What is DNA Replication?
- DNA replication is the biological process in which a DNA molecule is duplicated to produce two identical copies. This process is vital for cell division, growth, and the repair of damaged tissues. It ensures that every new cell inherits an accurate copy of the genetic material. DNA replication occurs in all living organisms and serves as a cornerstone of genetic inheritance.
- The structure of DNA is a double helix, consisting of two complementary strands that run in opposite directions. Each strand is composed of nucleotides, which include a deoxyribose sugar, a phosphate group, and one of four nitrogenous bases (adenine, thymine, cytosine, or guanine). The base-pairing rules—adenine pairing with thymine and cytosine pairing with guanine—maintain the double-stranded structure through hydrogen bonds. During replication, these hydrogen bonds are broken, allowing the two strands to separate.
- DNA replication is described as semi-conservative. This means that each newly formed DNA molecule consists of one strand from the original molecule and one newly synthesized strand. This mechanism ensures the preservation of genetic information across generations of cells.
- Replication begins at specific sites on the DNA called origins of replication. At these sites, the enzyme helicase unwinds the DNA helix, creating replication forks that move in both directions. Proteins stabilize the unwound DNA and assist in the replication process. DNA polymerase is the primary enzyme responsible for synthesizing new DNA strands by adding complementary nucleotides to each template strand. This synthesis occurs during the S-phase of the cell cycle in interphase.
- The process involves several additional proteins and enzymes, such as primase, which synthesizes short RNA primers to initiate DNA synthesis, and ligase, which seals nicks in the sugar-phosphate backbone of the new DNA strands. Proofreading mechanisms ensure high accuracy, correcting errors that may occur during nucleotide incorporation.
- In addition to occurring naturally within cells, DNA replication can be replicated artificially in a laboratory setting using techniques like the polymerase chain reaction (PCR). This method utilizes DNA polymerases, primers, and template DNA to amplify specific DNA sequences in vitro, enabling detailed genetic analysis and molecular biology applications.
- DNA replication’s precision and efficiency are critical for maintaining the integrity of genetic material, underpinning cellular functions, and supporting the continuity of life.
The goal for DNA replication is guarantee the accuracy of transfer in genetic info from one generation to the following. DNA replication is a crucial procedure that occurs prior to cell division and serves a variety of functions:
- Genetic Replication: The process of DNA replication makes sure that every daughter cell receives an exact replica that contains the same genetic content. It ensures the accurate transfer of information about genetics from mother cells back to the daughter cell during the process of cell division.
- Growth and development: DNA replication permits cells to divide and grow which allows for the development and maintenance of organs and tissues. In the process of producing two identical duplicates of DNA molecules, DNA replication supplies the genetic material that allows new cells to grow during tissue repair or growth processes.
- Repair and maintenance: DNA replication plays a part in the DNA repair mechanism. If DNA is damaged by external causes or mistakes in replication, cells are able to make use of the unaffected DNA strand to repair the damaged area during the process of replication.
- Genetic Evolution as well as Genetic Diversity: DNA replication is crucial for the creation of genetic diversity and also the development process. Replication that is accurate assures that genetic changes like mutations, or Recombination events, are effectively transmitted to generations afterward.
The main purpose in DNA replication is ensure DNA’s integrity, and to ensure the correct transmission of information genetic which allows for the growth, development and longevity of livin
Definition of DNA Replication
DNA replication is the biological process by which a cell duplicates its DNA molecule, producing two identical copies from one original DNA strand, ensuring the transmission of genetic information during cell division.
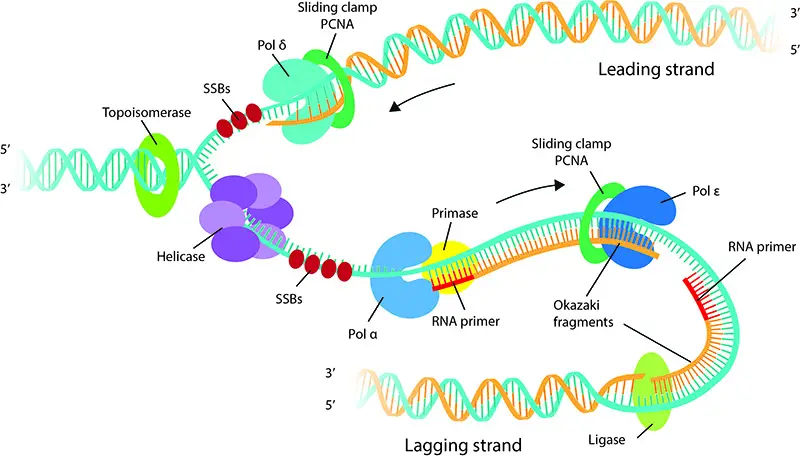
DNA synthesis is a meticulously orchestrated process that ensures the accurate replication of genetic information. The directionality and manner in which this synthesis occurs are fundamental to its precision.
Directionality of DNA Synthesis (5′-3′ Direction): DNA synthesis always proceeds in the 5′-3′ direction. This is because the enzymes responsible for adding nucleotides, known as DNA polymerases, can only add nucleotides to the free 3′ hydroxyl (OH) group of the growing DNA chain. As the synthesis progresses, nucleotides are sequentially added to this 3′ end, ensuring the elongation of the new strand in the 5′-3′ direction.
Semidiscontinuous Nature of DNA Synthesis: The two strands of the DNA double helix run in antiparallel directions, meaning one strand runs from 5′ to 3′ while the other runs from 3′ to 5′. Given that DNA synthesis can only proceed in the 5′-3′ direction, this poses a challenge when replicating both strands simultaneously as the replication fork progresses.
To address this challenge:
- Leading Strand Synthesis: One strand, termed the “leading strand,” is synthesized continuously in the 5′-3′ direction, moving with the replication fork.
- Lagging Strand Synthesis: The other strand, known as the “lagging strand,” is synthesized discontinuously. This synthesis involves the creation of short DNA segments called Okazaki fragments, named after Reiji Okazaki who discovered them in the 1960s. For each fragment, synthesis starts with a short RNA primer, followed by DNA synthesis in the 5′-3′ direction. However, since this direction is opposite to the movement of the replication fork, the synthesis of the lagging strand occurs in a piecewise manner, producing multiple Okazaki fragments.
Once all the Okazaki fragments are synthesized, they are joined together by the enzyme DNA ligase to form a continuous DNA strand.
DNA Replication Enzymes and Proteins
DNA replication is a fundamental biological process, relying on various enzymes and proteins to ensure accurate and efficient copying of genetic material. Below are key enzymes and proteins involved in this intricate mechanism:
- Nucleases
- Nucleases cleave the phosphodiester bonds between nucleotides.
- Exonucleases remove nucleotides from the ends of a DNA molecule. They can function in both 5′ to 3′ and 3′ to 5′ directions.
- Endonucleases cleave within the DNA strand. These enzymes are highly selective, acting on either DNA (DNase) or RNA (RNase).
- Restriction endonucleases cut DNA at specific recognition sites, typically 4 to 8 base pairs long, generating fragments.
- DNA Polymerase
- DNA polymerases synthesize new DNA strands by adding nucleotides to a template strand.
- In prokaryotic cells, there are three types:
- DNA polymerase I (involved in repair and processing Okazaki fragments).
- DNA polymerase II (a repair polymerase).
- DNA polymerase III (primary polymerase for DNA replication).
- In eukaryotic cells, five types are identified:
- DNA polymerase α (repair and synthesis).
- DNA polymerase β (repair function).
- DNA polymerase γ (replicates mitochondrial DNA).
- DNA polymerase δ (synthesizes lagging strands).
- DNA polymerase ε (synthesizes leading strands and repairs DNA).
- DNA Ligase
- DNA ligase joins DNA strands by catalyzing the formation of phosphodiester bonds between nucleotides.
- This process requires ATP and is crucial for sealing breaks in the DNA backbone after replication.
- DNA Helicase
- DNA helicases are motor proteins that unwind double-stranded DNA, generating single-stranded regions known as replication forks.
- They break hydrogen bonds between nucleotide bases to allow each strand to be copied.
- Helicase activity is energy-driven, using ATP to separate the DNA strands.
- DNA Primase
- DNA primase synthesizes short RNA primers, providing the necessary 3′ hydroxyl group for DNA polymerases to begin nucleotide addition.
- These primers are later replaced with DNA once elongation proceeds.
- DNA Topoisomerase
- DNA topoisomerases relieve torsional strain during DNA replication by creating transient nicks in the DNA backbone.
