What is Energy rich compound?
- An energy-rich compound, also known as a high-energy compound, is a molecule or substance that possesses a large amount of chemical potential energy, which can be released and utilized to perform work or provide energy for various biological, chemical, or physical processes. These compounds typically contain high-energy bonds that store energy in their molecular structures.
- One of the most common examples of an energy-rich compound is adenosine triphosphate (ATP), which serves as the primary energy currency in living organisms. ATP is composed of an adenosine molecule bonded to three phosphate groups. The high-energy bonds between the phosphate groups in ATP store energy that can be readily released and used by cells to drive metabolic reactions, muscle contractions, active transport processes, and other energy-requiring activities.
- Other examples of energy-rich compounds include various phosphorylated molecules like guanosine triphosphate (GTP) and creatine phosphate. Additionally, molecules like glucose and fatty acids are considered energy-rich because they can be broken down through metabolic processes to release energy in the form of ATP.
- In summary, energy-rich compounds are molecules that contain high-energy bonds and serve as a source of stored energy, which can be harnessed and utilized for various biological, chemical, and physical functions.
Phosphoenolpyruvate
- Phosphoenolpyruvate (PEP) is a high-energy compound and an important intermediate in both glycolysis and gluconeogenesis, which are metabolic pathways involved in the production and utilization of energy in cells.
- PEP is derived from the glycolytic intermediate, 2-phosphoglycerate, through the action of the enzyme enolase. It is then converted to pyruvate by the enzyme pyruvate kinase in the final step of glycolysis, resulting in the production of ATP.
- What makes PEP an energy-rich compound is the presence of a high-energy phosphate bond between the phosphate group and the carbon atom of the pyruvate moiety. This bond contains a large amount of potential energy that can be released and used for various cellular processes.
- In addition to its role in glycolysis, PEP also plays a crucial role in gluconeogenesis, which is the reverse pathway of glycolysis involved in the synthesis of glucose from non-carbohydrate precursors. During gluconeogenesis, PEP is generated from oxaloacetate, and it serves as a precursor for glucose synthesis.
- PEP is involved in various biochemical reactions beyond energy production. It is a key molecule in the biosynthesis of amino acids, such as phenylalanine, tyrosine, and tryptophan. PEP is also utilized in the synthesis of other important compounds, including certain neurotransmitters, nucleotides, and aromatic compounds.
- Overall, phosphoenolpyruvate is an energy-rich compound that serves as an intermediate in both glycolysis and gluconeogenesis, playing a vital role in energy metabolism and various biosynthetic pathways in living organisms.
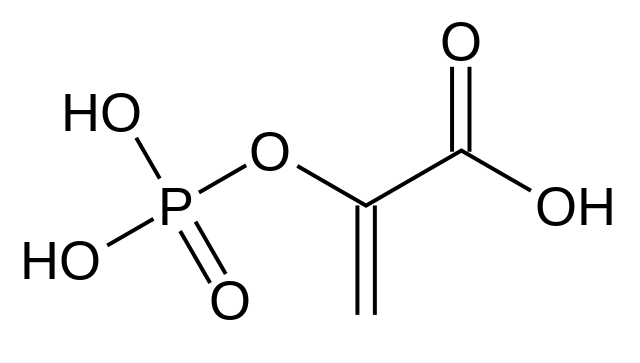
Structure of Phosphoenolpyruvate
The structure of phosphoenolpyruvate (PEP) consists of a three-carbon pyruvate molecule with an additional phosphate group attached to one of the carbon atoms. Here is the structural formula of PEP:
HO-C=O | P-O-P-OH | CH2-CHO
In the structure, the central carbon atom is double-bonded to an oxygen atom, forming a carbonyl group (C=O). The other two bonds of the central carbon atom are attached to an oxygen atom and a phosphate group (P-O-P-OH). The phosphate group consists of a phosphorus atom (P) bonded to three oxygen atoms (two of which are part of the phosphate group itself). Finally, the carbon atom adjacent to the phosphate group is attached to a hydrogen atom (H) and a carbonyl group (C=O).
The phosphate group and the double bond between the central carbon and oxygen atoms are responsible for the high-energy characteristics of phosphoenolpyruvate, enabling it to participate in energy-requiring reactions and serve as a crucial intermediate in metabolic pathways.
Functions of Phosphoenolpyruvate
Phosphoenolpyruvate (PEP) has several important functions in cellular metabolism. Here are some of its key roles:
- Energy Transfer: PEP serves as a high-energy compound in energy transfer reactions. It acts as a phosphate donor in the process of substrate-level phosphorylation, where it transfers its phosphate group to adenosine diphosphate (ADP) to produce adenosine triphosphate (ATP). This ATP can then be used as a source of energy for various cellular processes.
