What is Neuronal Membrane?
- The neuronal membrane plays a crucial role in the transmission of signals within the nervous system, serving as the foundation for how neurons communicate with each other. It acts as a barrier between the inside and outside of the neuron, allowing it to maintain an electrical charge difference known as the resting membrane potential. This resting potential is essential for the neuron to be ready to transmit signals when needed.
- The membrane itself is selectively permeable, meaning that it controls which ions, such as sodium (Na+), potassium (K+), and chloride (Cl-), can pass through it. This ion movement is critical for generating electrical signals. In its resting state, the inside of the neuron is negatively charged compared to the outside due to the distribution of ions. When the neuron is stimulated, the membrane allows for the rapid movement of ions, causing a temporary reversal of the charge—this is known as the action potential.
- Action potentials are the primary way neurons communicate over long distances. These electrical signals travel down the axon without diminishing in strength, which allows neurons to send information rapidly and accurately. The action potential is an all-or-nothing response, meaning that once it is triggered, it will propagate along the axon to its target without losing intensity.
- The neuronal membrane’s ability to generate and propagate action potentials is crucial for processes like reflexes. For instance, when you step on a thumbtack, the pain signal is quickly transmitted from the sensory neurons in your foot to the spinal cord. There, interneurons relay the signal to your brain and motor neurons, which trigger the withdrawal of your foot. This rapid signaling is made possible by the excitable nature of the neuronal membrane.
Components of Neuronal Membrane
Cytosol and Extracellular Fluid
The cytosol and extracellular fluid are essential environments for neuronal function, as they provide the medium for the movement of ions, which generate the electrical signals vital to neuron activity. The presence of water, along with ions dissolved in it, plays a critical role in facilitating these electrical potentials.
- Water as the Main Component
- Water is the primary substance in both the cytosol (inside the cell) and the extracellular fluid (outside the cell). Its unique properties make it an excellent solvent for ions, which are crucial for neuronal signaling.
- Polarity of Water: Water molecules are polar, meaning they have an uneven distribution of electrical charge. The oxygen atom in H₂O has a stronger attraction to electrons than the hydrogen atoms, making the oxygen slightly negative and the hydrogen slightly positive.
- Polar Covalent Bonds: Water molecules are held together by polar covalent bonds. This polarity allows water to dissolve other polar substances, including ions, which is essential for the movement of charged particles in the neuron.
- Ions and Their Role
- Ions, which are electrically charged atoms or molecules, are responsible for generating electrical potentials in neurons. These ions are dissolved in the cytosol and extracellular fluid, enabling neuronal communication.
- Ionic Bonds: For example, table salt (NaCl) consists of sodium (Na⁺) and chloride (Cl⁻) ions held together by ionic bonds. When salt dissolves in water, the ions separate because water’s polar molecules have a stronger attraction to the ions than the ions have for each other.
- Spheres of Hydration: When ions dissolve, they become surrounded by water molecules, forming “spheres of hydration.” These water molecules insulate the ions from each other, allowing them to move freely in solution. Sodium ions are surrounded by oxygen atoms (negative poles), while chloride ions are surrounded by hydrogen atoms (positive poles).
- Charge and Classification of Ions
- The electrical charge of ions plays a fundamental role in neuronal signaling. The difference between the number of protons and electrons in an atom determines its charge.
- Monovalent and Divalent Ions: Monovalent ions have a charge difference of 1 (e.g., Na⁺), while divalent ions have a charge difference of 2 (e.g., Ca²⁺).
- Cations and Anions: Positively charged ions are called cations (e.g., Na⁺), and negatively charged ions are called anions (e.g., Cl⁻). These ions are the key charge carriers in neurons, allowing for the conduction of electrical signals across cell membranes.
The Phospholipid Membrane
The phospholipid membrane is essential for maintaining the structure and function of neurons, creating a barrier that regulates the movement of substances between the inside and outside of the cell. The membrane’s unique composition of phospholipids plays a key role in separating the intracellular cytosol from the extracellular fluid, making it crucial for maintaining the neuron’s resting and action potentials.