- Class I topoisomerases make single-strand breaks to alleviate tension, while Class II topoisomerases break both strands.
- These enzymes are vital for preventing supercoiling and ensuring smooth progression of the replication fork.
- Single Strand Binding Proteins (SSBs)
- SSBs bind to single-stranded DNA, stabilizing it and preventing the strands from re-annealing or degrading.
- They assist in maintaining the DNA structure during replication, ensuring that the polymerase can access the template strand.
- These proteins also help in removing secondary structures, allowing the replication machinery to work efficiently.
DNA replication happens in DNA replication occurs during the S (synthesis) period of cell development. In eukaryotic cells that comprise human cells the cell cycle is comprised of distinct phases that include G1 (gap 1) (gap 1), S (synthesis) G2 (gap 2) as well as M (mitosis).
In the S phase in the S phase, cells’ DNA is replicated to prepare for cell division. It is a nitty-gritty procedure that involves unwinding in the structure known as the double helix and the division of DNA strands and the synthesis of complement strands by using already existing DNA strands for models. The result is the creation from two copies DNA molecules, also known as sister chromatids. These are linked by a region known as the centromere.
Following DNA replication in the S phase Cells progress into its G2 phase, in which it prepares for division of the cell. After G2 cells enter the M phase. In this stage, DNA replication is divided equally between two cells via the process known as mitosis.
It’s crucial to understand the fact that replication of DNA is an essential process that happens in all cells that divide to ensure the correct transfer of genetic information from an generation onto the following.
Summary Table of Enzymes and Proteins in DNA Replication
Enzyme/Protein | Function | Key Features |
---|---|---|
Nucleases | Cleave phosphodiester bonds in DNA or RNA. | – Exonucleases: Remove nucleotides from ends (5′ to 3′ or 3′ to 5′). – Endonucleases: Cleave within strands, e.g., restriction enzymes recognize specific sites. |
DNA Polymerase | Synthesizes new DNA strands using a template strand. | – Prokaryotic types: I (repair), II (repair), III (main replication). – Eukaryotic types: α, β, γ, δ, ε (various replication and repair functions). |
DNA Ligase | Joins DNA fragments by forming phosphodiester bonds. | – Seals nicks in the DNA backbone, requires ATP. |
DNA Helicase | Unwinds double-stranded DNA into single strands. | – Creates replication forks by breaking hydrogen bonds between base pairs. – Requires ATP for activity. |
DNA Primase | Synthesizes short RNA primers as starting points for DNA polymerases. | – Primers provide 3′ hydroxyl groups for nucleotide addition. – Primers are replaced with DNA during elongation. |
DNA Topoisomerase | Relieves torsional strain caused by helicase activity during replication. | – Class I: Single-strand breaks to relax supercoils. – Class II: Double-strand breaks to manage supercoiling. |
Single-Strand Binding Proteins (SSBs) | Stabilize single-stranded DNA and prevent it from re-annealing or degrading. | – Protects strands and removes secondary structures, enabling efficient replication and repair. |
DNA Replication Overview
DNA replication is the process by which a cell duplicates its DNA to ensure genetic information is transmitted to the next generation of cells. It involves three main steps: strand separation, priming, and the synthesis of new DNA strands. Below is a detailed breakdown of the process.
- Step 1: Opening the DNA Helix
- The double-stranded DNA uncoils and separates at a specific starting point called the origin of replication (e.g., oriC in E. coli).
- DNA helicase unwinds the helix, breaking hydrogen bonds between the base pairs, creating single-stranded regions.
- This unwinding forms a structure known as the replication fork.
- Step 2: Priming the Template Strands
- DNA primase synthesizes short RNA primers on the single-stranded DNA.
- These primers provide a 3′ hydroxyl group, necessary for DNA polymerase to begin adding nucleotides.
- Step 3: Assembly of New DNA Strands
- DNA polymerase organizes the synthesis of new DNA strands by adding nucleotides complementary to the template strands.
- In E. coli, DNA polymerase III is the primary enzyme driving the elongation process.
- Role of Enzymes and Proteins
- Enzymes are critical at every stage of DNA replication, catalyzing essential reactions:
- Helicase unwinds the DNA.
- Primase lays down primers for initiation.
- Polymerase builds the new DNA strands.
- Topoisomerase alleviates supercoiling caused by helicase activity.
- Single-strand binding proteins stabilize the unwound strands to prevent re-annealing or degradation.
- Enzymes are critical at every stage of DNA replication, catalyzing essential reactions:
- Organism-Specific Variations
- While the basic mechanism is conserved across organisms, specific details vary based on cell type.
- In prokaryotes like E. coli, replication starts at the oriC site, where DnaA proteins bind and initiate the process, utilizing ATP hydrolysis.
- Eukaryotic cells, in contrast, have multiple origins of replication to handle their larger genome size.
DNA is a double-stranded molecule that forms the genetic blueprint for all living organisms. Its unique structure involves a set of four types of nucleotides, each with a specific role in encoding genetic information. Understanding the architecture of DNA is crucial for explaining genetic functions and processes.
- Double Helix Formation:
DNA consists of two strands that coiled together to form a double helix.
Each strand is made of a chain of nucleotides, which are the building blocks of DNA. - Nucleotide Composition:
Nucleotides are the basic units of DNA and consist of three components:- Deoxyribose sugar
- Phosphate group
- Nucleobase: The four types of nucleobases are adenine (A), cytosine (C), guanine (G), and thymine (T).
These bases are divided into two categories:
- Purines: Adenine and guanine
- Pyrimidines: Cytosine and thymine
- Base Pairing:
The strands of DNA are held together by hydrogen bonds between complementary bases:- Adenine (A) pairs with thymine (T) using two hydrogen bonds.
- Guanine (G) pairs with cytosine (C) using three hydrogen bonds.
- Phosphodiester Bonds:
The backbone of the DNA strand is formed by phosphodiester bonds. These bonds link the 5′ carbon of one nucleotide’s sugar to the 3′ carbon of the next nucleotide’s sugar. This creates a continuous sugar-phosphate backbone.
Phosphodiester bonds are stronger than hydrogen bonds, ensuring stability within the strand. - Strand Directionality:
DNA strands have inherent directionality, labeled as 5′ to 3′.- The 5′ end refers to the end where the phosphate group is attached to the 5′ carbon of the deoxyribose sugar.
- The 3′ end refers to the end where the hydroxyl group (-OH) is attached to the 3′ carbon.
The two strands in the double helix run anti-parallel, meaning one strand runs 5′ to 3′, and the opposite strand runs 3′ to 5′.
- Replication and Synthesis:
DNA replication relies on the directionality of the strands.
DNA polymerase synthesizes a new strand by adding nucleotides to the 3′ end of the existing strand, ensuring replication only occurs in the 5′ to 3′ direction. - Strand Separation:
The two strands of the double helix are held together by hydrogen bonds between complementary bases. These bonds are weaker compared to phosphodiester bonds, allowing the strands to separate when necessary, such as during replication or transcription. - Redundancy in Information:
Due to complementary base pairing, the information in one strand of DNA can be inferred from the other. This redundancy is vital for error correction and replication accuracy.
DNA replication takes place within the nucleus cells that are eukaryotic. Eukaryotic cells, including the ones found within humans as well as other organisms with multicellularity, possess an individual compartment known as the nucleus in which DNA is kept.
In the nucleus of the cell DNA replication happens in a highly controlled and coordinated way. The process involves unwinding of the double helix, as well as the formation of new strands that complement each other. Specific proteins and enzymes are involved in the process of replication in order to guarantee the precision and efficacy in DNA replication.
In prokaryotic cell types, which comprise bacteria, the process of DNA replication takes place in the cytoplasm because there is no nucleus. Within these cell types, DNA is found as an elongated molecule. replication may occur at one specific location known as the site of replication.
In the end, whether within the nucleus in eukaryotic cells, or the prokaryotic cell cytoplasm DNA replication is an essential procedure that enables the reliable transmission of genetic information another generation.
DNA Replication Steps (DNA Replication Process)

Formation of Replication Fork
The replication fork forms when the double-stranded DNA unwinds, allowing the replication process to begin. This step is essential for exposing the template strands needed for synthesizing new DNA.
- Unwinding the DNA
- The double helix opens when hydrogen bonds between the complementary bases are broken.
- This unwinding starts at specific regions rich in adenine (A) and thymine (T), known as the origin of replication (Ori).
- These regions are targeted by initiator proteins, which recruit additional factors required for replication.
- Role of DNA Helicase
- DNA helicase is the enzyme responsible for unwinding the helix.
