Translocation in phloem refers to the process by which photoassimilates, primarily sugars, are transported over long distances within plants. This vital function occurs within the phloem, a type of vascular tissue responsible for distributing the products of photosynthesis from the sites of production to various sinks throughout the plant.
The primary photosynthate transported by phloem is sucrose, a non-reducing disaccharide. Sucrose is favored over reducing sugars like glucose and maltose due to its lower reactivity, which helps in maintaining stability during transport. Other carbohydrates, such as raffinose (a trisaccharide), stachyose (a tetrasaccharide), and verbascose (a pentasaccharide), are also translocated in different plant species. These sugars are essential for maintaining energy distribution and supporting growth and development.
The process of phloem translocation involves the movement of sugars from source tissues, where they are synthesized in the mesophyll cells, to sink tissues, where they are utilized or stored. This movement is facilitated by the phloem tissue, specifically through sieve elements, which include sieve tubes and sieve cells. Sieve tubes, which are found in angiosperms, are characterized by their continuous columns of sieve tube members connected by sieve plates. In contrast, sieve cells, found in gymnosperms and some other plants, are long, slender cells with sieve areas scattered along their length.
The translocation process operates through a mechanism known as pressure flow or mass flow. In this mechanism, sugars are actively loaded into the sieve tubes at the source, creating a high osmotic pressure that drives the flow of phloem sap towards areas of lower pressure at the sinks. This pressure gradient facilitates the efficient transport of nutrients across long distances within the plant.
Understanding phloem translocation is crucial not only for plant biology but also for agricultural practices. It influences crop productivity, yield, and the effectiveness of various agricultural chemicals, including herbicides and fertilizers. Therefore, research into phloem function and translocation processes is vital for improving crop management and agricultural productivity.
Phloem structure
The structure of phloem is integral to its role in transporting nutrients and organic compounds throughout the plant. Phloem tissue comprises several key components, each contributing to its functionality in nutrient distribution.
- Sieve Elements: Sieve elements, including sieve tubes and sieve cells, are the primary conduits for phloem transport. Sieve tubes, found in angiosperms, consist of elongated cells arranged end to end, forming a continuous tube. These cells are characterized by large sieve pores, which are membrane-lined openings facilitating the flow of phloem sap. Sieve cells, present in gymnosperms, share similar functions but have a different structural organization.
- Sieve Plates: The end walls of sieve tubes contain sieve plates, which are perforated with numerous sieve pores. These pores, ranging from 1 to 15 micrometers in diameter, allow for the efficient passage of phloem sap. The development of sieve plates involves the selective degeneration of sieve element protoplasm, leaving behind a largely open, membrane-bound tube.
- Companion Cells: Companion cells are closely associated with sieve elements, forming the sieve element–companion cell complex. These cells are characterized by their dense cytoplasm, rich in ribosomes, mitochondria, and rough endoplasmic reticulum. Companion cells provide the metabolic support necessary for the maintenance and function of sieve elements. They are connected to sieve elements via plasmodesmata, facilitating the exchange of materials and signaling.
- Phloem Parenchyma: Phloem parenchyma cells are involved in the storage and lateral transport of nutrients within the phloem. These cells have a more generalized role compared to sieve elements and companion cells but contribute to the overall functionality of the phloem tissue.
- Phloem Fibres: Phloem fibres provide structural support to the phloem tissue. They are often associated with the xylem and help maintain the mechanical integrity of the plant.
- Transport Mechanism: The translocation of phloem sap occurs through a pressure flow mechanism. This process involves the active loading of sugars into sieve tubes at the source, creating a high osmotic pressure that drives the movement of sap towards sinks where the sugars are utilized or stored.
- Sealing Mechanisms: To prevent loss of phloem sap due to damage, phloem employs rapid sealing mechanisms. In dicotyledonous species, P-protein forms an immediate seal by becoming trapped in sieve pores. Additionally, callose, a polysaccharide, is deposited to block sieve pores in response to wounding or environmental stress. Callose deposition can be reversible, allowing sieve tubes to regain function after damage is repaired.
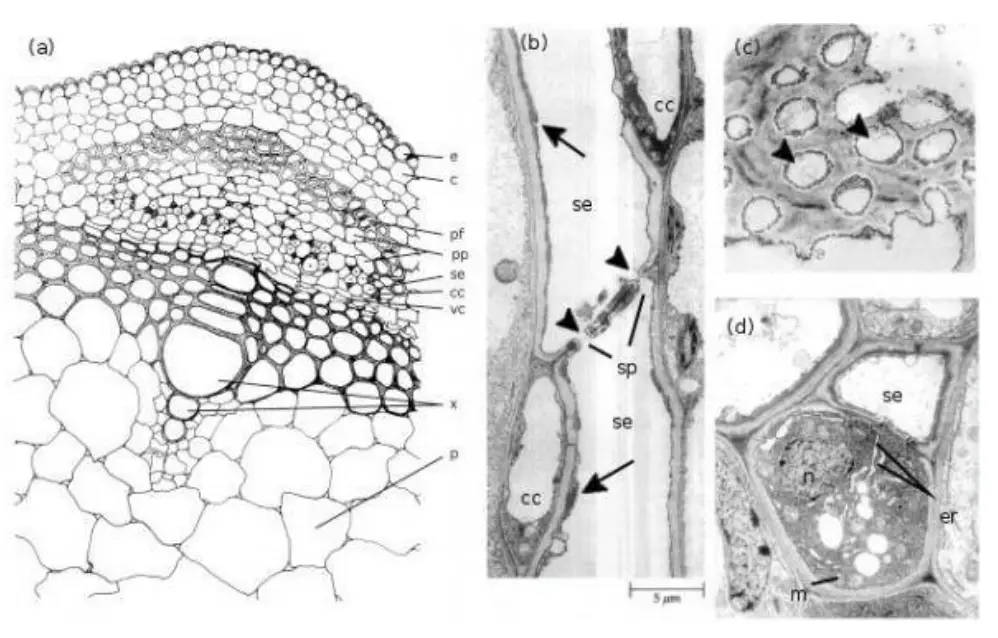
Evidences that translocation occurs through phloem
Evidences supporting the translocation of substances through phloem are derived from various experimental and observational studies. These pieces of evidence underscore the role of phloem in transporting nutrients, particularly sugars, throughout the plant.