- Phospholipids as the Building Blocks
- Phospholipids are the primary components of the neuronal membrane. They have both hydrophilic and hydrophobic properties that dictate how they interact with water and other molecules.
- Hydrophilic Head: The “head” of a phospholipid contains a phosphate group, which is polar. Because of this polarity, it is hydrophilic, meaning it attracts water and interacts with the aqueous environments inside (cytosol) and outside (extracellular fluid) the cell.
- Hydrophobic Tail: The “tail” of the phospholipid consists of long hydrocarbon chains, which are nonpolar. These tails are hydrophobic, meaning they repel water and avoid interactions with water molecules.
- Phospholipid Bilayer Structure
- The phospholipids in the neuronal membrane are organized into a bilayer, which serves as a fundamental barrier between the cytosol and extracellular fluid.
- Bilayer Arrangement: The hydrophilic heads of the phospholipids face outward, toward the water-based environments on both sides of the membrane, while the hydrophobic tails face inward, avoiding water and forming a barrier in the middle.
- Barrier Function: This bilayer structure isolates the neuron’s internal environment from the external surroundings. It prevents water-soluble ions and molecules from freely passing through, ensuring that the neuron maintains its electrical potentials.
- Function in Neuronal Signaling
- The phospholipid membrane plays an active role in maintaining the electrical signals necessary for neuron communication.
- Selective Permeability: While the bilayer blocks most ions and polar molecules, it allows certain molecules, such as oxygen and carbon dioxide, to pass through. Special proteins embedded in the membrane help regulate the movement of ions like sodium and potassium, which are critical for generating action potentials.
- Role in Resting and Action Potentials: The membrane’s ability to control ion flow ensures that the neuron can maintain a negative resting membrane potential. During an action potential, changes in ion permeability lead to rapid shifts in membrane charge, enabling signal transmission along the axon.
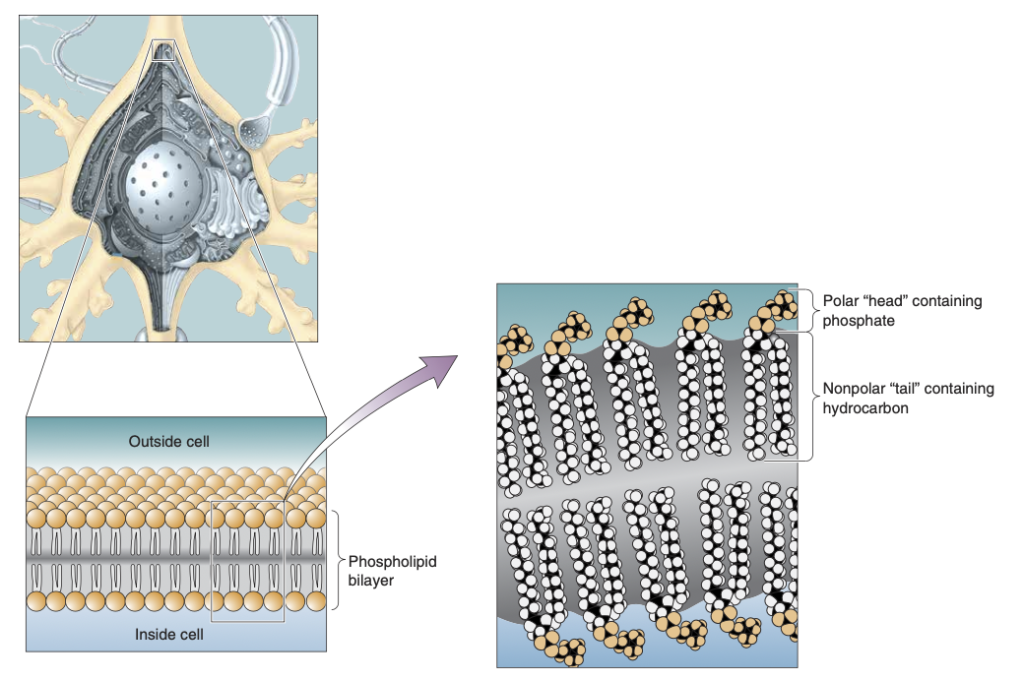
Protein
The neuronal membrane is integral to the function of neurons, characterized by a unique array of protein molecules that distinguish them from other cell types. These proteins facilitate essential functions such as chemical reaction catalysis, structural support through the cytoskeleton, and neurotransmitter reception. Moreover, they play a crucial role in establishing and propagating resting and action potentials by enabling ion movement across the membrane.
