Multiphoton microscopy is a cutting-edge imaging technique widely used in biology and medicine to study living tissues with minimal harm. Instead of relying on single high-energy photons like conventional microscopes, this method harnesses multiple low-energy photons that act together to produce a fluorescent signal. The trick lies in timing—when these photons strike a molecule simultaneously, their combined energy activates fluorescent markers, lighting up specific structures. This approach allows researchers to peer deeper into tissues than traditional methods, as the lower-energy light scatters less and causes fewer disruptions to cells. The result is sharper images with less background interference, perfect for observing delicate processes in real time. It’s especially handy for tracking brain activity, watching cells interact in developing organisms, or exploring thick tissue layers without slicing them apart. By prioritizing precision and gentleness, multiphoton microscopy has become a favorite for studying life’s intricate details while keeping samples intact and healthy.
What is Multiphoton Microscopy?
- Based on the simultaneous absorption of two or more lower‑energy photons to activate fluorophores inside a tiny sample, multiphoton microscopy is a nonlinear fluorescence imaging method.
- The excitation mechanism limits fluorescence emission to the focus volume and uses femtosecond pulsed infrared lasers to attain the high photon flux required for multiphoton absorption, hence enabling intrinsic optical sectioning.
- Maria Goeppert‑Mayer created the theoretical foundation of multiphoton excitation in 1931 by means of her PhD dissertation on two‑photon quantum transitions.
- Two-photon absorption in CaF₂:Eu²⁺ crystals was proven by Wolfgang Kaiser in 1961 and in cesium vapor by Isaac Abella in 1962, therefore verifying the viability of multiphoton techniques.
- At Cornell University in 1990 Winfried Denk, James Strickler, and Watt W. Webb invented and patented the first useful use of two-photon laser scanning fluorescence microscopy.
- Using near-infrared excitation light—which is dispersed less in biological tissues—multiphoton microscopy provides deeper tissue penetration of up to almost one millimeter.
- Using longer wavelengths and the quadratic dependency of excitation on photon flux helps the method reduce photobleaching and photodamage outside the focus area.
- High signal-to-background ratios and enhanced picture contrast follow from intrinsic three-dimensional optical sectioning accomplished without the requirement for confocal pinholes.
- Among the limitations include the high cost and complexity of femtosecond pulsed laser systems as well as perhaps reduced lateral resolution in thin materials as compared to single-photon confocal imaging.
- In many spheres of life science study, the method has grown indispensible.
- In vivo imaging of neuronal structure and activity in live animals, neuroscience
- Research on cancer, classification of skin and breast cancer tissues, investigation of tumor cell interactions
- Long-term live imaging of embryos and tissue morphogenesis in developmental biology and embryology
- Materials science and tissue engineering; three-dimensional imaging of scaffolds and biomaterials
- Variants include three-photon excitation microscopy provide novel uses in deep-brain imaging and photopharmacology and stretch imaging depth farther into scattering tissues.
Principle of Multiphoton Microscopy
- Based on nonlinear fluorescence excitation, multiphoton microscopy absorbs two or more infrared photons almost simultaneously by a fluorophore to attain an excited state similar to that generated by a single higher‑energy photon.
- The absorption probability grows with the nth power of instantaneous light intensity (Iⁿ for n‑photon excitation), thereby requiring high peak intensities attained only with femtosecond pulsed lasers.
- While keeping low average power to reduce sample heating, femtosecond Ti:Sapphire lasers, tuned at 700–1000 nm with pulse widths of ~100% and repetition rates ~80% offer the high photon flux needed for effective multiphoton absorption.
- Nonlinear excitation limits fluorescence production to the focused volume (sub‑femtoliter), thereby producing intrinsic optical sectioning without a confocal pinhole and so lowering out‑of‑focus background.
- Minimizing photobleaching and photodamage in areas beyond the imaging plane, the confined excitation volume outside the focus plane gets inadequate photon flux for multiphoton absorption.
- Infrared excitation wavelengths are inside the biological optical windows (700–1300 nm), where tissue absorption and scattering are reduced, therefore allowing imaging depths up to ~1 mm in scattering material.
- Usually acquired using nondescanned detectors near the objective, emitted fluorescence is readily captured and enhances signal from deep within tissues by means of scattered photons.