- Exudation Through Incision in Bark: When an incision is made in the bark of a deciduous tree, a liquid exudate rich in sugars is observed. This liquid, emerging from the sieve elements, indicates that these cells are actively involved in the transport of nutrients. The presence of high sugar concentrations in the exudate reinforces the role of phloem in distributing photosynthates.
- Ring and Girdling Experiments: Historically, M. Malpighi’s experiments in the 17th century involved removing a ring of bark from a tree, a technique known as girdling. This procedure resulted in a noticeable swelling of the bark above the ring and the development of adventitious roots in that region. The swelling is attributed to the accumulation of photosynthates that could not be transported downward due to the removal of the phloem-containing bark. This evidence demonstrates that phloem is essential for the downward translocation of nutrients and that its removal disrupts this process.
- Analyses of Sap from Aphids: Observations by entomologists have shown that aphids, small sap-sucking insects, feed by inserting their stylets into sieve tubes. The sap extracted by aphids is rich in sugars, confirming that sieve tubes are the primary conduits for the transport of these nutrients. This feeding behavior provides direct evidence of phloem’s role in translocating sugars.
- Evidence from Tracer Techniques: The use of radioactive tracers, such as 14CO2^{14}CO_214CO2, has allowed researchers to track the movement of labeled sugars through phloem. When 14CO2^{14}CO_214CO2 is fixed photosynthetically and its incorporation into sugars is monitored, high concentrations of the labeled sugars are observed in sieve elements. This technique provides compelling evidence that phloem is involved in the translocation of sugars from sources to sinks within the plant.
Theories of translocation
Translocation in plants involves the movement of nutrients, particularly sugars, through the phloem tissue. This process can be categorized into short-distance and long-distance translocation, each explained by different theories.
Short-Distance Translocation Theories
- Protoplasmic Streaming Theory: Proposed by De Vries in 1885 and supported by Curtis in 1935, this theory suggests that solutes move bidirectionally through sieve plates due to protoplasmic streaming, similar to cyclosis. This cytoplasmic movement within sieve elements facilitates the transport of substances over short distances.
- Transcellular Streaming Theory: Introduced by Thaine in 1964, this theory posits that solutes and fluids move linearly along sieve tubes via transcellular strands. These strands move up and down within the sieve tubes, suggesting a linear pathway for solute transport.
- Electroosmosis: Spanner and Jones proposed this theory in 1958 and 1970, which involves the movement of solutes driven by electroosmosis. According to this theory, an electrical current passing through sieve tubes facilitates the translocation of sugars.
- P-Protein Function: Recent evidence indicates that P-proteins, present in sieve elements, play a role in sealing sieve pores during insect invasion rather than promoting translocation. This function is crucial for protecting sieve tubes from damage and maintaining transport integrity.
Long-Distance Translocation Theory
- Pressure Flow Theory: Proposed by Ernst Münch in 1926, this theory explains the movement of solutions through sieve elements driven by an osmotic pressure gradient between source and sink regions. According to Münch’s hypothesis:
- Phloem Loading: At the source tissue, an increase in solute concentration within sieve tubes leads to a rise in osmotic pressure. This osmotic gradient causes water to enter sieve tubes from adjacent xylem vessels, increasing turgor pressure.
- Phloem Unloading: At the sink tissue, solutes are transported out of sieve tubes, decreasing solute concentration and osmotic pressure. This reduction leads to water exiting the sieve tubes and being reabsorbed by nearby xylem vessels, maintaining a continuous flow circuit.
These theories collectively provide a comprehensive understanding of translocation processes in plants. Short-distance theories address the local movement of solutes within sieve tubes, while the pressure flow theory explains the long-distance transport of nutrients from source to sink.
What is Phloem loading?
Phloem loading is a critical process in plants that facilitates the movement of sugars, particularly sucrose, from the photosynthetic cells of the leaf to the sieve tubes in the phloem. This process is crucial for distributing nutrients throughout the plant.
The transport of sugars from mesophyll cells to the sieve tubes can occur through two primary pathways: the symplastic and apoplastic pathways. In the symplastic pathway, sugars move directly from cell to cell through plasmodesmata, which are microscopic channels that connect the cytoplasm of adjacent cells. This movement occurs without leaving the cytoplasm. Alternatively, in the apoplastic pathway, sugars initially move through the cell walls outside the protoplasts before being transported into the sieve tubes.
For sugars traveling via the apoplastic pathway, an active transport mechanism is employed. This process, known as sucrose-H+ symport or cotransport, involves the use of energy derived from ATP. Protons (H+) are pumped out of the sieve tube cells, creating a higher concentration of H+ outside the cell. This concentration gradient drives protons back into the cytoplasm through a sucrose-H+ symporter, which simultaneously transports sucrose from the apoplast into the cytoplasm of the sieve tubes.
The exact mechanism of sucrose transfer from mesophyll cells to the apoplast is not well understood. Phloem loading is selective and varies among plant species. Some plants utilize both symplastic and apoplastic pathways within the same sieve tube elements or between different elements. Variations in phloem loading can also be influenced by factors such as the type of sugar being transported, the type of companion cells present, and the density of plasmodesmata connecting the sieve tubes to surrounding cells.