- Protein Diversity in Neurons
- Neuronal proteins exhibit a wide range of shapes, sizes, and chemical characteristics, reflecting their diverse functions.
- Proteins are constructed from 20 different amino acids, each containing a central carbon atom, an amino group, a carboxyl group, and a variable R group.
- The unique properties of the R groups determine the behavior and interactions of each amino acid, contributing to the functional diversity of neuronal proteins.
- Neuronal proteins exhibit a wide range of shapes, sizes, and chemical characteristics, reflecting their diverse functions.
- Protein Synthesis and Structure
- Proteins are synthesized in the ribosomes of the neuronal cell body, where amino acids are linked by peptide bonds to form polypeptide chains.
- Primary structure refers to the linear sequence of amino acids.
- Secondary structure involves the coiling of the chain into structures like alpha helices, driven by hydrogen bonding.
- Tertiary structure results from interactions among R groups, leading to complex three-dimensional shapes.
- Quaternary structure arises when multiple polypeptide chains interact to form a larger functional protein.
- Proteins are synthesized in the ribosomes of the neuronal cell body, where amino acids are linked by peptide bonds to form polypeptide chains.
- Channel Proteins
- Channel proteins are crucial for ion transport across the neuronal membrane, featuring regions that are hydrophobic and hydrophilic.
- Typically, four to six protein subunits assemble to create a functional ion channel.
- Ion channels exhibit selective permeability; for instance, potassium channels allow only potassium ions to pass through, while sodium channels are selective for sodium ions.
- Gating mechanisms enable these channels to open or close in response to changes in the membrane’s microenvironment, which is essential for neuronal signaling.
- Channel proteins are crucial for ion transport across the neuronal membrane, featuring regions that are hydrophobic and hydrophilic.
- Ion Pumps
- In addition to channels, some membrane proteins form ion pumps, which are critical for maintaining ion gradients across the membrane.
- These pumps utilize the energy from ATP hydrolysis to transport ions against their concentration gradients, thus playing a key role in neuronal signaling and excitability.
- Ion pumps contribute to the establishment of resting potential and are vital for the proper functioning of neurons.
- In addition to channels, some membrane proteins form ion pumps, which are critical for maintaining ion gradients across the membrane.