- The two‑photon absorption rate W can be expressed as W = δ × I², where δ is the two‑photon cross section in Göeppert‑Mayer units (1 GM = 10⁻⁵⁰ cm⁴ s photon⁻¹) and I is the photon flux
- Multiphoton processes also yield coherent signals like second harmonic generation, in which two photons combine to make a single photon at half the wavelength, therefore allowing label-free imaging of highly organized structures like collagen.
- Higher‑order excitation variants, such as three‑photon microscopy (∝ I³), use longer wavelengths (~1300–1700 nm) to further extend imaging depth and reduce background in highly scattering tissues
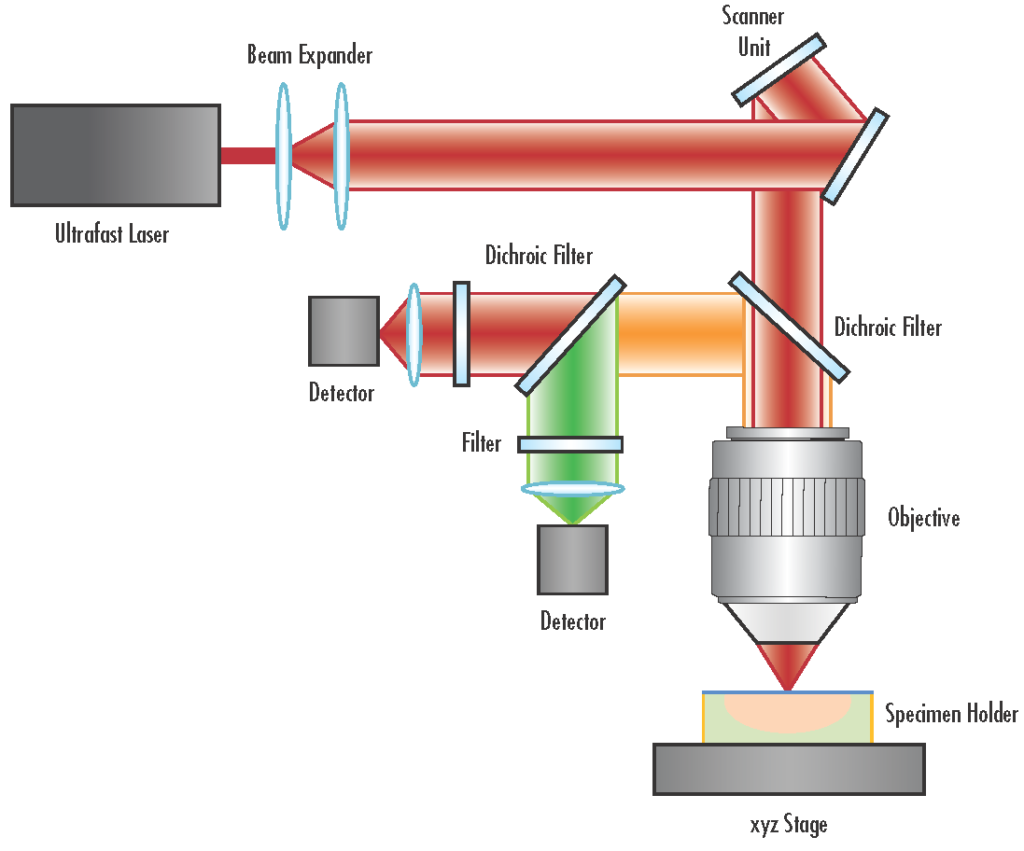
Instrumentation of Multiphoton Microscope
A multiphoton microscope integrates several specialized components to facilitate nonlinear optical imaging, enabling high-resolution, three-dimensional visualization of specimens. The primary components include:
- Ultrafast Pulsed Laser Source: Typically, a femtosecond Ti:Sapphire laser provides the high peak power necessary for multiphoton excitation. These lasers emit pulses in the near-infrared spectrum, which penetrate deeper into biological tissues with reduced scattering and absorption.
- Beam Steering and Scanning System: Comprising galvanometric mirrors or resonant scanners, this system directs the laser beam across the sample in a raster pattern, enabling point-by-point image acquisition.
- Beam Expansion and Relay Optics: A series of lenses expand and relay the laser beam to fill the back aperture of the objective lens, ensuring optimal focusing and maximizing the excitation efficiency at the focal point.
- Objective Lens: High numerical aperture (NA) objectives focus the laser beam into the specimen. Objectives designed for multiphoton microscopy often have long working distances and are corrected for chromatic aberrations over a broad wavelength range to accommodate the near-infrared excitation light.