Experimental research has shown that the patterns of phloem loading are associated with plant family, growth habit, and environmental conditions. Additionally, phloem loading is not limited to leaves; it also occurs in storage organs and at stem nodes where root-produced metabolites are transferred from the xylem to the phloem.
The study of phloem loading has evolved over time, with early research focusing on the export of sucrose from germinating seeds. This understanding has since expanded to encompass various cellular pathways and regulatory mechanisms involved in assimilate loading across different plant species.
Pathway of phloem loading in source leaves
Phloem loading in source leaves involves the transport of photoassimilates from mesophyll cells to the sieve elements and companion cells (se–cc complexes) within the leaf’s vascular system. This process is essential for the distribution of sugars, particularly sucrose, throughout the plant.
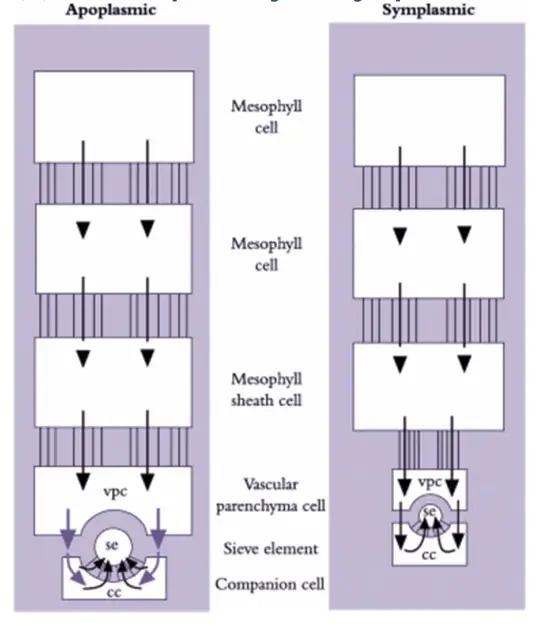
- Transport Pathways:
- Symplasmic Pathway: This involves the movement of photoassimilates through plasmodesmata, which are cytoplasmic channels that connect adjacent cells. In this pathway, sugars move directly from mesophyll cells to the sieve elements through a continuous cytoplasmic network. Symplasmic phloem loading is characterized by extensive plasmodesmal connections and is often found in plant families such as trees, shrubs, and some cucurbits.
- Apoplasmic Pathway: Here, photoassimilates first move through the cell wall continuum before entering the sieve elements. This pathway requires active transport mechanisms, including the sucrose-H+ symporter, which facilitates the movement of sucrose from the apoplast into the cytoplasm of sieve elements. Plants exhibiting apoplasmic loading generally have fewer plasmodesmata connecting sieve elements to companion cells and often belong to herbaceous and crop species.
- Structural Considerations:
- In dicotyledonous leaves, the minor veins, which include a single xylem element, vascular parenchyma cells, and sieve elements surrounded by companion cells, are the principal sites for phloem loading. These veins form an extensive network and are crucial for loading photoassimilates.
- Monocotyledonous leaves, with their parallel vein structures, transport photoassimilates from small veins to larger veins through transverse connections.
- Mechanisms of Loading:
- Symplasmic Loading: This process can be passive or active. In passive symplasmic loading, sugars diffuse through plasmodesmata without a concentrating step. Active symplasmic loading involves biochemical conversion of sucrose to raffinose oligosaccharides (RFOs), which are then trapped in the sieve elements for long-distance transport.
- Apoplasmic Loading: In this pathway, photoassimilates are actively transported across membranes. Experimental evidence, such as the use of fluorescent dyes and the manipulation of sucrose/proton symporters, supports the presence of apoplasmic steps in phloem loading.
- Experimental Evidence:
- Studies using fluorescent dyes and inhibitors have demonstrated the existence of symplasmic pathways. For example, the use of PCMBS (a specific inhibitor) and molecular biology techniques have confirmed the apoplasmic steps in phloem loading, particularly in plants like potatoes.
- Vascular Parenchyma Role:
- Vascular parenchyma cells play a significant role in facilitating the exchange of photoassimilates to the phloem apoplast. These cells ensure direct delivery of photoassimilates to the sieve elements and have been shown to possess increased surface areas to support this transfer.
- Leaf Anatomy and Pathway Variation:
- Some plant species exhibit the potential for simultaneous use of both symplasmic and apoplasmic pathways. The specific pathways used may vary within the same leaf, across different sieve elements, or in different vein orders, highlighting the complexity of phloem loading mechanisms.
Mechanisms of phloem loading
Phloem loading is a fundamental process in plants that involves the movement of photoassimilates from the mesophyll cells to the sieve elements and companion cells (se–cc complexes) in the phloem. This process is characterized by two main mechanisms: symplasmic loading and apoplasmic loading. Each mechanism explains how solutes are concentrated and transported within the phloem.