- It separates the two strands at the origin, creating a Y-shaped structure known as the replication fork.
- As helicase moves along the DNA, it continues exposing single strands to serve as templates.
- Managing Topological Stress
- Unwinding the DNA generates torsional strain, leading to supercoiled regions ahead of the replication fork.
- Topoisomerase alleviates this strain by introducing negative supercoils, ensuring smooth progression of the replication machinery.
- Bidirectional Fork Movement
- The replication fork operates in two directions.
- The leading strand is synthesized continuously in the 5′ to 3′ direction, following the helicase.
- The lagging strand, oriented 3′ to 5′, requires periodic synthesis of short fragments (Okazaki fragments) to maintain 5′ to 3′ elongation.
- Initiation of Replication
- Formation of the replication fork exposes single-stranded templates.
- This structural change marks the beginning of the DNA replication process, ensuring access to the genetic code for duplication.
1. Initiation
Initiation begins once the replication fork exposes single-stranded DNA, setting the stage for new strand synthesis. This step involves stabilizing the template strands and preparing them for elongation.
- Strand Orientation
- DNA strands run in opposite directions: the leading strand is oriented 5′ to 3′ toward the fork, while the lagging strand runs 3′ to 5′ away from it.
- This difference in orientation affects how new strands are synthesized.
- Stabilization of Single Strands
- Single-stranded DNA-binding proteins (SSBs) attach to the exposed strands.
- These proteins prevent the strands from recoiling and help maintain their structure during replication.
- Role of DNA Primase
- DNA primase synthesizes short RNA primers on the single-stranded templates.
- These primers are essential because they provide the free 3′ hydroxyl group needed for DNA polymerase to begin nucleotide addition.
- Primer Placement
- On the leading strand, a single primer is sufficient to initiate continuous synthesis.
- On the lagging strand, primers are placed at multiple points to allow the synthesis of short Okazaki fragments.
2. Elongation

Elongation is the phase where the actual synthesis of new DNA strands occurs. Once primers are in place, DNA polymerase begins adding nucleotides to form the new strands.
- Leading Strand Synthesis
- The leading strand runs from 5′ to 3′ toward the replication fork.
- DNA polymerase adds nucleotides continuously in the 5′ to 3′ direction.
- Because the strand is oriented in the correct direction, the process happens without interruption. Only one primer is needed to initiate this synthesis.
- Lagging Strand Synthesis
- The lagging strand runs in the opposite direction (3′ to 5′), which means DNA polymerase cannot add nucleotides continuously.
- As the replication fork advances, the lagging strand needs multiple primers to begin nucleotide synthesis.
- The synthesis on the lagging strand occurs in fragments, known as Okazaki fragments.
- Fragmentation of the Lagging Strand
- As DNA polymerase works in the opposite direction, the lagging strand is built in small segments.
- These short stretches of DNA are called Okazaki fragments and are later joined together by DNA ligase.
4. Termination
Termination marks the end of DNA replication, where the newly synthesized strands are finalized and any errors are corrected. This process ensures that the replication machinery stops at the right point, and the DNA strands are complete.
- Primer Removal and DNA Sealing
- RNA primers are removed from both the leading and lagging strands by the exonuclease activity of DNA polymerase.
- The gaps left behind after primer removal are filled with DNA, and the resulting nicks are sealed by DNA ligase.
- Proofreading and Error Correction
- DNA polymerase performs proofreading during elongation to ensure that errors in nucleotide incorporation are caught.
- If mistakes are found, the incorrect nucleotides are removed and replaced with the correct ones.
- End Replication Problem in Eukaryotes
- Eukaryotic chromosomes are linear, and when the RNA primer at the 5′ end of the daughter strand is removed, there is no 3′ hydroxyl group available for DNA polymerase to fill the gap.
- This results in a missing segment of DNA at the end of the strand.
- The gap is filled by special sequences called telomeres, which contain G:C-rich repeats. These telomeres don’t code for proteins but are crucial for protecting the DNA from loss of genetic information.
- Termination Sequence Recognition
- DNA replication halts when the replication forks reach specific termination sequences known as ter sequences.
- These sequences, approximately 23 base pairs long, serve as binding sites for the TUS protein.
- The ter-TUS complex binds to these sequences and halts the movement of the replication fork, effectively terminating the replication process.
Step Name | Description |
---|---|
1. Initiation | DNA synthesis begins at designated sites in the template strand’s coding regions called origins. Initiator proteins target these sites and assemble a replication complex. |
2. Elongation | DNA polymerase grows the new DNA daughter strand by attaching to the original unzipped template strand and the initiating short RNA primer. One strand is synthesized continuously (leading strand) while the other is synthesized in fragments (lagging strand). |
3. Termination | After synthesis, exonuclease removes all RNA primers from the original strands. The primers are replaced with the right nucleotide bases. DNA ligase then joins the Okazaki fragments. |
What is Okazaki Fragment?
Okazaki fragments are short DNA sequences synthesized on the lagging strand during DNA replication. These fragments are crucial for maintaining the integrity of the DNA strand when replication occurs in opposite directions.
- Lagging Strand Synthesis
- The two DNA strands are antiparallel, meaning one strand is synthesized in the 5′ to 3′ direction, while the other runs in the opposite direction, 3′ to 5′.
- DNA polymerase can only add nucleotides in the 5′ to 3′ direction. Therefore, the strand running 3′ to 5′ must be synthesized differently.
- Formation of Okazaki Fragments
- The lagging strand is synthesized in small, discontinuous segments called Okazaki fragments.
- These fragments are created by the extension of RNA primers, which are short RNA sequences that initiate the replication of the lagging strand.
- RNA Primers
- Unlike the leading strand, the lagging strand synthesis requires RNA primers.
- RNA primers are synthesized by the enzyme primase and are 3-10 nucleotides long. They are complementary to the lagging strand template at the replication fork.
- These primers serve as starting points for DNA polymerase to extend and create Okazaki fragments.
- Joining of Okazaki Fragments
- Once the Okazaki fragments are synthesized, they are linked together by the enzyme DNA ligase, forming a continuous strand.
- The process of joining these fragments is critical to complete the lagging strand synthesis.
- Critical Role of RNA
- Despite being part of the DNA replication process, Okazaki fragments contain RNA-DNA joints.
- This highlights the essential role of RNA primers in the replication of the lagging strand, bridging the gap between RNA and DNA synthesis.
DNA polymerase is a family of enzymes essential for DNA replication. These enzymes are responsible for adding nucleotides to a growing DNA strand, ensuring accurate and efficient replication. DNA polymerase functions by extending an existing strand of nucleotides, utilizing a template strand to guide the addition of new nucleotides.
- Initiation and Primer Requirement:
DNA polymerase cannot begin synthesizing a new strand independently.
To start, a short RNA primer is needed, which is created and paired with the template DNA strand. - Extension Process:
Once the primer is in place, DNA polymerase extends the strand by adding new nucleotides.
The new nucleotides are selected based on their complementarity to the template strand.
The addition occurs at the 3′ end of the growing DNA strand, with each new nucleotide connected via phosphodiester bonds. - Energy Source:
The energy required for DNA polymerization comes from the hydrolysis of high-energy phosphate bonds attached to each nucleotide.
These nucleotides, known as nucleoside triphosphates, consist of a base attached to three phosphate groups.
When a nucleotide is added to the DNA strand, the phosphate bond between the new nucleotide and the existing chain is broken, releasing two phosphate groups as pyrophosphate. - Proofreading and Error Correction:
DNA polymerases are highly accurate in their synthesis.
The intrinsic error rate is less than one mistake for every 10 million nucleotides added.
Many DNA polymerases also have proofreading capabilities, where they can delete mismatched nucleotides from the end of the strand.
This proofreading process helps correct errors during DNA synthesis, improving accuracy. - Post-replication Repair:
After DNA replication, there are additional mismatch repair mechanisms that can detect errors in the newly synthesized strand.
These mechanisms can distinguish between the newly synthesized strand and the original template strand, ensuring that mismatched bases are corrected. - Replication Speed:
The rate of DNA replication is remarkably fast.
For example, during phage T4 DNA synthesis in E. coli at 37°C, the elongation rate is approximately 749 nucleotides per second. - Mutation Rate:
Despite the high accuracy, occasional errors do occur.
The mutation rate during T4 DNA replication is about 1.7 mutations per 10^8 base pairs.
What is Replication Fork?

The replication fork is a key site where DNA replication occurs. It is where the DNA double helix unwinds, and both strands are used as templates to create new DNA sequences.