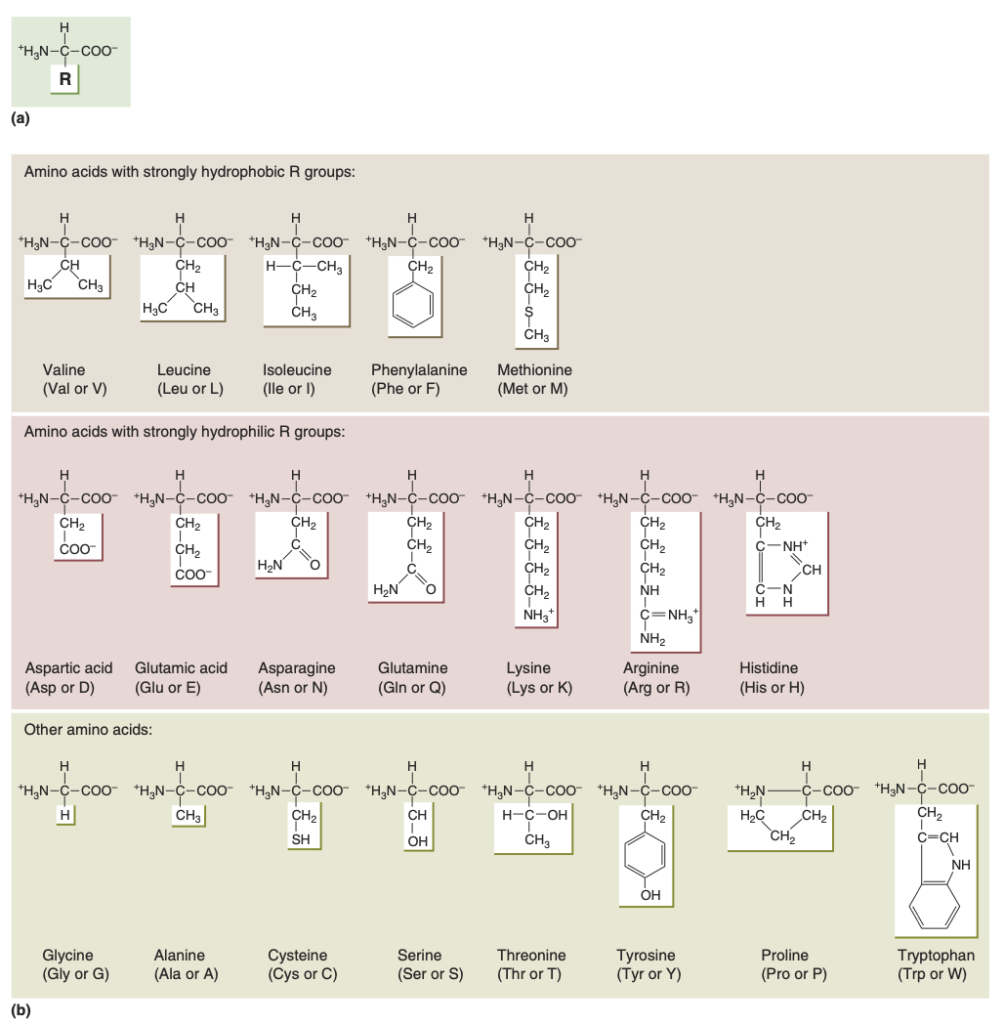
The movement of ions
The movement of ions across neuronal membranes is fundamental to the functioning of the nervous system. Understanding how ions traverse these membranes involves exploring the principles of diffusion and electrical forces, both of which drive ionic movement in specific directions.
- Basic Principles of Ion Movement
- The movement of ions can be likened to crossing a bridge; channels in the membrane provide pathways for ions to travel.
- However, the mere presence of these channels does not guarantee ion movement; external forces are required to facilitate this process.
- Therefore, two primary factors influence ionic movement: concentration gradients (diffusion) and electrical gradients (electricity).
- The movement of ions can be likened to crossing a bridge; channels in the membrane provide pathways for ions to travel.
- Diffusion
- Ions and molecules are in constant motion, driven by thermal energy. This random movement results in a net flow from areas of higher concentration to areas of lower concentration, known as diffusion.
- For instance, when sodium chloride (NaCl) is dissolved in water, Na⁺ and Cl⁻ ions will naturally spread to equalize their concentrations across the solution.
- This net movement occurs when there are channels permeable to the ions and a concentration gradient exists across the membrane. The result is that ions flow down their concentration gradients.
- Ions and molecules are in constant motion, driven by thermal energy. This random movement results in a net flow from areas of higher concentration to areas of lower concentration, known as diffusion.
- Electrical Forces
- In addition to diffusion, electrical fields can induce ion movement due to the charge of the ions. Charged particles are influenced by electrical potential differences, which cause them to move toward oppositely charged areas.
- For example, in a solution containing NaCl, Na⁺ ions will migrate toward the negative terminal of a battery (cathode), while Cl⁻ ions will move toward the positive terminal (anode).
- This movement constitutes electrical current, which is defined by the relationship between potential (voltage) and conductance. Voltage (V) represents the difference in charge, while conductance (g) indicates the ability of charged particles to migrate.