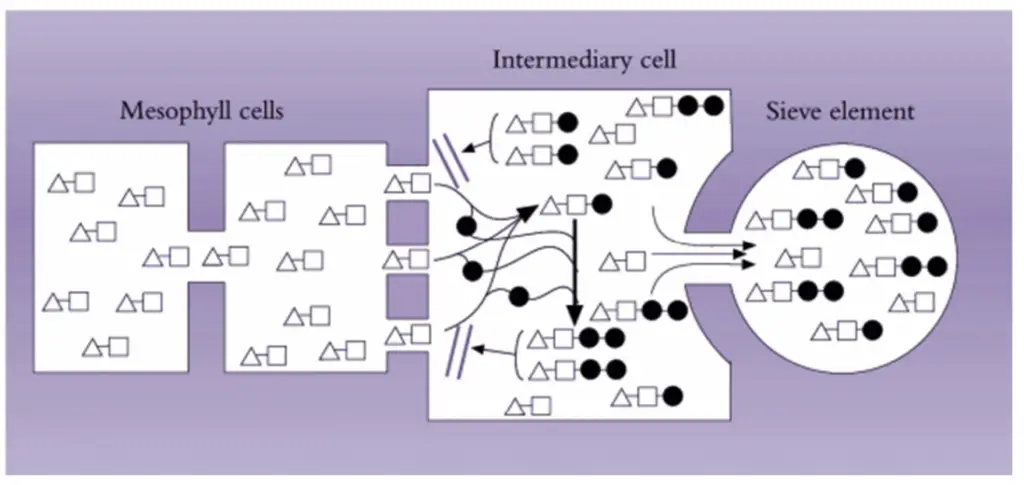
- General Characteristics:
- Elevated Solute Concentration: Phloem loading results in significantly higher solute concentrations in the sieve elements and companion cells compared to the surrounding cells. This elevated concentration is a key feature that any phloem loading mechanism must account for.
- Selective Loading: The loading process is selective, with specific solutes being concentrated in the se–cc complexes. Chemical analyses of phloem sap reveal solute concentrations that differ from those in surrounding cells, indicating a selective mechanism.
- Symplasmic Loading:
- Mechanism: In symplasmic loading, photoassimilates, particularly sucrose, move through plasmodesmata connecting mesophyll cells to companion cells. Within the companion cells, sucrose is converted enzymatically into raffinose oligosaccharides (e.g., raffinose, stachyose). This conversion maintains a concentration gradient that drives sucrose from mesophyll cells into the se–cc complexes.
- Role of Plasmodesmata: Plasmodesmata serve as channels through which sucrose and oligosaccharides are transported. The size-exclusion limit of plasmodesmata prevents the back-diffusion of larger oligosaccharides, ensuring that high concentrations of these compounds accumulate in the phloem. This model explains both the concentration and selectivity of photoassimilates in the phloem.
- Apoplasmic Loading:
- Mechanism: Apoplasmic loading involves the movement of photoassimilates through the cell wall continuum before entering the sieve elements. This process requires energy and is mediated by specific membrane transporters. The sucrose-H+ symporter, which facilitates the active transport of sucrose into the sieve elements, is a key component of this mechanism.
- Energy Dependence: High sucrose concentrations in phloem sap and experimental evidence showing the blockage of photoassimilate loading by PCMBS (a specific inhibitor) indicate that sucrose loading is energy-dependent. Molecular studies have identified sucrose porters in leaf tissues, which support the symport of sucrose and protons.
- Transport Mechanisms: Recent advances in molecular biology, including the cloning of sucrose efflux proteins, have provided insights into the mechanisms of sucrose transport from the apoplasm into the sieve elements. These proteins play a crucial role in facilitating the uptake and distribution of photoassimilates within the phloem.
Sink regulation of phloem loading
The regulation of phloem loading by sink demand is a crucial aspect of nutrient distribution in plants. This regulation determines how photoassimilates are exported from source leaves to various sink tissues, such as fruits, roots, and growing shoots. The following points outline the mechanisms through which sink demand influences phloem loading:
- Sink Effects on Export:
- Source-Limited Systems: In source-limited systems, the capacity for photoassimilate export is constrained by the source’s ability to increase the transport pool. These systems exhibit a slower response to changes in sink demand because the rate of export depends on the ability of leaves to boost their transport capacity.
- Sink-Limited Systems: Conversely, in sink-limited systems, alterations in sink demand result in immediate changes in photoassimilate export. This rapid response is evident when the presence of fruits or high temperatures accelerates the export of 14C-labeled photoassimilates from leaves. For leaves utilizing apoplasmic loading, changes in sink demand likely affect photoassimilate export by modifying membrane transport properties and influencing biochemical partitioning within the leaf.
- Sink Effects on Membrane Transport:
- Turgor Pressure and Phytohormones: Variations in the turgor pressure of phloem sap or changes in phytohormone levels can signal alterations in sink demand. Turgor pressure changes in the phloem sap are quickly transmitted through sieve tubes to source tissues, prompting adjustments in solute transport rates mediated by membrane-associated transporters. This mechanism allows phloem loading to respond swiftly, often within minutes, to shifts in sink demand.
- Phytohormonal Regulation: Phytohormones, such as gibberellins and abscisic acid, also play a role in sink regulation. Gibberellin levels increase in leaves adjacent to developing inflorescences, enhancing photoassimilate export. In contrast, abscisic acid levels often decrease in response to higher sink demand. Direct application of auxins and gibberellic acid to source leaves has been shown to boost photoassimilate export without affecting photosynthesis or partitioning, indicating a direct role in upregulating phloem loading.
- Sink Influences on Biochemical Partitioning:
- Substrate Feedback Response: When sink demand for photoassimilates increases, it can trigger a substrate feedback response. This response involves the acceleration of sucrose export from source pools, which alleviates feedback inhibition on photoassimilate delivery. Subsequently, there is an increase in the activity of key regulatory enzymes involved in sucrose biosynthesis, leading to a higher flow of sucrose into transport pools.
- Photosynthetic Rate Limitation: If photoassimilate flow is limited by the rate of photosynthesis, the enzymes responsible for sucrose biosynthesis are not inhibited by substrate levels. Therefore, the plant must increase the activity of these enzymes to meet elevated sink demand, rather than relying on feedback inhibition mechanisms.
What is Phloem unloading?
Phloem unloading is a critical process in the distribution of photoassimilates within plants, marking the final stage of photoassimilate transport from source leaves to various sink tissues. This process involves the removal of photoassimilates from the phloem and their delivery to sink cells, where they are utilized for growth, metabolism, and storage.