- Formation of the Replication Fork
- The replication fork is formed when the helicase enzyme unwinds the DNA helix, exposing single-stranded regions at the origin of replication.
- At the fork, primase synthesizes short RNA primers to initiate the replication process.
- DNA polymerase then elongates the new DNA strand by adding nucleotides.
- Bidirectional Nature
- The replication fork operates in a bi-directional manner.
- This means replication occurs in two opposite directions from the fork—one on the leading strand and the other on the lagging strand.
- Leading Strand Synthesis
- The leading strand is synthesized continuously in the 5′ to 3′ direction.
- After the DNA strands separate, a short RNA primer is made at the 5′ end, which allows DNA polymerase to start adding nucleotides.
- This process is uninterrupted, as the DNA polymerase moves in the same direction as the replication fork.
- Lagging Strand Synthesis
- The lagging strand is synthesized in the 3′ to 5′ direction, opposite to the movement of the fork.
- Due to this, replication on the lagging strand is not continuous but instead occurs in small segments known as Okazaki fragments.
- These fragments are formed by RNA primers, with DNA polymerase extending the primers and adding nucleotides.
- Synthesis Process
- As the DNA strands continue to separate, the exposed 3′ to 5′ template strands are used for the discontinuous synthesis of Okazaki fragments on the lagging strand.
- These fragments are later joined together by DNA ligase, forming a continuous lagging strand.
Role of Enzymes in DNA Replication
DNA replication relies on a series of specialized enzymes to ensure that genetic material is accurately copied. These enzymes interact in a well-coordinated manner, each fulfilling distinct functions necessary for the process.
- DNA Polymerase
- The core enzyme in DNA replication is DNA-dependent DNA polymerase, which catalyzes the polymerization of the new DNA strand.
- It uses deoxyribonucleoside triphosphates as substrates and provides the energy needed for the process.
- There are three types of DNA polymerase:
- DNA Polymerase I: Primarily involved in DNA repair. It performs three activities:
- 5′ to 3′ polymerase activity
- 5′ to 3′ exonuclease activity
- 3′ to 5′ exonuclease activity
- DNA Polymerase II: Responsible for primer extension and proofreading during replication.
- DNA Polymerase III: The main enzyme responsible for in vivo DNA replication.
- DNA Polymerase I: Primarily involved in DNA repair. It performs three activities:
- Helicase
- Helicase unwinds the DNA double helix by breaking the hydrogen bonds between the two strands.
- This action creates the replication fork, a critical structure for DNA replication.
- Ligase
- Ligase is essential for joining the Okazaki fragments on the lagging strand.
- It seals the nicks between these fragments, ensuring the continuity of the newly synthesized strand.
- Primase
- Primase synthesizes the RNA primer that is complementary to the DNA template strand.
- This primer serves as the starting point for DNA polymerase to begin adding nucleotides.
- Endonucleases
- Endonucleases are enzymes that make cuts in the DNA strand, either single-stranded or double-stranded.
- These cuts are important for repairing or modifying DNA.
- Single-Stranded Binding Proteins
- Single-stranded binding proteins (SSBPs) bind to the single-stranded DNA during replication.
- They prevent the DNA from re-annealing or forming secondary structures, ensuring that the single-stranded DNA remains available for replication.
DNA Replication Process in Prokaryotes
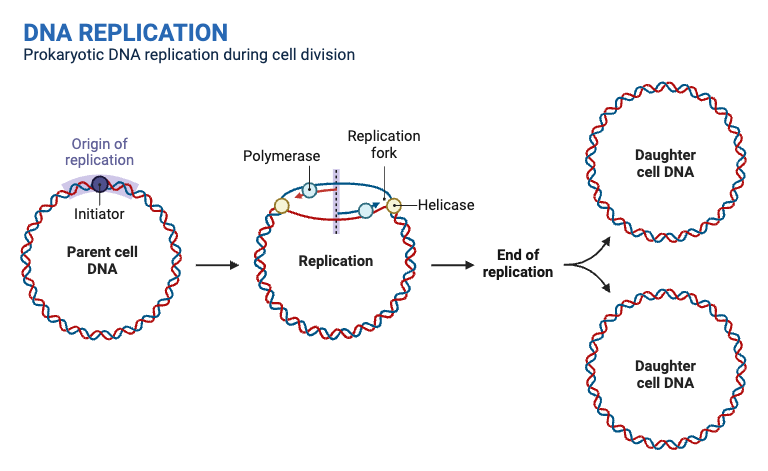
DNA replication in prokaryotes follows a well-defined sequence of events, involving several enzymes working together to accurately duplicate the genetic material. This process occurs at the origin of replication and is tightly regulated to ensure proper DNA synthesis.
- Initiation at the Origin of Replication
- The process begins when the DNA strands unwind at the origin of replication.
- Helicase unwinds the DNA further by breaking the hydrogen bonds between the two strands, creating the replication fork.
- Prevention of DNA Rewinding
- To prevent the DNA from rewinding, single-strand binding proteins coat the unwound DNA around the replication fork.
- This ensures the strands remain separated and available for replication.
- Managing DNA Supercoiling
- Topoisomerase alleviates the tension and prevents supercoiling of the DNA as it unwinds.
- This allows the replication fork to proceed smoothly without the formation of tangled DNA.
- Primer Synthesis
- Primase synthesizes RNA primers, which are complementary to the single-stranded DNA template.
- These primers provide a starting point for DNA polymerase to begin adding nucleotides.
- DNA Elongation
- DNA polymerase III starts adding nucleotides at the 3′ end of the RNA primer, elongating the new DNA strand.
- The process occurs continuously on the leading strand and discontinuously on the lagging strand, forming Okazaki fragments.
- Primer Removal and DNA Repair
- Once the DNA strands are elongated, the RNA primers are removed.
- DNA polymerase I fills in the gaps left by the primers.
- Finally, ligase seals the DNA fragments together, ensuring the integrity of the new strand.
This process of DNA replication in prokaryotes ensures that the genetic material is accurately copied and passed on to the next generation of cells. Each step is crucial for maintaining the fidelity of the replication process.
Erwin Chargaff, a prominent biochemist, made a pivotal observation regarding the composition of DNA, leading to what is now known as Chargaff’s Rule. Through his research, Chargaff discerned a consistent relationship between the nitrogenous bases present in DNA. Specifically, he noted that the quantity of adenine (A) always equaled the quantity of thymine (T), and similarly, the quantity of cytosine (C) was always equivalent to the quantity of guanine (G).
Mathematically, this relationship can be represented as: A = T and C = G
This discovery was foundational in understanding the pairing mechanism of these bases in the DNA double helix structure. Chargaff’s Rule essentially posits that in any given DNA molecule, the proportion of purine bases (A and G) will always match the proportion of pyrimidine bases (T and C), maintaining the structural integrity and consistency of the DNA across diverse organisms.
DNA Replication Process in Eukaryotes
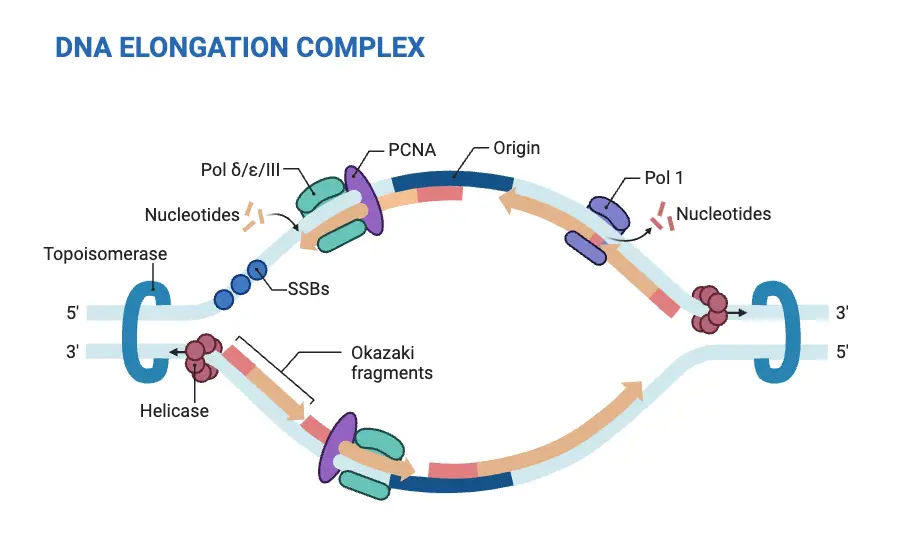
DNA replication in eukaryotic cells follows many of the same principles seen in prokaryotic replication, but it is more intricate due to the presence of multiple origins of replication and a more complex set of regulatory mechanisms.