- In addition to diffusion, electrical fields can induce ion movement due to the charge of the ions. Charged particles are influenced by electrical potential differences, which cause them to move toward oppositely charged areas.
- Ohm’s Law
- Ohm’s Law relates current (I) to voltage (V) and conductance (g) through the equation I = gV.
- A higher voltage will increase current flow if conductance is present. Conversely, even a large voltage will not generate current if the conductance is zero due to lack of available channels.
- For ions to cross a membrane, two conditions must be met: the membrane must contain specific ion channels, and a voltage difference must be established across the membrane.
- Ohm’s Law relates current (I) to voltage (V) and conductance (g) through the equation I = gV.
- Ion Channels and Conductance
- Ion channels facilitate the selective passage of specific ions, making the movement highly regulated.
- The permeability of these channels, combined with existing concentration and electrical gradients, determines the net flow of ions.
- Thus, both diffusion and electrical forces work in concert to control ionic movements, essential for establishing resting membrane potentials and initiating action potentials in neurons.
- Ion channels facilitate the selective passage of specific ions, making the movement highly regulated.
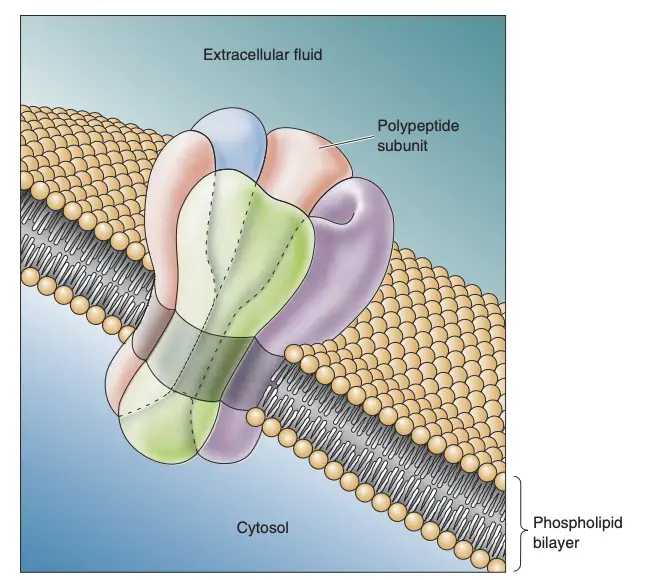
The ionic basis of the resting membrane potential
The resting membrane potential (Vm) of a neuron is a critical aspect of its physiological state, representing the electrical charge difference across the neuronal membrane when the neuron is not actively transmitting signals. This potential is essential for various cellular functions and plays a pivotal role in the generation of action potentials.
- Definition and Measurement of Resting Membrane Potential
- The resting membrane potential is quantified as approximately -65 millivolts (mV), indicating that the interior of the neuron is negatively charged relative to the outside.
- This potential can be measured using a microelectrode, which is a fine glass tube filled with an electrically conductive solution. The microelectrode is inserted into the neuron, allowing for the measurement of the electrical potential difference between the inside of the neuron and the external environment.
- The resting membrane potential is quantified as approximately -65 millivolts (mV), indicating that the interior of the neuron is negatively charged relative to the outside.
- Ionic Distribution and Its Role
- The resting membrane potential is primarily determined by the distribution of ions across the neuronal membrane, particularly sodium (Na⁺), potassium (K⁺), chloride (Cl⁻), and organic anions (A⁻).
- Inside the neuron, K⁺ ions are found in higher concentrations, while Na⁺ ions are more prevalent outside the neuron. This difference in ion concentration creates a concentration gradient, contributing to the negative charge inside the cell.
- The resting membrane potential is primarily determined by the distribution of ions across the neuronal membrane, particularly sodium (Na⁺), potassium (K⁺), chloride (Cl⁻), and organic anions (A⁻).
- Functions of Ion Channels and Pumps
- Specific ion channels and pumps regulate the movement of these ions across the membrane, thus maintaining the resting potential.