- Non-descanned Detectors (NDDs): Positioned close to the objective lens, NDDs collect fluorescence signals emitted from the focal volume. These detectors are typically photomultiplier tubes (PMTs) optimized for low-light detection and are essential for capturing scattered photons from deep within the specimen.
- Emission Filters and Dichroic Mirrors: These optical elements selectively transmit fluorescence emissions while blocking excitation light, ensuring that only the desired signal reaches the detectors.
- Control and Data Acquisition System: Integrated software interfaces with the hardware components to control scanning parameters, synchronize data collection, and reconstruct images for analysis.
Sample Preparation Steps in Multiphoton Microscope
- High-quality multiphoton imaging depends on proper sample preparation as it directly influences the imaging depth and signal-to-noise ratio.
- The necessary actions differ depending on the kind of the material; tissue slices and cultured cells call for different procedures.
- For tissue sections
- Fixation at pH 7.2–7.4 using 4% paraformaldehyde in phosphate-buffered saline (PBS) reduces degradation and retains cellular architecture.
- Thoroughly rinsing the tissue in PBS eliminates any remaining fixative to avoid interference with subsequent processes.
- Usually 50–100 µm thick, sectioning the preserved tissue with a vibratome or microtome into slices guarantees enough penetration of excitation light.
- If immunostasking is intended, permeabilization with a detergent solution—e.g., 0.1% Triton X-100 in PBS—helps antibodies or fluorescent probes enter more easily.
- Reducing non-specific binding during subsequent labeling by blocking with a suitable serum solution—such as 5% normal serum in PBS
- Using fluorescent dyes or primary and secondary antibodies targets certain biological components, therefore assuring compatibility with wavelengths of multiphoton stimulation.
- To improve imaging depth, optional optical clearing with certain reagents can help to minimize scattering in thick tissues.
- Stabilizing the sample for imaging requires mounting the tissue slices onto microscope slides using an appropriate mounting media (like one including anti-fade reagents).
- For cultured cells
- Using high optical quality imaging dishes or coverslips gives a flat, easily accessible substrate for imaging from seed cells.
- Fixation with 4% paraformaldehyde maintains intracellular structures and cell shape.
- Agents like 0.1% Triton X-100 provide permeabilization that lets fluorescent markers pass the cell membrane when needed.
- Under multiphoton stimulation, staining with fluorescent dyes or antibodies specific to the target molecules helps visualize.
- Covering slides with the suitable mounting media reduces photobleaching and preserves fluorescence during imaging.
- Minimizing photodamage, lowering background fluorescence, and obtaining the optimum imaging performance in multiphoton microscopy depend on optimizing these techniques depending on the kind of material and experimental objectives.
Operating Procedure of Multiphoton Microscope
- Sign in the instrument log book under your name, date, time, imaging goal.
- Check the cooling water circulator’s stability at 25°C and that flow is continuous to preserve laser performance.
- Verify all beam lines are contained and interlock by don laser safety glasses rated OD > 700 nm.
- Turn the laser key from standby to on and let at least ten minutes warm-up to attain stable mode-lock conditions.
- Match the excitation peak of your fluorophore by adjusting the laser wavelength using the front-panel dial; then, note the chosen wavelength.
- Power on microscope electronics progressively (e.g., power supply #1–#7), waiting several seconds between each to guarantee correct startup.
- Starting the imaging program and configuring device parameters
- set Pockels cell controller to zero and PMT high voltage
- Disabling mechanical origin search will enable resonant scanning and XY stage control.
- Set the sample on the stage and find the area of interest using either bright field or epifluorescence.
- Turn down the room lights, put the emission cube to position 1, then pull out the light route selection to activate the multiphoton beam path.
- To turn on the interlock, close the front sliding panel; open the laser shutter.
- Choose live scan mode, then progressively raise PMT voltage and Pockels cell (AOTF) amplitude until the image window shows fluorescence.
- For best signal-to– noise, maximize imaging settings including laser power at sample, PMT gain, scan speed, pixel dwell times.
- For repeatability, log all imaging parameters including laser wavelength, power, PMT voltages, Pockels cell voltage, scan settings.
- Position the emission filter cube between two and six and push in the light path selection to disable the multiphoton route.
- Set Pockels cell controller to zero and PMT high voltage to zero.
- Close the laser shutter, leave the imaging program, then turn the laser key back to standby.
- As with the front system, repeat the first log book input, cold water check, laser key activation and wavelength adjustment.