- Multiple Origins of Replication
- Unlike prokaryotes, which have a single origin of replication, eukaryotes have multiple origins across their genome.
- These origins allow for the replication of the larger and more complex eukaryotic chromosomes in a timely manner.
- Pre-replication Complex
- Before DNA replication begins, eukaryotic cells assemble a pre-replication complex (pre-RC).
- This complex includes initiator proteins that help set up the replication machinery at each origin.
- Enzymes Involved in Replication
- While the basic steps of DNA replication are similar to prokaryotes, the enzymes involved differ.
- The main enzyme for DNA polymerization in eukaryotes is DNA polymerase δ (Pol δ), which performs the polymerization process.
- In prokaryotes, this role is carried out by DNA polymerase III.
- Replication Process
- The replication process begins with the unwinding of DNA by helicases.
- Primase synthesizes RNA primers, which are necessary for DNA polymerases to start elongation.
- Like in prokaryotes, eukaryotic polymerases add nucleotides to the growing strand, synthesizing the leading and lagging strands.
Enzymes involved in DNA Replication in the prokaryote, E. coli
E. coli Gene | Enzyme/Protein Function | Description |
---|---|---|
dnaA | Initiator Protein | Melts DNA at oriC, exposing two template ssDNA strands |
dnaB | Helicase | Unwinds the DNA helix at the front end of each replication fork during replication |
dnaC | Helicase Loader | Loads the DnaB Helicase onto the ssDNA template strands |
dnaG | Primase | Synthesizes RNA primers used to initiate DNA synthesis |
dnaE | α-Catalytic Subunit of DNA Polymerase III | Catalytic subunit of the main replicative polymerase during DNA replication |
dnaQ | ε-Editing Subunit of DNA Polymerase III | Editing subunit of the main replicative polymerase during DNA replication |
dnaN | β-clamp subunit of DNA Polymerase III | Clamping subunit of the main replicative polymerase during DNA replication |
polA | DNA Polymerase I | Processes Okazaki fragments and also fills in gaps during DNA repair processes |
polB | DNA Polymerase II | Proofreading and editing, especially on lagging strand synthesis and some involvement in DNA repair |
ssb | Single Stranded Binding Proteins (SSB) | Bind with single-stranded regions of DNA in the replication fork and prevent the strands from rejoining |
gyrA, gyrB | DNA Gyrase | Type II Topoisomerase involved in relieving positive supercoiling tension caused by the action of Helicase |
parC, parE | Topoisomerase IV | Type II Topoisomerase involved in decatenation of daughter chromosomes during DNA replication |
ligA | DNA Ligase | Fixes nicks in the DNA backbone during DNA replication, DNA damage, and DNA repair processes |
DNA, or deoxyribonucleic acid, is termed a polynucleotide molecule due to its structural composition. At its core, DNA is composed of smaller units known as nucleotides. Each nucleotide consists of three components: a phosphate group, a deoxyribose sugar, and one of four nitrogenous bases – deoxyadenylate (A), deoxyguanylate (G), deoxycytidylate (C), or deoxythymidylate (T).
When numerous nucleotides link together through phosphodiester bonds, they form a long chain. This chain, due to its composition of multiple nucleotides, is referred to as a polynucleotide. Given that DNA possesses two such chains that coil around each other to form the iconic double helix structure, it is aptly described as a molecule comprising two polynucleotide chains. This intricate arrangement ensures the storage and transmission of genetic information within organisms.
Differences between DNA replication in Eukaryotes and Prokaryotes
DNA replication is a fundamental process in all living organisms, ensuring that genetic information is passed from one generation to the next. Both prokaryotic and eukaryotic cells follow a semi-conservative method of DNA replication, but the process varies in complexity due to differences in their size and genome structure.
Similarities:
- Unwinding of DNA: Both prokaryotes and eukaryotes utilize specific enzymes to unwind the DNA double helix in preparation for replication.
- Role of DNA Polymerase: In both types of organisms, the enzyme DNA polymerase plays a pivotal role in synthesizing the new DNA strands.
- Semi-conservative Replication: Both prokaryotes and eukaryotes follow a semi-conservative replication model, where each of the two resulting DNA molecules has one old and one new strand.
- Okazaki Fragments: The lagging strand in both organisms is synthesized discontinuously in the form of Okazaki fragments.
- RNA Primers: Initiation of DNA replication in both prokaryotes and eukaryotes requires short RNA primers.
Differences:
- Genome Size: Eukaryotes typically have a much larger genome compared to prokaryotes. Eukaryotic cells possess about 25 times more DNA than prokaryotic cells.
- Origins of Replication: Eukaryotic DNA replication begins at multiple origins of replication, and it occurs unidirectionally within the cell’s nucleus. In contrast, prokaryotic DNA has a single origin of replication, and replication proceeds bidirectionally.
- DNA Polymerases: Eukaryotic cells have multiple types of DNA polymerases, whereas prokaryotes generally have fewer types.
- Rate of Replication: DNA replication is slower in eukaryotes, taking up to 400 hours, while in prokaryotes, it’s much faster, completing in about 40 minutes.
- Telomere Replication: Eukaryotic chromosomes have linear structures with telomeres at their ends, requiring a special mechanism for replicating telomeres. Prokaryotes, with their circular chromosomes, do not have telomeres.
- Timing of Replication: Eukaryotic cells replicate their DNA only during the S-phase of the cell cycle. In contrast, prokaryotic cells, not being bound by a defined cell cycle, can replicate their DNA almost continuously.
In conclusion, while there are foundational similarities in the DNA replication process of prokaryotes and eukaryotes, the differences arise due to the complexities of eukaryotic cellular and genomic structures.
Aspect | Eukaryotes | Prokaryotes |
---|---|---|
Unwinding of DNA | Both utilize enzymes to unwind DNA. | Both utilize enzymes to unwind DNA. |
Role of DNA Polymerase | DNA polymerase synthesizes new strands. | DNA polymerase synthesizes new strands. |
Replication Model | Semi-conservative replication. | Semi-conservative replication. |
Okazaki Fragments | Present in the lagging strand. | Present in the lagging strand. |
RNA Primers | Required for initiation. | Required for initiation. |
Genome Size | Larger genome (about 25 times more DNA). | Smaller genome. |
Origins of Replication | Multiple origins, unidirectional. | Single origin, bidirectional. |
DNA Polymerases | Multiple types. | One or two types. |
Rate of Replication | Slower (up to 400 hours). | Faster (about 40 minutes). |
Telomere Replication | Special mechanism for telomeres. | No telomeres (circular chromosomes). |
Timing of Replication | During S-phase of the cell cycle. | Almost continuously. |
Applications of DNA Replication
DNA replication is at the core of genetic processes, enabling the transmission of genetic information. It plays a critical role in maintaining cellular function and ensuring the integrity of an organism’s genome. Beyond its fundamental biological purpose, DNA replication has been harnessed in various fields, from clinical diagnostics to cutting-edge biotechnology.
- Genome Sequencing
- DNA replication allows for the accurate transfer of genetic information, a process that is crucial for genome sequencing.
- Whole human genome sequencing is now possible, providing detailed insights into an individual’s genetic makeup.
- Gene Cloning
- The ability to replicate DNA is central to gene cloning. By copying specific genes, scientists can study their function or create genetically modified organisms.
- Enzyme Applications
- The enzymes involved in DNA replication, such as DNA polymerase and primase, have been extensively studied for their broader applications.
- These enzymes are crucial for Polymerase Chain Reaction (PCR), a technique that amplifies DNA in vitro and is used in diagnostics, sequencing, and recombinant DNA technology.
- CRISPR and Cas9 Technology
- Advances in DNA replication have directly influenced technologies like CRISPR/Cas9.
- The CRISPR system uses nucleases to cut and edit specific DNA sequences, making it a powerful tool for gene editing and potential treatments for genetic disorders.
- Complementary DNA (cDNA)
- The process of DNA replication is also involved in the synthesis of complementary DNA (cDNA), which is crucial for understanding gene expression and cloning.
- Broad Applications in Genetic Engineering
- Any technique that involves genes relies on DNA replication. Whether in gene therapy, genetic modification, or diagnostic testing, replication ensures that the necessary genetic material is available for manipulation and analysis.
What is DNA replication stress?