- The sodium-potassium pump (Na⁺/K⁺ ATPase) actively transports three Na⁺ ions out of the neuron and two K⁺ ions into the neuron for each cycle, consuming ATP in the process. This action is crucial for sustaining the concentration gradients of these ions.
- Potassium channels allow K⁺ ions to move out of the neuron, while sodium channels limit Na⁺ influx, which helps maintain the negative interior of the cell.
- Specific ion channels and pumps regulate the movement of these ions across the membrane, thus maintaining the resting potential.
- Electrochemical Gradients
- The resting membrane potential results from the interplay between chemical gradients (due to ion concentrations) and electrical gradients (due to the charge of the ions).
- As K⁺ ions exit the neuron through leak channels, the interior becomes more negative. Conversely, the presence of negatively charged organic anions, which cannot cross the membrane, further contributes to the negative charge.
- The resting membrane potential results from the interplay between chemical gradients (due to ion concentrations) and electrical gradients (due to the charge of the ions).
- Significance of the Resting Membrane Potential
- The maintenance of a negative resting membrane potential is essential for neuronal excitability and the generation of action potentials.
- When a neuron receives sufficient stimulation, the membrane potential can change, leading to depolarization and the initiation of an action potential.
- Thus, the resting membrane potential serves as the baseline from which neuronal signaling begins, highlighting its crucial role in the overall functioning of the nervous system.
- The maintenance of a negative resting membrane potential is essential for neuronal excitability and the generation of action potentials.
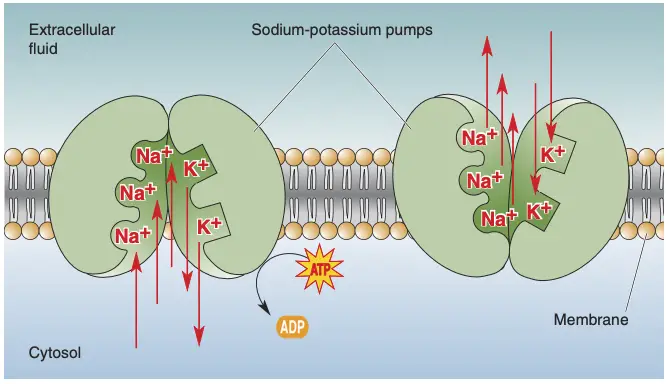
Equilibrium Potentials
Equilibrium potentials are crucial in understanding how ions contribute to the electrical properties of cells, particularly in neurons. These potentials represent the voltage at which the net movement of a specific ion across the membrane ceases because the electrical forces balance the concentration gradients.
- Definition of Equilibrium Potential
- The equilibrium potential (E_ion) for an ion is the membrane voltage at which the chemical and electrical driving forces acting on the ion are equal and opposite, resulting in no net movement of that ion across the membrane.
- Each ion has a unique equilibrium potential based on its concentration gradient and charge.
- The equilibrium potential (E_ion) for an ion is the membrane voltage at which the chemical and electrical driving forces acting on the ion are equal and opposite, resulting in no net movement of that ion across the membrane.
- Mechanism of Establishing Equilibrium Potentials
- To illustrate, consider a scenario where a cell contains a high concentration of potassium (K⁺) ions inside, with a lower concentration outside.
- When potassium channels are introduced into the membrane, K⁺ ions diffuse out of the cell due to the concentration gradient. This movement leaves behind negatively charged anions (A⁻), causing the inside of the cell to become negatively charged.
- To illustrate, consider a scenario where a cell contains a high concentration of potassium (K⁺) ions inside, with a lower concentration outside.
- Significant Factors Influencing Equilibrium Potentials
- Ionic Concentration Gradients: The difference in concentration of the ion across the membrane drives its movement.
- Even small changes in ion concentration can lead to substantial alterations in membrane potential. For example, a minor shift in K⁺ concentration can result in a significant change in Vm.
- Electrical Forces: As K⁺ ions exit the cell, the growing negative charge inside creates an opposing electrical force that eventually counterbalances the force of diffusion.