- Glycolysis: PEP is an intermediate in glycolysis, the metabolic pathway that breaks down glucose to produce energy. In the final step of glycolysis, PEP is converted to pyruvate by the enzyme pyruvate kinase, resulting in the production of ATP. This step also generates a high-energy phosphate bond in PEP, which drives the energy production.
- Gluconeogenesis: PEP plays a crucial role in gluconeogenesis, which is the reverse pathway of glycolysis. In gluconeogenesis, PEP is generated from oxaloacetate and serves as a precursor for glucose synthesis. Through a series of enzymatic reactions, PEP is converted to glucose, allowing the production of glucose from non-carbohydrate sources.
- Biosynthesis: PEP is involved in the biosynthesis of various compounds in the cell. It serves as a precursor for the synthesis of aromatic amino acids like phenylalanine, tyrosine, and tryptophan. PEP is also used in the production of other important molecules, including certain neurotransmitters, nucleotides, and aromatic compounds.
- Carbon Dioxide Fixation: PEP is utilized in certain metabolic pathways for carbon dioxide fixation. In plants, the enzyme phosphoenolpyruvate carboxylase incorporates carbon dioxide into PEP, forming oxaloacetate in a process known as C4 photosynthesis. This mechanism helps plants efficiently capture carbon dioxide and minimize water loss.
Overall, phosphoenolpyruvate plays a critical role in energy metabolism, acting as a high-energy compound and an intermediate in both glycolysis and gluconeogenesis. It also contributes to the biosynthesis of various important molecules, highlighting its significance in cellular metabolism.
1,3- Bisphosphoglycerate
1,3-Bisphosphoglycerate (1,3-BPG) is a phosphorylated intermediate in the glycolytic pathway. It is formed during the conversion of glyceraldehyde 3-phosphate to 1,3-BPG by the enzyme glyceraldehyde 3-phosphate dehydrogenase. 1,3-BPG subsequently undergoes further enzymatic reactions to produce ATP and pyruvate, leading to the generation of energy.
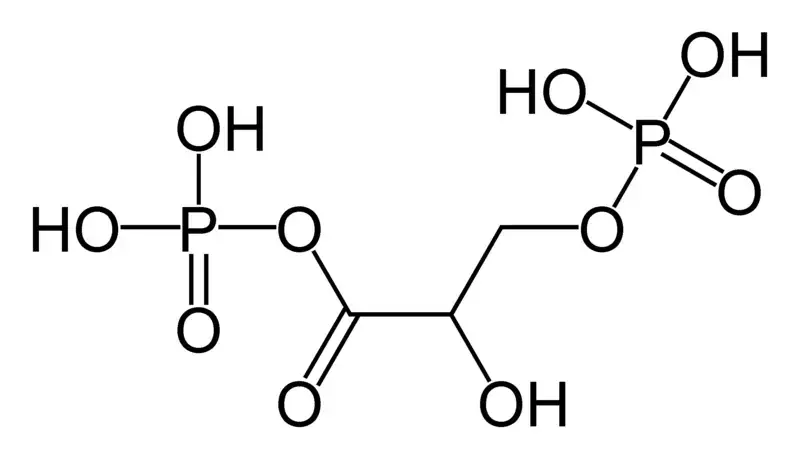
Here is the structural formula of 1,3-Bisphosphoglycerate:
HO-C(=O)-CH2-O-PO3H2
|
O
|
P-O-P-OH
In the structure, the central carbon atom is part of a glycerate backbone, with a carboxyl group (C=O) and a hydroxyl group (OH) attached to it. Two phosphate groups (PO3H2) are connected to the carbon atom through oxygen atoms. The phosphate groups in 1,3-BPG contain high-energy bonds that store potential energy.
The primary function of 1,3-BPG is to transfer a high-energy phosphate group to adenosine diphosphate (ADP) to produce adenosine triphosphate (ATP) through the process of substrate-level phosphorylation. This occurs in the later stages of glycolysis. The enzyme phosphoglycerate kinase catalyzes the transfer of the phosphate group from 1,3-BPG to ADP, resulting in the production of ATP and the conversion of 1,3-BPG to 3-phosphoglycerate.
1,3-BPG also has an important role in regulating oxygen affinity in red blood cells. It binds to hemoglobin, reducing its affinity for oxygen and promoting the release of oxygen to tissues in need.
