Lineage Commitment
- Pluripotent stem cells, capable of becoming any cell type, must tightly regulate gene expression to ensure proper lineage commitment. Specific transcription factors (e.g., Oct4, Sox2, and Nanog) maintain the pluripotent state, while others promote commitment to differentiated states.
- The transition from a pluripotent state to a committed cell type involves the downregulation of pluripotency genes and the activation of lineage-specific genes.
- https://inspiritvr.com/gene-expression-and-cell-specialization-study-guide/#:~:text=Generic%20embryonic%20cells%20differentiate%20into,determining%20how%20a%20cell%20works.
- https://texasgateway.org/resource/cell-specialization-and-differentiation
- https://www.savemyexams.com/international-a-level/biology/edexcel/18/revision-notes/3-cell-structure-reproduction–development/reproduction–inheritance/3-18-cell-specialisation/
- https://library.fiveable.me/ap-bio/unit-6/mutations/study-guide/WIuGA11Yy2RsVq8JpSnt
- https://studyrocket.co.uk/revision/ap-biology-college-board/gene-expression-and-regulation/gene-expression-and-cell-specialization