Phloem unloading consists of two main phases: sieve element unloading and post-sieve element transport. Sieve element unloading refers to the movement of photoassimilates from the sieve element-companion cell (se–cc) complexes into the surrounding tissues. Once in the vicinity of sink cells, photoassimilates undergo post-sieve element transport to enter recipient sink cells.
Inside sink cells, photoassimilates are either catabolized through respiratory pathways, used in biosynthesis for maintenance and growth, or stored as macromolecules such as starch and fructans. This metabolic fate is crucial for the plant’s energy balance and development.
The process of phloem unloading and subsequent utilization of photoassimilates can occur across a diverse array of configurations, including morphological structures (e.g., apices, stems, roots, and reproductive organs), anatomical types (e.g., differentiating sieve elements and protophloem sieve elements), developmental stages (e.g., cell division and expansion), and metabolic functions (e.g., storage of soluble compounds and growth). Consequently, various strategies for phloem unloading and sink utilization must be considered to understand the plant’s resource allocation and productivity.
Cellular pathways of phloem unloading
Phloem unloading is a complex process involving the movement of photoassimilates from the phloem into sink cells, and it can follow three primary pathways: apoplasmic, symplasmic, or a combination of both with an apoplasmic step intervening. Each pathway reflects different mechanisms of photoassimilate transport and utilization in various sink tissues.
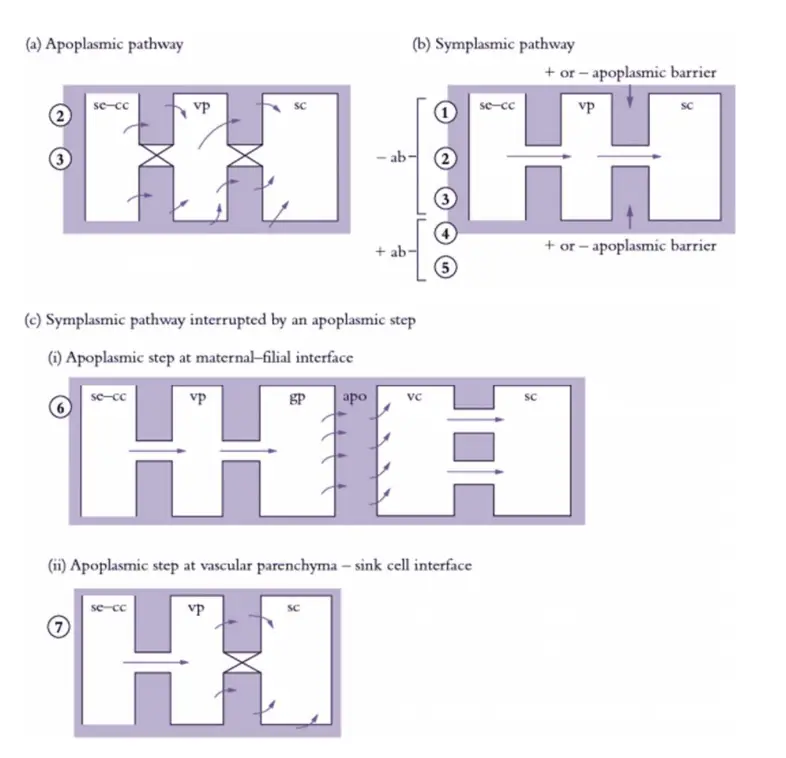
- Apoplasmic Pathways
- Direct Movement: In the apoplasmic pathway, photoassimilates move directly from sieve element-companion cell (se–cc) complexes into the surrounding apoplasm, which is the extracellular space. This pathway is prevalent in roots and stems, where the apoplasm serves as a temporary storage area.
- Function: Apoplasmic unloading is crucial along the axial transport route of these organs, allowing for reversible storage of photoassimilates in vascular parenchyma and ground tissues.
- Symplasmic Pathways
- Symplasmic Transport: In symplasmic unloading, photoassimilates travel through plasmodesmata, the channels connecting adjacent cells. This pathway is observed in various sink types, including terminal growth sinks like root and shoot apices, as well as vegetative storage organs such as stems and tubers.
- Metabolic Conversion: Typically, photoassimilates are metabolized into polymeric forms within the sink cells. However, in some cases like sugar cane, sucrose is stored in its unloaded form in parenchyma cells of stems, reaching high concentrations.
- Symplasmic Pathway Interrupted by Apoplasmic Steps
- Interruption and Exchange: In certain cases, such as developing seeds or tissues with genetic differences (e.g., maternal-filial interfaces), the symplasmic pathway may be interrupted by an apoplasmic step. Photoassimilates are first effluxed into the apoplasm and then taken up by the filial tissues through specialized cells.
- Examples: For instance, in developing seeds, photoassimilates are unloaded from sieve elements and transported symplasmically to effluxing cells, which release them into the seed apoplasm. The filial cells then uptake these photoassimilates from the apoplasm.
- Pathway Linkage with Sink Function and Pathway Switching
- Symplasmic Preference: Generally, symplasmic pathways are favored due to their lower resistance compared to apoplasmic routes. They are commonly engaged unless an apoplasmic step is required due to high solute concentrations or genetic differences between tissues.
- Pathway Switching: An example of pathway switching occurs in tomato fruits. Initially, photoassimilates are unloaded symplasmically to support cell division, but as the fruit develops and accumulates sugars, the process shifts to an apoplasmic route to isolate phloem transport from rising osmotic pressures in storage cells.
- Radial Unloading in Roots and Stems: Depending on the source/sink ratio, the pathway may switch between apoplasmic and symplasmic routes. At low source/sink ratios, apoplasmic unloading might be preferred to avoid futile unloading. At high ratios, plasmodesmal opening facilitates symplasmic unloading into storage pools.