- Turn on the gear and computer’s power; then, start the multiphoton imaging program.
- Set PMT high voltage off and initial laser intensity to 0.7 using software control.
- Mount the sample, switch off room lights, put the filter cube to position 1, then draw out the light path selection.
- Through the hardware controller, open the laser shutter and check PMT readings to be zero before raising voltage.
- Set continuous scan mode and raise PMT high voltage to 70–80% until the image window shows a non-zero signal.
- From 0.7 to the required level, progressively increase laser intensity; then, fine-tune PMT gain, laser power, scan rate and acquisition parameters.
- Set PMT high voltage off and use software to zero laser intensity.
- Position the filter cube between 2 and 6 then press in the light path selection.
- Close the imaging program, close the laser shutter, then turn the laser key to standby.
- Use fluorescent alignment slides to assess beam alignment following significant wavelength changes to guarantee best excitation path alignment.
- Using lens tissue and distilled water, clean objective lenses before every session to avoid optical distortions.
- Daily stage leveling and vibration isolation help to keep imaging stability.
- Monthly calibration of the Pockels cell or AOTF guarantees precise control of laser intensity.
- Keep a maintenance journal including software updates, laser servicing intervals, and water circulator maintenance.
Applications of Multiphoton Microscopy
- Using fluorescent markers, in vivo imaging of neuronal structure and function in live brain tissue and entire animals allows mapping of neural networks and monitoring synaptic dynamics with submicron resolution and imaging depths up to 1 mm.
- Using endogenous fluorescence, fluorescent labeling and second harmonic generation, noninvasive viewing and characterisation of tumor tissues in skin, breast, prostate and bladder malignancies evaluates tumor margins and microenvironment in real time.
- Using shallow tissue penetration and high contrast of multiphoton excitation, real time monitoring of skin physiology, aging, regeneration, transdermal transport of medications, cosmetics, and nanoparticles is achieved.
- Developmental processes and organogenesis may be studied using long term live imaging of embryos, organoids and tissue morphogenesis with low phototoxicity and photobleaching.
- Adaptive and innate immune responses at single cell resolution are clarified by real-time observation of immune cell movement, interaction and activation inside lymph nodes and inflamed tissues.
- Evaluation of bladder and prostate cancer tissues and assessment of spermatogenesis phases in seminiferous tubules help to direct therapeutic decisions and enhance surgical results.
- To evaluate tissue constructions and guide regeneration treatments, three dimensional imaging of scaffolds, extracellular matrix components and biomaterials incorporating label free second harmonic generating viewing of collagen and bone structures.
- High resolution intravital imaging of blood arteries, cardiac myocytes and hemodynamics in healthy and sick tissues to investigate vascular remodeling and cardiac physiology in vivo.
- Second harmonic generation and coherent anti-Stokes Raman scattering offer contrast for nonfluorescent materials like collagen, myosin, and lipids without exogenous labeling.
- Real-time tracking of medicinal drug, nanoparticle, and cosmetic penetration and dispersion throughout tissues helps to maximize delivery methods and assess effectiveness.
Advantages of Multiphoton Microscopy
- NIR excitation at 700–1300 nm reduces scattering proportional to λ⁻⁴, enabling imaging depths up to ~1 mm in biological tissues
- Nonlinear excitation eliminates the requirement for confocal pinholes by confining fluorescence to the focus volume (sub‑femtoliter), hence giving intrinsic optical sectioning.
- Localised multiphoton absorption reduces photobleaching and photodamage beyond the focus plane, hence extending sample viability during extended imaging.
- Second and third harmonic generation as well as autofluorescence produced by multiphoton methods help to see endogenous structures free from exogenous dyes.
- Reduced phototoxicity and heating caused by lower absorption of NIR light by biological chromophores makes multiphoton microscopy ideal for live-cell and in vivo imaging.
- Multiple fluorophoresite excitation under a single tuned femtosecond laser simplifies multicolour imaging and lowers alignment complexity.
- In deep tissue imaging, high signal to- background ratios in scattering samples enhance contrast and quantitative accuracy.
- Three-dimensional precise reconstruction of cellular and subcellular structures is made possible by submicron lateral and axial resolution.
- Long-term investigations of dynamic biological events like neuronal activity, embryogenesis, and tumour growth are made possible by compatibility with live imaging.
- Three-photon microscopy and other advanced modalities enhance imaging depth and contrast, therefore creating new opportunities for deep-brain and organ imaging.