In summary, 1,3-Bisphosphoglycerate is an intermediate molecule in glycolysis that carries high-energy phosphate groups. Its main function is to transfer a phosphate group to ADP, leading to ATP production. Additionally, it plays a role in oxygen transport and regulation in red blood cells.
Functions of 1,3- Bisphosphoglycerate
To clarify, the primary function of 1,3-BPG is its involvement in energy production during glycolysis. Here are the correct functions of 1,3-Bisphosphoglycerate:
- Energy Generation: 1,3-BPG is an intermediate molecule in the glycolytic pathway, which is a central metabolic pathway involved in the breakdown of glucose to produce energy. It is formed during the conversion of glyceraldehyde 3-phosphate to 1,3-BPG by the enzyme glyceraldehyde 3-phosphate dehydrogenase. The formation of 1,3-BPG is coupled with the production of NADH, a reduced coenzyme that carries electrons to the electron transport chain for further ATP production.
- ATP Production: 1,3-BPG serves as a substrate for the enzyme phosphoglycerate kinase. In this reaction, a high-energy phosphate group from 1,3-BPG is transferred to adenosine diphosphate (ADP), resulting in the production of adenosine triphosphate (ATP). This process is known as substrate-level phosphorylation, where ATP is generated directly during the glycolytic pathway.
- Regulation of Oxygen Release in Muscle: Although 1,3-BPG is not directly involved in oxygen transport, its levels indirectly influence the release of oxygen from hemoglobin in muscle tissues. High concentrations of 1,3-BPG in red blood cells decrease the affinity of hemoglobin for oxygen. This allows oxygen to be more readily released from hemoglobin and delivered to metabolically active tissues, such as muscles, where oxygen demand is higher.
Thioesters
- A thioester is a chemical compound that contains a functional group composed of a carbonyl group (C=O) bonded to a sulfur atom (S) instead of an oxygen atom (O). It is structurally similar to an ester, which has the carbonyl group bonded to an oxygen atom. In a thioester, the sulfur atom forms a bond with a carbon atom through the carbonyl carbon, resulting in a C=S bond.
- Thioesters are notable for their involvement in various biological and chemical processes. They play crucial roles in metabolism, particularly in the process of fatty acid synthesis and degradation. Thioesters are intermediates in these metabolic pathways, facilitating the transfer of acyl groups (acyl-CoA) and participating in reactions such as thioester hydrolysis and thioesterification.
- Additionally, thioesters are found in certain biochemical reactions, such as the formation of peptide bonds during protein synthesis. The thioester bond in the active site of enzymes called thioesterases enables the catalysis of various reactions.
- Thioesters also find applications in synthetic organic chemistry, where they are utilized as intermediates for the synthesis of complex molecules. They can undergo various chemical transformations, including nucleophilic additions and substitutions, making them versatile building blocks in organic synthesis.
- Overall, thioesters are important compounds with diverse roles in biology and chemistry, and their unique structural characteristics contribute to their functional properties and reactivity.
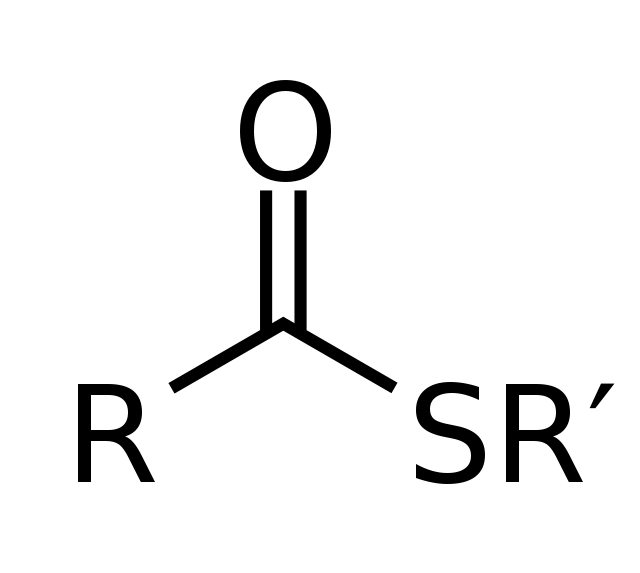
Structure of Thioesters
The structure of thioesters is similar to that of esters, with the key difference being the substitution of the oxygen atom in the carbonyl group with a sulfur atom. Here is the general structure of a thioester:
R-C(=O)-SR’
In this structure:
- R represents an organic group or hydrogen atom bonded to the carbonyl carbon.