Mechanisms of phloem unloading
Phloem unloading is a crucial process for the distribution of photoassimilates from the phloem to various sink tissues. This process can be categorized into three main mechanisms: apoplasmic transport, symplasmic transport, and symplasmic transport interrupted by an apoplasmic step. Each mechanism plays a distinct role in the efficiency and regulation of photoassimilate distribution.
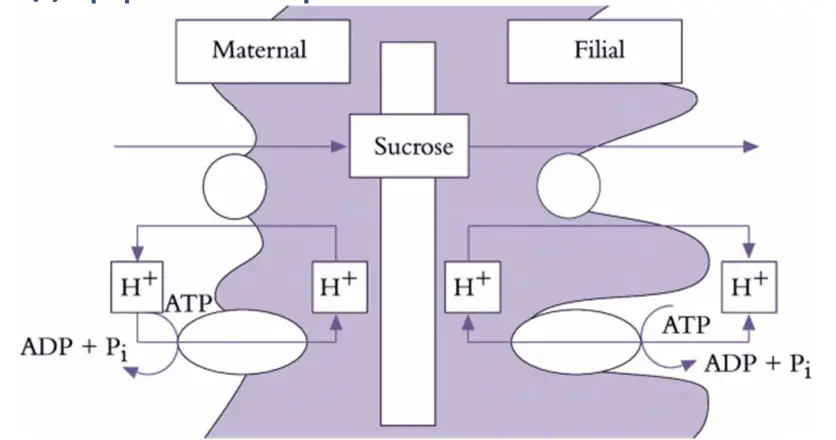
- Apoplasmic Transport
- Mechanism: Apoplasmic transport involves the movement of photoassimilates from sieve element-companion cell (se–cc) complexes into the surrounding apoplasm, the extracellular space. This process is driven by a transmembrane concentration gradient of sugars. Photoassimilates can leak passively from se–cc complexes into the apoplasm due to this gradient.
- Active Retrieval: To maintain efficient unloading, sugars in the apoplasm are often retrieved actively by a sucrose/proton symporter mechanism. This symport system couples the movement of sucrose with protons to transport sugars from the apoplasm into sink cells.
- Influence of Sucrose Metabolism: In some sink tissues, such as developing fruits or roots, extracellular invertase enzymes hydrolyze sucrose, reducing its concentration in the apoplasm and enhancing sucrose unloading from se–cc complexes. This hydrolysis prevents the reuptake of sugars by the symporters and can signal processes like cell division.
- Symplasmic Transport
- Mechanism: Symplasmic transport occurs through plasmodesmata, the cytoplasmic channels connecting adjacent cells. This pathway is often mediated by cytoplasmic streaming and can involve both diffusion and bulk flow mechanisms.
- Diffusion Component: In root tips, for example, diffusion of photoassimilates through plasmodesmata is influenced by concentration gradients. Higher concentrations of photoassimilates in the apoplasm lead to increased movement through the symplasmic pathway.
- Bulk Flow Component: Bulk flow, driven by turgor pressure gradients, also contributes to the movement of photoassimilates. This flow depends on the hydraulic conductivity of plasmodesmata and the pressure difference between source and sink cells.
- Quantitative Models: The rate of diffusion (Rd) through the symplasm is determined by the number of plasmodesmata (n), their conductivity (Kd), and the concentration difference (ΔC). Bulk flow rate (Rf) is calculated based on flow speed (S), cross-sectional area (A), and concentration (C) of sucrose, with flow speed being influenced by hydraulic conductivity (Lp) and turgor pressure difference (ΔP).
- Symplasmic Transport Interrupted by an Apoplasmic Step
- Mechanism: In some systems, such as developing seeds or certain tissue interfaces, the symplasmic pathway is interrupted by an apoplasmic step. This means photoassimilates first move into the apoplasm before being taken up by sink cells.
- Energy Requirements: In legume seed pods, for instance, the energy requirement for sucrose efflux from maternal tissues is not always clear. Facilitated diffusion might be sufficient due to steep concentration gradients. However, energy-dependent mechanisms like sucrose/proton antiporters can be involved in some cases.
- Integration of Demand and Supply: In seeds, photoassimilate demand by filial tissues integrates with the supply from maternal tissues through turgor pressure adjustments. Turgor pressure in seed coat cells can be modulated to regulate the rate of photoassimilate efflux and maintain the balance between supply and demand.
Source–path–sink transport processes
Phloem transport is a critical physiological process in plants, responsible for distributing photoassimilates from their production sites to various sink regions where they are utilized or stored. This process involves a well-coordinated series of events categorized into three main stages: source processes, path processes, and sink processes. Understanding each component of this system helps elucidate how plants manage nutrient allocation efficiently.
(a) Source Processes
- Photoassimilate Production:
- Photosynthesis and Export: The primary source of photoassimilates is fully expanded leaves where photosynthesis occurs. As a leaf develops, its net photosynthetic rate increases, leading to a rise in sucrose export. Initially, a young leaf exports sucrose to meet the demands of growing tissues. Once the leaf reaches approximately 30% of its final size, it begins exporting excess sucrose due to a surplus in photosynthetic production compared to growth needs.
- Sucrose Dynamics: Net sucrose export from the leaf is closely aligned with the photosynthetic rate, peaking when the leaf attains its full size and subsequently declining. The import of minerals like phosphorus continues throughout the leaf’s expansion, with export beginning only after the leaf is fully mature.
- Storage Pools:
- Primary and Secondary Sources: Besides immediate export from mature leaves, photoassimilates are also sourced from storage pools along the phloem pathway, including petioles, stems, and roots. These pools, resulting from the leakage of photoassimilates from vascular tissues, act as secondary sources to buffer the supply to sinks against fluctuations in primary source output.