- At equilibrium, the influx of K⁺ due to electrical attraction is equal to its efflux due to diffusion, leading to a stable equilibrium potential, which can be calculated for K⁺.
- Ionic Concentration Gradients: The difference in concentration of the ion across the membrane drives its movement.
- Calculation of Equilibrium Potentials
- The equilibrium potential can be calculated using the Nernst equation, which takes into account the ion’s concentration on both sides of the membrane and its charge.
- For instance, if K⁺ is more concentrated inside the cell, the equilibrium potential (E_K) would be negative, typically around -80 mV, signifying that electrical forces would pull K⁺ back into the cell when Vm approaches this value.
- The equilibrium potential can be calculated using the Nernst equation, which takes into account the ion’s concentration on both sides of the membrane and its charge.
- Individual Ion Contributions
- Each ion contributes uniquely to the overall membrane potential, and its equilibrium potential can be determined independently.
- For example, if sodium (Na⁺) channels are open, Na⁺ would flow into the cell due to its concentration gradient, leading to a positive equilibrium potential (E_Na) if the cell’s interior becomes positively charged.
- Each ion contributes uniquely to the overall membrane potential, and its equilibrium potential can be determined independently.
- Implications for Neuronal Function
- Understanding equilibrium potentials is essential for grasping how neurons generate action potentials and maintain resting membrane potentials.
- The ionic driving force, which is the difference between the actual membrane potential (V_m) and the equilibrium potential (E_ion), dictates the direction and magnitude of ionic currents, influencing neuronal excitability and signaling.
- Understanding equilibrium potentials is essential for grasping how neurons generate action potentials and maintain resting membrane potentials.
The Distribution of Ions Across the Membrane
The distribution of ions across the neuronal membrane is fundamental for establishing the membrane potential, which is critical for neuronal function. The differential concentrations of specific ions inside and outside the neuron are maintained primarily by ion pumps, which actively transport ions against their concentration gradients.
- Key Ion Concentrations
- The principal ions involved in neuronal activity include potassium (K⁺), sodium (Na⁺), calcium (Ca²⁺), and chloride (Cl⁻).
- Potassium (K⁺): Concentrated about 20 times more inside the neuron (100 mM) than outside (5 mM), resulting in an equilibrium potential (E_K) of approximately -80 mV.
- Sodium (Na⁺): More prevalent outside (150 mM) than inside (15 mM), leading to an equilibrium potential (E_Na) of about +62 mV.
- Calcium (Ca²⁺): Extremely low inside (0.0002 mM) compared to outside (2 mM), with a high equilibrium potential (E_Ca) of around +123 mV.
- Chloride (Cl⁻): Exists at a concentration of 150 mM outside versus 13 mM inside, corresponding to an equilibrium potential (E_Cl) of approximately -65 mV.
- The principal ions involved in neuronal activity include potassium (K⁺), sodium (Na⁺), calcium (Ca²⁺), and chloride (Cl⁻).
- Establishment of Ionic Concentration Gradients
- Ionic gradients are primarily established through the action of specific ion pumps, notably the sodium-potassium pump and the calcium pump.
- Sodium-Potassium Pump: This enzyme utilizes ATP to exchange three sodium ions (Na⁺) from inside the cell for two potassium ions (K⁺) from outside. This active transport mechanism is essential for maintaining the high intracellular K⁺ concentration and the low intracellular Na⁺ concentration.
- The pump’s activity consumes about 70% of the total ATP used by the brain, illustrating its critical role in neuronal energetics.
- Calcium Pump: This pump actively transports calcium ions (Ca²⁺) out of the cell, maintaining a low intracellular concentration. Other mechanisms, such as calcium-binding proteins and organelles like mitochondria, further assist in sequestering Ca²⁺ to prevent excessive accumulation in the cytosol.