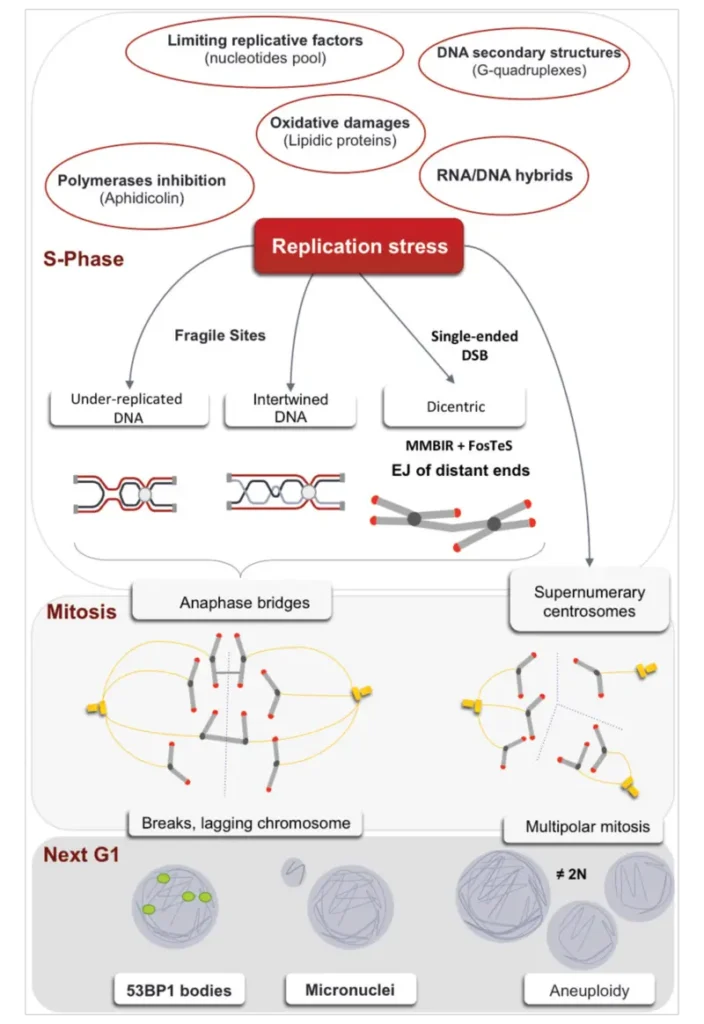
DNA replication stress refers to the various challenges and disruptions that occur during the process of DNA replication, impacting the accurate and efficient duplication of genetic material. This stress can lead to stalled replication forks and potential genomic instability. The following points outline the causes, consequences, and management of DNA replication stress:
- Causes of DNA Replication Stress
- Unusual DNA Structures: Abnormal DNA structures, such as G-quadruplexes and cruciforms, can impede the progression of the replication machinery, causing replication stress.
- Mismatched Ribonucleotides: Incorporation of ribonucleotides instead of deoxyribonucleotides can lead to mismatches and disruptions in the replication process.
- Concurrent Replication and Transcription: The simultaneous occurrence of DNA replication and transcription can create conflicts, leading to replication stress due to the collision between the replication machinery and RNA polymerase.
- Inadequate Availability of Replication Factors: Limited availability or dysfunction of essential replication factors, such as DNA polymerases and helicases, can hinder efficient DNA replication.
- Fragile Sites on DNA: Specific regions of the genome, known as fragile sites, are more prone to replication stress due to their structural complexity or high-density repetitive sequences.
- Overexpression of Oncogenes: The overexpression or constitutive activation of oncogenes can disrupt normal replication processes and induce replication stress.
- Inaccessible Chromatin: Dense or modified chromatin structures can hinder the access of replication machinery to DNA, contributing to replication stress.
- Consequences of DNA Replication Stress
- Stalled Replication Forks: Replication stress can lead to the stalling of replication forks, where the DNA replication process is halted, potentially resulting in incomplete or erroneous DNA synthesis.
- Fork Collapse: If replication forks are not stabilized, they may collapse, leading to double-strand breaks and genomic instability. This collapse can disrupt the integrity of the genome and contribute to mutagenesis.
- Management and Repair Mechanisms
- Regulatory Proteins: Proteins such as ATM (Ataxia Telangiectasia Mutated) and ATR (ATM and Rad3-Related) are crucial for managing replication stress. These kinases are recruited to sites of DNA damage and play a key role in stabilizing replication forks and coordinating repair processes.
- Activation of Repair Mechanisms: Upon fork stalling or collapse, cellular repair mechanisms are activated to address DNA damage. These include the homologous recombination repair pathway and the recruitment of repair factors to reassemble and restore replication forks.
- Overall Impact
- Genomic Instability: Persistent replication stress and failure to adequately resolve stalled replication forks can lead to genomic instability, contributing to various diseases, including cancer.
- Cellular Adaptation: Cells have evolved multiple strategies to manage and adapt to replication stress, ensuring the maintenance of genome integrity and proper cellular function.
Proofreading in DNA replication is a crucial mechanism that ensures the accuracy of the DNA copying process. It is an error-correcting procedure used to identify and correct incorrect nucleotides inserted during DNA synthesis. The following points explain the key aspects of proofreading:
- DNA Polymerase and Error Correction:
- DNA polymerases are the enzymes responsible for adding nucleotides during replication. However, errors can occur, such as inserting an incorrect base. Proofreading is an inbuilt function of DNA polymerase that allows the detection and correction of these mistakes.
- When an incorrect base is paired, the polymerase pauses and reverses by one base pair. Using its 3′ to 5′ exonuclease activity, it removes the mismatched base and replaces it with the correct one. This ensures that DNA replication proceeds with high accuracy.
- Types of Polymerases with Proofreading Activity:
- In bacteria, DNA polymerases I, II, and III have proofreading abilities. The exonuclease activity of these enzymes ensures that any errors made during replication are corrected before the process continues.
- In eukaryotic organisms, only the DNA polymerases delta and epsilon, which are involved in the elongation phase of replication, have proofreading capabilities.
- Impact on Mutation Rates:
- The efficiency of proofreading directly affects mutation rates. If the proofreading mechanism is impaired, such as through mutations in genes like DNA polymerase epsilon, the rate of mutations can increase dramatically. For example, human colorectal cancers can exhibit hypermutated genotypes when proofreading is lost.
- Different species exhibit variations in the extent of proofreading, which influences their mutation rates. Species with larger population sizes or greater genomic complexity may exhibit more efficient proofreading mechanisms.
- Post-Replication Error Correction:
- In some cases, errors may escape the immediate proofreading process during replication. These errors are corrected by a post-replication mechanism known as mismatch repair. In this process, enzymes recognize and excise incorrectly paired nucleotides, replacing them with the correct base to prevent permanent mutations.
- In bacteria like E. coli, mismatch repair distinguishes between newly synthesized and parental DNA strands by the presence of methyl groups on the parental strand. The newly synthesized strand, lacking these methyl groups, is identified as the source of the error. In eukaryotes, the mechanism is less clear but may involve recognition of nicks in the new DNA strand.
- Nucleotide Excision Repair:
- Besides proofreading, nucleotide excision repair is another mechanism that fixes errors, such as thymine dimers caused by UV radiation. Enzymes cut out the erroneous DNA segment, and DNA polymerase fills in the correct sequence, followed by ligase sealing the strand.
Mechanism of DNA Proofreading
DNA proofreading is an essential mechanism that ensures the accuracy of DNA replication by correcting errors in nucleotide pairing. Mistakes, if left uncorrected, can result in mutations, which may lead to serious consequences such as cancer. The proofreading mechanism is primarily driven by DNA polymerase, which verifies each newly added base to maintain replication fidelity.
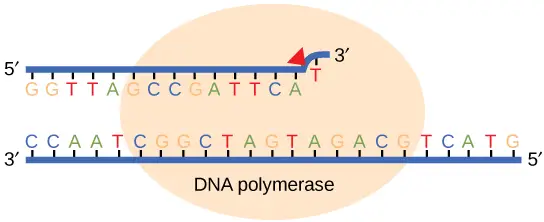
- Proofreading During Replication:
- DNA polymerase plays a dual role during replication, both synthesizing the DNA strand and proofreading it. As each new nucleotide is added, the enzyme checks whether the base has correctly paired with the template strand. If a correct match is detected, replication proceeds smoothly.
- In cases where an incorrect base is added, the DNA polymerase halts replication. Through its exonuclease activity, DNA polymerase III excises the incorrect nucleotide by breaking the phosphodiester bond. After removing the error, the enzyme inserts the correct nucleotide and resumes replication. This continuous verification and correction process ensures high accuracy during replication.