- Photoassimilate Accumulation:
- In Leaves and Other Tissues: In leaves that do not form starch, high concentrations of sugars may accumulate in the vacuoles of mesophyll cells or be made available for immediate phloem loading. Additionally, nutrients and amino acids from the transpiration stream may be exported directly or stored temporarily before phloem transport.
(b) Path Processes
- Phloem Loading:
- Mechanism: Phloem loading involves the transfer of assimilates such as sucrose and amino acids into sieve elements of the phloem. This process requires overcoming significant concentration and electrochemical gradients and varies among plant species.
- Transport Mechanism: The primary mechanism for longitudinal transport through the sieve elements is mass flow, driven by an osmotic pressure gradient. This gradient is established by a high concentration of solutes at the source end and a lower concentration at the sink end.
- Phloem Translocation:
- Mass Flow: Assimilates move through the sieve elements from the source to the sink by mass flow, which relies on the pressure difference created by the osmotic gradient. This mass flow ensures the efficient delivery of photoassimilates throughout the plant.
- Phloem Unloading:
- At the Sink: Upon reaching the sink, assimilates exit the sieve elements and move into recipient sink cells. Here, they are either metabolized to meet the sink cells’ growth and maintenance needs or stored in vacuoles or polymeric forms. The method of unloading varies depending on the specific functions of the sink organs.
(c) Sink Processes
- Assimilate Utilization:
- Metabolism and Storage: Sink organs typically have low transpiration rates, with the exception of developing leaves. Assimilates arriving at the sink cells are either metabolized to fulfill energy and growth requirements or stored as polymers or in vacuoles.
- Demand-Driven Import:
- Driving Forces: The metabolic activities and storage demands of sink cells create a demand for assimilates, which drives the import process. The rate of phloem import is ultimately regulated by the sink’s capacity to utilize or store incoming photoassimilates.
Regulation of phloem transport
Phloem transport, essential for distributing photoassimilates from source tissues to sink regions, is regulated by a complex interplay of internal and external factors. This regulation ensures that plants effectively manage the flow of nutrients according to developmental needs and environmental conditions. The primary mechanisms of regulation include internal factors such as proximity of sources and sinks, source/sink regulation, and growth hormones, as well as external environmental factors like water stress, mineral deficiencies, light, temperature, CO2 levels, and biotic interactions.
A. Internal Factors
- Proximity of the Source and Sink
- Spatial Relationships: The efficiency of phloem transport is influenced by the spatial arrangement of sources and sinks within the plant. Mature leaves, often positioned higher on the plant, predominantly supply growing shoot tips and young leaves, while lower leaves are more likely to support the root system. Intermediate leaves can export photoassimilates to both directions, bypassing intervening mature leaves.
- Direct Vascular Connections: Source leaves preferentially supply sinks with which they have direct vascular connections, thereby optimizing the transport process and minimizing the distance photoassimilates must travel.
- Regulation by Source and Sink
- Photosynthetic Activity: The rate of phloem transport is closely tied to the photosynthetic activity of source tissues. An increase in photosynthetic rate leads to higher production of triose phosphates, which are converted into sucrose. This process is regulated by the activity of sucrose phosphate synthetase (SPS), which, in turn, is influenced by both sink demands and environmental factors.
- Feedback Mechanisms: When photosynthetic rates are low, the activity of SPS is not inhibited by high sucrose concentrations, leading to increased phloem transport to meet sink demands. Conversely, high sucrose levels in mesophyll cells can inhibit sucrose transporters, affecting phloem loading and photosynthesis.
- Growth Hormones
- Phytohormonal Regulation: Hormones play a critical role in regulating phloem transport. Auxins and cytokinins are known to enhance phloem transport rates, while abscisic acid tends to inhibit it. For example, gibberellins increase photoassimilate export without affecting photosynthesis directly.
- Turgor Pressure: Changes in turgor pressure, influenced by hormone levels, can signal sink demands and adjust the rate of photoassimilate transport accordingly.
B. Environmental Factors
- Water and Salt Stress
- Osmotic Adjustment: Water stress can influence phloem transport by altering osmotic potentials within sieve tubes. Despite adverse conditions, high osmotic potential in the phloem can help maintain sap flow. Osmotic stress can lead to increased sucrose biosynthesis as opposed to starch, aiding in osmotic adjustment.
- Impact on Reproductive Stages: During reproductive stages, water deficits affect the allocation of sugars to flowers, seeds, or fruits, often compromising transport to roots.
- Mineral Deficiency
- Nutrient Allocation: Mineral deficiencies can shift resource allocation within the plant. For instance, nitrogen and phosphorus deficiencies reduce photosynthetic efficiency and carbohydrate transport, while potassium and magnesium deficiencies impact other aspects of plant metabolism.
- Polyol Transport: Polyols, which act as osmotically active and antioxidant molecules, enhance long-distance transport during stress conditions, positively influencing root metabolism and water potential.
- Effect of Light
- Photosynthesis: Light conditions directly impact phloem loading through increased sucrose synthesis and energy provision. Adequate light enhances photosynthetic rates and subsequent sucrose production, optimizing phloem transport.
- Effect of Temperature
- Phloem Loading Modes: Temperature influences the mode of phloem loading. In tropical regions, symplastic loaders dominate, whereas temperate zones often feature apoplastic loaders. Symplastic loaders are more sensitive to cold temperatures compared to apoplastic loaders.
- Effect of CO2
- Carbon Export: CO2 levels affect the export of photoassimilates. While daytime export rates remain stable regardless of CO2 levels, nighttime export rates decrease under normal CO2 conditions but remain unchanged under elevated CO2, indicating higher export to sink organs with increased CO2.