- Sodium-Potassium Pump: This enzyme utilizes ATP to exchange three sodium ions (Na⁺) from inside the cell for two potassium ions (K⁺) from outside. This active transport mechanism is essential for maintaining the high intracellular K⁺ concentration and the low intracellular Na⁺ concentration.
- Ionic gradients are primarily established through the action of specific ion pumps, notably the sodium-potassium pump and the calcium pump.
- Role of Ion Pumps in Neuronal Function
- Ion pumps are essential for the maintenance of the resting membrane potential, which is crucial for the excitability of neurons.
- Without these pumps, the concentration gradients necessary for generating action potentials would collapse, leading to a loss of neuronal function.
- Therefore, ion pumps are integral to the background processes that sustain neuronal activity, even if they do not receive as much attention as ion channels.
- Ion pumps are essential for the maintenance of the resting membrane potential, which is crucial for the excitability of neurons.
Relative Ion Permeabilities of the Membrane at Rest
The relative ion permeabilities of the neuronal membrane at rest are essential for establishing the resting membrane potential, a critical factor in neuronal excitability and function. While the concentration gradients of ions provide a foundation, the permeability of the membrane to these ions ultimately determines the actual membrane potential.
- Ion Concentration and Membrane Potential
- The membrane potential is influenced by the relative concentrations of key ions, primarily potassium (K⁺) and sodium (Na⁺).
- If the membrane were exclusively permeable to K⁺, the potential would stabilize at approximately -80 mV (E_K).
- If the membrane were solely permeable to Na⁺, the potential would reach around +62 mV (E_Na).
- In reality, the membrane is permeable to both ions, resulting in a membrane potential that is an average influenced by both E_K and E_Na.
- The membrane potential is influenced by the relative concentrations of key ions, primarily potassium (K⁺) and sodium (Na⁺).
- Role of the Goldman Equation
- The Goldman equation enables the calculation of the resting membrane potential by accounting for the relative permeabilities of the membrane to different ions.
- Assuming a permeability ratio where K⁺ permeability is about 40 times greater than that of Na⁺, the Goldman equation predicts a resting potential of approximately -65 mV, aligning with observed values.
- This equation highlights how a small change in permeability can significantly influence the overall membrane potential.
- The Goldman equation enables the calculation of the resting membrane potential by accounting for the relative permeabilities of the membrane to different ions.
- Potassium Channels and Their Function
- Potassium channels play a pivotal role in maintaining resting membrane potential and are characterized by their selectivity and diversity.
- Most potassium channels consist of four subunits arranged to form a central pore, allowing selective permeability primarily to K⁺.
- The unique arrangement of amino acids within the channel, particularly in regions called the pore loops, creates a selectivity filter that allows K⁺ to pass while excluding other ions.
- Mutations in these channels can lead to significant neurological disorders, underscoring their importance in cellular function.
- Potassium channels play a pivotal role in maintaining resting membrane potential and are characterized by their selectivity and diversity.
- Influence of External Potassium Concentration
- The neuronal membrane is particularly sensitive to changes in the concentration of extracellular potassium ([K⁺]ₒ).
- An increase in [K⁺]ₒ can lead to depolarization, shifting the membrane potential from -65 mV to values closer to zero.
- This sensitivity necessitates regulatory mechanisms to maintain stable extracellular potassium levels, such as the blood-brain barrier and astrocytic buffering.
- The neuronal membrane is particularly sensitive to changes in the concentration of extracellular potassium ([K⁺]ₒ).
- Astrocytes and Potassium Regulation
- Astrocytes play a crucial role in buffering extracellular potassium. They contain potassium pumps and channels that allow them to take up excess K⁺ during periods of neuronal activity.
- This process, known as potassium spatial buffering, helps dissipate elevated potassium concentrations across the extensive network of astrocytic processes, thus protecting neuronal function.
- Unlike neurons, muscle cells lack similar buffering mechanisms, making them more vulnerable to fluctuations in extracellular potassium levels.
- Astrocytes play a crucial role in buffering extracellular potassium. They contain potassium pumps and channels that allow them to take up excess K⁺ during periods of neuronal activity.