- Mismatch Repair Post-Replication:
- Some errors escape detection during replication and are corrected afterward through a process called mismatch repair. Specific enzymes recognize incorrectly paired bases and remove them from the newly synthesized strand. In E. coli, this is achieved by detecting the methylation patterns on the parental DNA strand—only the parental strand is methylated, allowing the enzymes to differentiate between old and new DNA.
- In eukaryotes, the exact mechanism is less well-understood, but it is thought to involve recognition of nicks in the newly synthesized strand. The repair proteins identify these nicks and remove the incorrect nucleotide, after which DNA polymerase fills in the gap with the correct base. The process concludes with DNA ligase sealing the newly added bases by creating a phosphodiester bond.
- Nucleotide Excision Repair:
- In some cases, DNA damage occurs due to external factors, such as UV radiation, which can cause thymine dimers. These dimers disrupt normal base pairing and must be repaired to prevent mutations. Nucleotide excision repair addresses these issues by cutting both the 3′ and 5′ ends of the incorrect base, removing the damaged segment of DNA.
- Once the damaged section is excised, DNA polymerase fills the gap with the correct nucleotides, and DNA ligase seals the strand. This mechanism is crucial for maintaining DNA integrity in cells exposed to environmental damage.
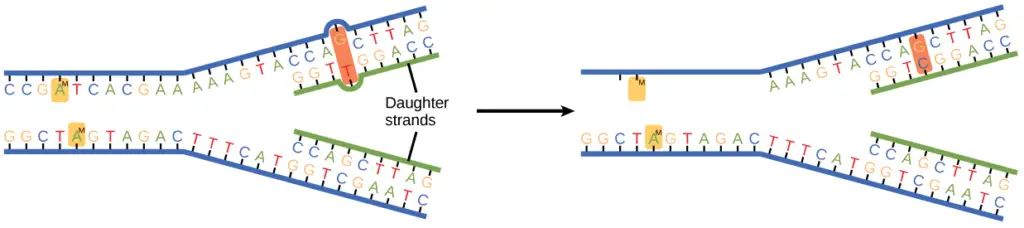
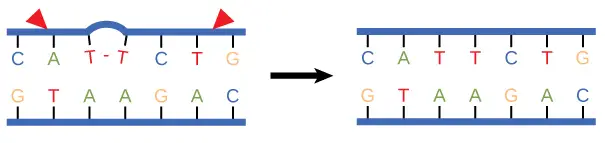
What is Chargaff’s Rule?
Erwin Chargaff, a prominent biochemist, made a pivotal observation regarding the composition of DNA, leading to what is now known as Chargaff’s Rule. Through his research, Chargaff discerned a consistent relationship between the nitrogenous bases present in DNA. Specifically, he noted that the quantity of adenine (A) always equaled the quantity of thymine (T), and similarly, the quantity of cytosine (C) was always equivalent to the quantity of guanine (G).
Mathematically, this relationship can be represented as: A = T and C = G
This discovery was foundational in understanding the pairing mechanism of these bases in the DNA double helix structure. Chargaff’s Rule essentially posits that in any given DNA molecule, the proportion of purine bases (A and G) will always match the proportion of pyrimidine bases (T and C), maintaining the structural integrity and consistency of the DNA across diverse organisms.
Why dna replication is called semiconservative?

- The process of DNA replication is termed “semiconservative” due to the manner in which DNA molecules are duplicated. This concept was elucidated following the groundbreaking discovery of the double helix structure of DNA by James Watson and Francis Crick in 1953. Their revelation suggested a potential mechanism for DNA replication, where the two strands of the double helix separate and each serves as a template for the synthesis of a new complementary strand.
- In semiconservative replication, when the double helix is unwound, each of the two parental strands acts as a template. As new nucleotides are added, they form complementary base pairs with the template strand. Consequently, each newly synthesized DNA molecule consists of one original (parental) strand and one newly formed strand. This ensures that the replicated DNA retains the original genetic information.
- To validate this model of replication, Matthew Meselson and Franklin Stahl conducted a pivotal experiment in 1958. They cultivated the bacterium Escherichia coli in a medium containing a heavy isotope of nitrogen (15N), which became incorporated into the DNA.
- Subsequently, they shifted the bacteria to a medium with a lighter isotope (14N) and observed the DNA after one and two generations using ultracentrifugation. The results displayed distinct bands representing DNA densities.
- After one generation in the 14N medium, the DNA showed an intermediate density, suggesting that it contained a mix of 15N and 14N. After two generations, two bands were evident: one of intermediate density and one corresponding to 14N DNA.
- These observations confirmed that each new DNA molecule consisted of one parental strand and one newly synthesized strand, thereby validating the semiconservative model of DNA replication.
- In essence, the semiconservative nature of DNA replication ensures the preservation and accurate transmission of genetic information from one generation of cells to the next, with each daughter DNA molecule inheriting one strand from the original DNA and one newly synthesized strand.
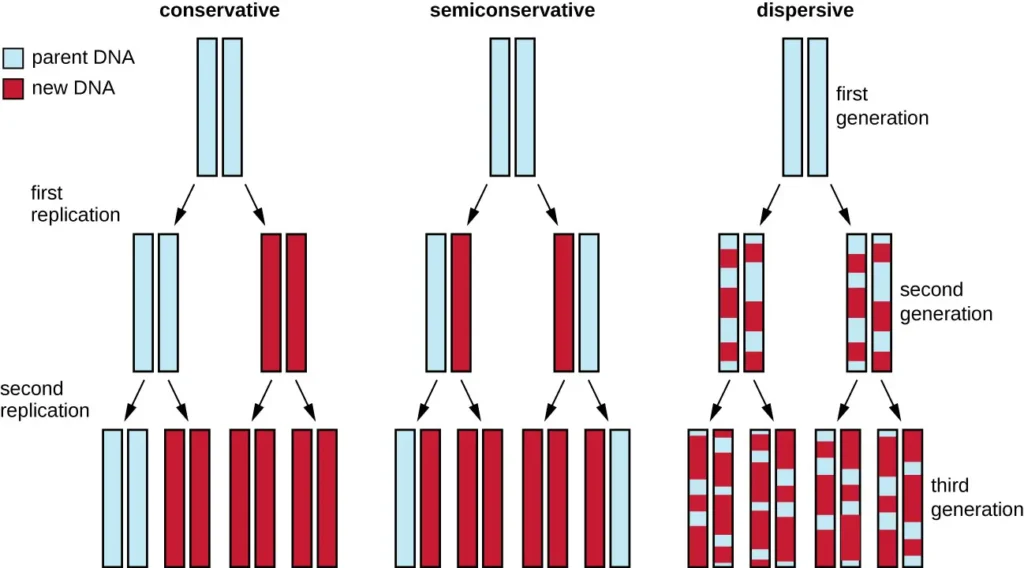
DNA Replication Mind Map
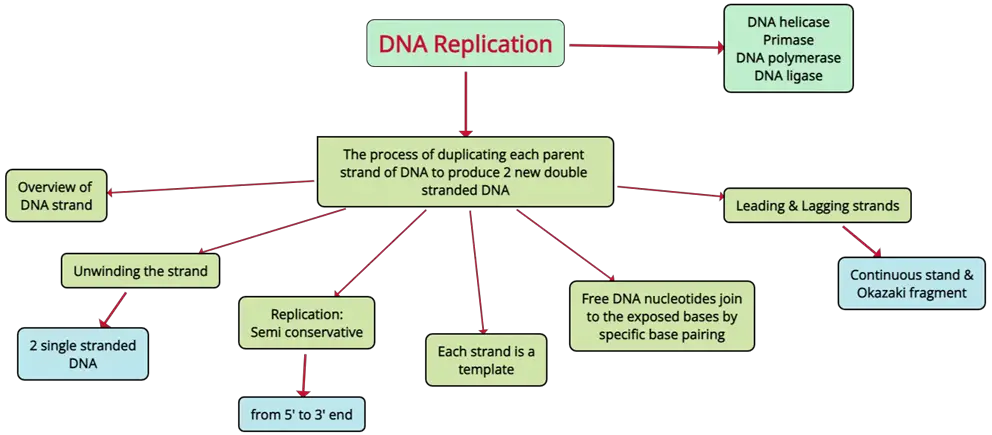
There are several drugs that inhibit DNA synthesis. These drugs can be classified into different categories based on their mechanism of action.