- Biotic Factors
- Microbial Interactions: Both mutualistic and pathogenic microbes impact phloem transport by altering source-sink balance. Pathogens increase sugar demand, which can disrupt the normal flow and distribution of photoassimilates.
- Herbicide Applications: Some strategies, such as using glyphosate, block phloem transport in parasites while leaving the host plant relatively unaffected, thus disrupting sugar transfer to the parasites.
Pressure flow hypothesis
The pressure flow hypothesis, also known as the mass flow hypothesis, provides a widely accepted explanation for the movement of sugars through the phloem. This model, first proposed by Ernst Münch in 1930, describes how a pressure gradient facilitates the bulk flow of photoassimilates from source to sink tissues in plants. While the hypothesis has experimental support, it is important to note that it may not fully apply to non-flowering vascular plants due to structural differences in their phloem systems.
Mechanism of the Pressure Flow Hypothesis
- Loading of Sugars at the Source
- Active Transport: At the source, typically the leaves, sugars (mainly sucrose) are actively transported into the companion cells of the phloem. This process involves specific transport proteins and requires energy in the form of ATP.
- Increased Concentration: The active transport of sugars into the companion cells raises the concentration of sugars within these cells above that found in the adjacent sieve tube elements.
- Diffusion into Sieve Tubes
- Movement into Sieve Tube Elements: The elevated sugar concentration in the companion cells creates a concentration gradient that drives the diffusion of sugars into the sieve tube elements. This movement further increases the sugar concentration within the sieve tubes.
- Water Potential and Osmosis: The increase in sugar concentration decreases the water potential within the sieve tube elements, causing water to enter the sieve tubes by osmosis from surrounding tissues.
- Creation of Positive Pressure
- Fluid Pressure Generation: The influx of water into the sieve tubes results in a positive fluid pressure within the phloem at the source end. This pressure is higher compared to the pressure at distant sink locations.
- Pressure Gradient: The resulting pressure gradient drives the movement of phloem sap through the sieve tubes towards areas of lower pressure, i.e., the sinks.
- Unloading at the Sink
- Sugar Utilization and Pressure Reduction: At the sink, which could be growing tissues or storage organs, sugars are unloaded from the phloem sap. This unloading reduces the concentration of sugars in the sieve tube elements at the sink.
- Continuous Flow: The decrease in sugar concentration at the sink leads to a reduction in water potential and thus pressure. This pressure reduction at the sink maintains the pressure gradient, ensuring the continuous bulk flow of the phloem sap from source to sink.
Importance of Translocation in Phloem
- Nutrient Distribution
- Resource Allocation: Translocation ensures that essential nutrients produced in photosynthetically active regions (sources) are efficiently distributed to non-photosynthetic parts of the plant (sinks). This distribution is critical for the growth and development of roots, fruits, seeds, and other expanding tissues.
- Uniform Growth: By providing a steady supply of sugars and other nutrients, translocation supports uniform growth and development across different plant parts, helping maintain structural integrity and overall plant health.
- Energy Supply
- Metabolic Fuel: Sugars and other photoassimilates transported through the phloem serve as the primary energy source for various metabolic processes in sink tissues. These processes include cell division, elongation, and the synthesis of proteins and other organic compounds.
- Storage and Utilization: In storage organs such as roots and tubers, translocated sugars are converted into storage forms like starch, which can be mobilized when needed for growth, reproduction, or stress responses.
- Growth and Development Regulation
- Developmental Coordination: The translocation of photoassimilates helps coordinate developmental processes by supplying growing regions with the necessary resources. This is particularly important during periods of rapid growth or reproductive development.
- Source-Sink Relationships: Translocation maintains the balance between source and sink activities, ensuring that growth demands are met and preventing nutrient imbalances that could hinder plant development.
- Response to Environmental Changes
- Adaptation to Stress: Translocation helps plants adapt to environmental stress by reallocating resources. For example, under drought conditions, plants may redirect sugars from less critical tissues to roots to enhance water uptake and survival.
- Seasonal Adjustments: During seasonal changes, such as winter dormancy or flowering periods, translocation adjusts nutrient flow to support specific plant needs, such as preparing storage organs for winter or providing resources for seed development.
- Intercellular Communication
- Signaling: The movement of photoassimilates through the phloem also facilitates the transport of signaling molecules that can influence growth and developmental responses in distant tissues. This intercellular communication helps synchronize plant functions and responses to internal and external cues.
- https://www.egyankosh.ac.in/bitstream/123456789/16371/1/Unit-14.pdf
- https://mccollegeonline.co.in/attendence/classnotes/files/1585912976.pdf
- https://www.scribd.com/document/210110998/Translocation
- https://www.mpgmahavidyalaya.org/userfiles/Phloem%20Loading%20and%20Unloading%20in%20Plants.pdf
- https://epgp.inflibnet.ac.in/epgpdata/uploads/epgp_content/botany/01._plant_physiology-ii/phloem_transport/et/5853_et_phloemtransportet.pdf
- https://www.asps.org.au/wp-content/uploads/Chapter-5.pdf
- http://www.lscollege.ac.in/sites/default/files/e-content/Translocation%20of%20solutes.pdf
- https://www.ncbi.nlm.nih.gov/pmc/articles/PMC439437/pdf/plntphys00306-0126.pdf
- https://edurev.in/p/62209/Lecture-5-Translocation-in-Phloem
- http://www.eagri.org/eagri50/PPHY261/lec05.pdf
- https://www.narajolerajcollege.ac.in/document/sub_page/20210125_225210.pdf
- https://www.internationalscholarsjournals.com/articles/note-on-translocation-activity-in-plants.pdf
- https://ncert.nic.in/ncerts/l/kebo111.pdf