Limitations of Multiphoton Microscopy
- Often limited to a single tuned Ti:Sapphire laser, the cost and complexity of femtosecond laser systems necessitate major capital investment and specialised maintenance and limit multiphoton configurations.
- Common Ti:Sapphire lasers restrict effective excitation of several red-shifted fluorophores without additional optical parametric oscillator modules to around 680–1080 nm.
- Poor two-photon absorption Typical fluorophores require high peak intensities to provide adequate signal, therefore lowering fluorescence brightness relative to one-photon excitation and complicating probe selection. Cross-sections (∼1–100 GM) also require high peak intensities.
- Unpredictable two-photon excitation spectra—often showing widening, red-shifting and unexpected peaks compared to single-photon spectra—demand empirical characterisation for every dye and complicate multicolour studies.
- Emitted fluorescence stays in the visible spectrum and is vulnerable to scattering and absorption, therefore restricting practical imaging depths to ~1 mm and reducing signal-to-‑noise at deeper depths.
- High local photon concentrations at the focus plane can cause photodamage and photobleaching during extended or high-speed imaging, therefore influencing live-sample viability.
- Scanner inertia and pixel dwell lengths limit point-scanning acquisition speeds, therefore impeding capture of fast dynamic processes and big volumetric datasets.
- Because of longer excitation wavelengths, spatial resolution (~0.29 µm lateral, ~0.97 µm axial with NA 1.4 at 900 nm) is intrinsically poorer than confocal microscopy (~0.16 µm lateral, ~0.52 µm axial at 488 nm).
- Demand specialised optics and consistent calibration to prevent pulse widening; maintenance of ultrashort pulse durations at the sample demands dispersion correction and exact beam alignment.
- Ti:Sapphirebased multiphoton systems demand specialised optical tables, vibration isolation and environmental control that restrict mobility for clinical or field deployment as they are large and challenging to move.
- Dzhagalov IL, Melichar HJ, Ross JO, Herzmark P, Robey EA. Two-photon imaging of the immune system. Curr Protoc Cytom. 2012 Apr;Chapter 12:Unit12.26. doi: 10.1002/0471142956.cy1226s60. PMID: 22470153; PMCID: PMC3662370.
- Katz MJ, Huland DM, Ramasamy R. Multiphoton microscopy: applications in Urology and Andrology. Transl Androl Urol. 2014 Mar 1;3(1):77-83. doi: 10.3978/j.issn.2223-4683.2014.01.01. PMID: 25741460; PMCID: PMC4345420.
- Yew E, Rowlands C, So PT. Application of Multiphoton Microscopy in Dermatological Studies: a Mini-Review. J Innov Opt Health Sci. 2014 Jan 3;7(5):1330010. doi: 10.1142/S1793545813300103. PMID: 25075226; PMCID: PMC4112132.
- Luu, P., Fraser, S.E. & Schneider, F. More than double the fun with two-photon excitation microscopy. Commun Biol 7, 364 (2024). https://doi.org/10.1038/s42003-024-06057-0
- Tauer U. Advantages and risks of multiphoton microscopy in physiology. Exp Physiol. 2002 Nov;87(6):709-14. doi: 10.1113/eph8702464. PMID: 12530403.
- Luu, P., Fraser, S.E. & Schneider, F. More than double the fun with two-photon excitation microscopy. Commun Biol 7, 364 (2024). https://doi.org/10.1038/s42003-024-06057-0
- Larson, A. Multiphoton microscopy. Nature Photon 5, 1 (2011). https://doi.org/10.1038/nphoton.an.2010.2
- https://physoc.onlinelibrary.wiley.com/doi/pdf/10.1113/eph8702464
- https://bpb-us-e2.wpmucdn.com/faculty.sites.uci.edu/dist/3/352/files/2011/03/J171_MPMLiu.pdf
- https://w3.biosci.utexas.edu/pmc/webdocs/Multiphoton.pdf
- https://www.microscopyu.com/techniques/multi-photon/multiphoton-microscopy
- https://www.sciencedirect.com/science/article/abs/pii/S0092867424008304
- https://www.sciencedirect.com/science/article/abs/pii/S0168010221002455
- https://www.mdpi.com/1422-0067/22/5/2657
- https://www.frontiersin.org/journals/neuroscience/articles/10.3389/fnins.2024.1360482/
- https://www.frontiersin.org/journals/immunology/articles/10.3389/fimmu.2020.00039/