- C(=O) denotes the carbonyl group, which consists of a carbon atom double-bonded to an oxygen atom.
- S represents the sulfur atom, which replaces the oxygen atom found in esters.
- R’ represents an organic group or hydrogen atom bonded to the sulfur atom.
The carbon-sulfur bond is a covalent bond formed between the carbonyl carbon and the sulfur atom, resulting in a thioester. The sulfur atom is capable of forming a stable bond with the carbon atom due to similar electronic properties to oxygen, allowing for thioesters to exhibit reactivity and participate in various chemical reactions.
Thioesters can vary in their organic groups (R and R’) attached to the carbonyl carbon and sulfur atom, respectively. The specific arrangement of these organic groups contributes to the overall structure and properties of individual thioester compounds.
It’s worth noting that the general structure provided above represents a simplified view, and the actual structure of a thioester may exhibit additional features depending on the specific compound and its surroundings.
Functions of Thioesters
Thioesters serve several important functions in various biological and chemical processes. Here are some of their key functions:
- Energy storage and transfer: Thioesters are involved in energy storage and transfer in living organisms. For example, acyl-CoA molecules, which are thioesters of fatty acids with coenzyme A (CoA), play a central role in cellular energy metabolism. Acyl-CoA molecules serve as carriers of activated fatty acids, facilitating their oxidation for energy production or their incorporation into lipid synthesis pathways.
- Fatty acid synthesis and degradation: Thioesters are crucial intermediates in fatty acid synthesis and degradation pathways. Acyl carrier protein (ACP), a protein that binds fatty acid intermediates as thioesters, is involved in fatty acid synthesis. Thioester linkages in ACP facilitate the transfer of acyl groups between enzymes, allowing the stepwise elongation of fatty acid chains. Similarly, during fatty acid degradation, thioester intermediates such as acyl-CoA are formed and subsequently metabolized to release energy.
- Protein synthesis: Thioesters play a role in protein synthesis, specifically in the formation of peptide bonds. During translation, an activated amino acid is attached to a transfer RNA (tRNA) molecule through a thioester linkage, forming an aminoacyl-tRNA. This aminoacyl-tRNA serves as the substrate for peptide bond formation, enabling the elongation of polypeptide chains during protein synthesis.
- Enzymatic reactions: Thioesters are involved in various enzymatic reactions. Enzymes called thioesterases catalyze the hydrolysis of thioester bonds, leading to the cleavage of thioester intermediates. Thioesterases are essential in processes such as fatty acid degradation and the release of metabolites from thioester derivatives.
- Chemical synthesis: Thioesters find applications in synthetic organic chemistry. They serve as versatile building blocks and intermediates in various chemical reactions. Thioesters can undergo nucleophilic additions and substitutions, allowing for the synthesis of complex molecules and the introduction of functional groups.
Overall, thioesters play crucial roles in energy metabolism, fatty acid metabolism, protein synthesis, enzymatic reactions, and chemical synthesis. Their ability to store and transfer energy, participate in key biochemical pathways, and exhibit reactivity makes them essential compounds in biological systems and valuable tools in chemical synthesis.
ATP
ATP stands for adenosine triphosphate. It is a molecule that serves as the primary energy currency in living organisms. ATP is often referred to as the “molecular unit of currency” because it provides energy for cellular processes.
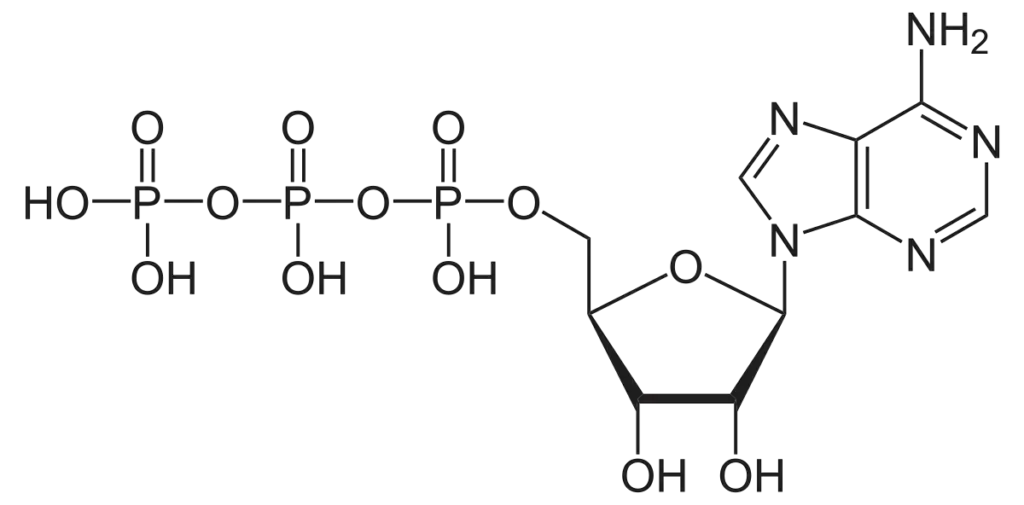
The structure of ATP consists of three main components:
- Adenosine: This is a nucleoside composed of the nitrogenous base adenine and the sugar ribose.
- Triphosphate: ATP contains three phosphate groups (hence “triphosphate”) linked together by high-energy phosphoanhydride bonds. These phosphate groups are labeled α, β, and γ, with γ being the closest to the ribose sugar.
The high-energy phosphate bonds in ATP are crucial for its role as an energy carrier. When one of the phosphate groups is cleaved from ATP through a hydrolysis reaction, energy is released. This energy can be harnessed and used by cells to drive various biological processes.
ATP is involved in numerous cellular activities, including:
- Energy transfer: ATP transfers energy to other molecules in the cell, making it available for various endergonic (energy-consuming) reactions. For example, ATP provides the energy needed for muscle contraction, active transport of ions across cell membranes, and synthesis of macromolecules like proteins and nucleic acids.
- Enzymatic reactions: Many enzymes require ATP as a cofactor to catalyze chemical reactions. ATP often serves as a source of phosphate groups that can be transferred to specific substrates, activating them or facilitating reactions.
- Signal transduction: ATP can function as an extracellular signaling molecule. It can be released from cells and interact with specific cell surface receptors, initiating signal transduction pathways and regulating various physiological processes.
- Nucleic acid synthesis: ATP is a precursor for the synthesis of RNA and DNA. During nucleic acid synthesis, ATP provides the necessary energy and phosphate groups for the formation of phosphodiester bonds between nucleotides.
Importantly, ATP is not a long-term energy storage molecule. Cells must constantly replenish ATP levels through processes like cellular respiration, photosynthesis (in plants), and other metabolic pathways. These processes convert energy from nutrients or light into ATP, ensuring a continuous supply of energy for cellular activities.
In summary, ATP is a vital molecule involved in energy transfer, enzymatic reactions, signal transduction, and nucleic acid synthesis. Its high-energy phosphate bonds make it a versatile and universal energy carrier in living systems.
FAQ
What are energy-rich compounds?
Energy-rich compounds are molecules that store and release large amounts of energy, which can be used for various biological processes.
What is the primary energy-rich compound in living organisms?
The primary energy-rich compound in living organisms is adenosine triphosphate (ATP). It serves as the universal energy currency in cells.
How is ATP synthesized?
ATP is synthesized through cellular respiration or photosynthesis, depending on the organism. These processes convert energy from nutrients or light into ATP through complex biochemical reactions.
How is energy released from ATP?
Energy is released from ATP when one of its phosphate groups is hydrolyzed through the action of enzymes. This hydrolysis breaks the high-energy phosphate bond, releasing energy.
Can other molecules serve as energy-rich compounds?
Yes, other molecules can serve as energy-rich compounds. Examples include guanosine triphosphate (GTP), creatine phosphate, and certain high-energy thioesters.
How is energy stored in energy-rich compounds?
Energy is stored in energy-rich compounds through the formation of high-energy bonds, such as phosphoanhydride bonds. These bonds have a significant amount of potential energy, which can be released upon hydrolysis.
What is the role of energy-rich compounds in metabolism?
Energy-rich compounds like ATP play a central role in metabolism by providing energy for various cellular processes, including biosynthesis, active transport, and muscle contraction.
Can energy-rich compounds be regenerated?
Yes, energy-rich compounds can be regenerated through processes such as phosphorylation. For example, ADP (adenosine diphosphate) can be phosphorylated to regenerate ATP using energy derived from metabolic reactions.
Are energy-rich compounds only found in cells?
Energy-rich compounds are primarily found within cells, where they are involved in intracellular energy transfer. However, they can also be found in extracellular spaces, where they may have signaling functions.
How do energy-rich compounds relate to overall energy balance in organisms?
Energy-rich compounds play a crucial role in maintaining the overall energy balance in organisms. They store energy from nutrient breakdown or light absorption and release it as needed to support essential biological processes, maintaining energy homeostasis within the organism.