One class of drugs that inhibits DNA synthesis is antimetabolites. These drugs mimic the structure of essential metabolic molecules, such as nucleic acids and proteins, and interfere with their function. Examples of antimetabolites that inhibit DNA synthesis include:
- Methotrexate: This drug inhibits the enzyme dihydrofolate reductase, which is involved in the synthesis of thymidine, one of the building blocks of DNA.
- Azathioprine: This drug inhibits the enzyme inosine monophosphate dehydrogenase, which is involved in the synthesis of guanosine, another building block of DNA.
- Cytarabine: This drug inhibits the enzyme ribonucleotide reductase, which is involved in the synthesis of deoxyribonucleotides, the precursors to DNA.
Other drugs that inhibit DNA synthesis include:
- Cisplatin: This drug forms covalent bonds with DNA, causing DNA strands to cross-link and preventing DNA synthesis.
- Bleomycin: This drug generates reactive oxygen species that damage DNA, leading to DNA strand breaks and inhibiting DNA synthesis.
- Doxorubicin: This drug intercalates into DNA and causes DNA damage, leading to DNA strand breaks and inhibiting DNA synthesis.
It is important to note that these drugs can have serious side effects, and their use is typically limited to specific medical conditions. They should only be used under the supervision of a healthcare professional.
Quiz Practice
DNA Replication Worksheet
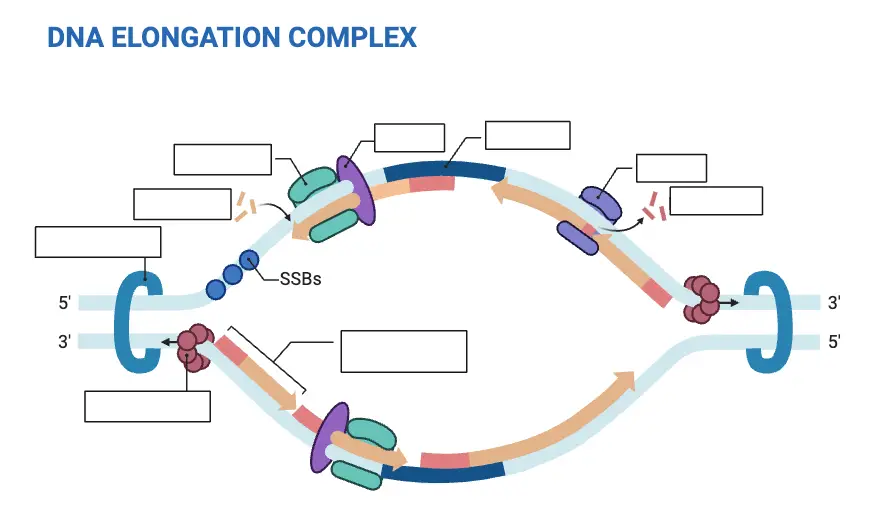
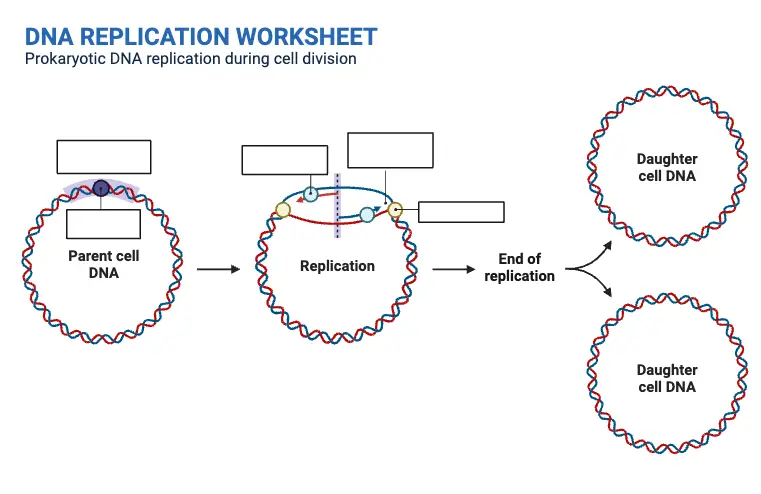
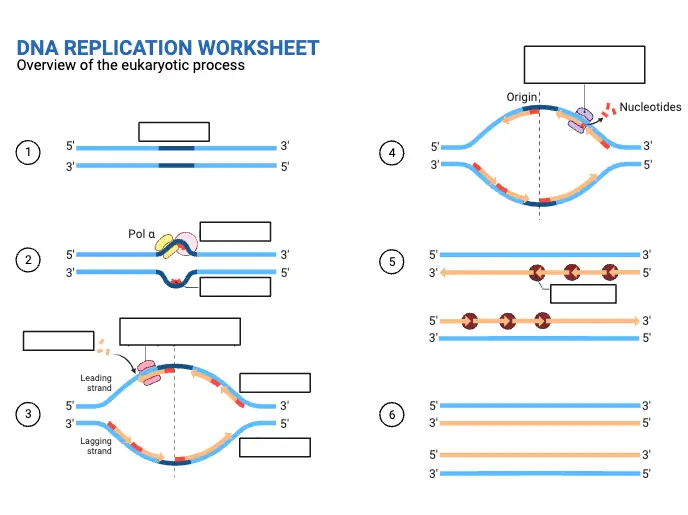
Practice
[mcq_display ids=”65899,65901,65900,65902,65905,65904,65903,65908,65906,65907″]
FAQ
In eukaryotic cells, DNA replication occurs in the nucleus where the chromosomes are located. In prokaryotes, DNA replication occurs in the cytoplasm since they lack a nucleus. Within the nucleus, replication begins at multiple origins of replication, where the DNA strands are unwound, and the replication machinery is assembled to duplicate the genetic material.
DNA replication is called semiconservative because each of the two newly synthesized DNA molecules consists of one original (parental) strand and one newly synthesized strand. This mechanism preserves half of the original DNA in each of the resulting molecules. The term "semiconservative" refers to the conservation of one strand of the original DNA in each new DNA molecule, as opposed to completely conservative replication, where the original molecule is preserved intact.
DNA replication takes place in the nucleus of eukaryotic cells and in the cytoplasm of prokaryotic cells. In eukaryotes, the DNA is organized into chromosomes within the nucleus, and replication begins at specific origins of replication on each chromosome. In prokaryotic cells, the DNA is in the form of a single circular chromosome, and replication starts at the origin of replication within the cytoplasm.
Yes, DNA replication is semiconservative. This means that when DNA replicates, each of the two resulting DNA molecules consists of one original strand (parental) and one newly synthesized strand. This method of replication was confirmed through the Meselson-Stahl experiment, which showed that the original DNA is conserved in each new DNA molecule, making the process semiconservative rather than conservative (where the entire original strand would be preserved) or dispersive (where segments of the original and new DNA would be mixed).
DNA replication is considered semiconservative because each of the two resulting DNA molecules contains one strand from the original DNA and one newly synthesized strand. This ensures that the genetic information is accurately passed on while maintaining a template of the original DNA sequence. This model, confirmed by the Meselson-Stahl experiment, contrasts with other possible replication mechanisms, such as conservative replication, where the entire original strand would be preserved in one molecule and the other would be completely new.
Helicase is an enzyme that plays a critical role in DNA replication by unwinding the DNA double helix. It breaks the hydrogen bonds between complementary base pairs, separating the two DNA strands and creating a replication fork. This allows the DNA to be accessed by the replication machinery for copying. Without helicase, the DNA strands would remain tightly wound and inaccessible for replication.
The function of DNA replication is to duplicate the cell’s genetic material before cell division, ensuring that each daughter cell receives an identical copy of the genome. This is crucial for the maintenance of genetic integrity and the proper function of cells. DNA replication enables organisms to grow, repair damaged tissues, and reproduce by passing on genetic information to the next generation of cells. Without replication, cells would lack the necessary genetic material to function properly.
DNA replication takes place in the S phase of the cell cycle. The S phase follows the G1 phase (growth phase) and precedes the G2 phase (second growth phase). During this phase, the entire genome of the cell is duplicated, ensuring that the genetic material is passed on accurately to the daughter cells.
Replication of DNA occurs during the S phase of the cell cycle. This phase is specifically dedicated to the accurate copying of the cell’s DNA to ensure that both daughter cells receive an identical copy of the genetic material after cell division. The process is tightly regulated to prevent errors or mutations from occurring during replication.
DNA replication occurs during the S phase (Synthesis phase) of the cell cycle, which is between the G1 phase (cell growth) and the G2 phase (preparation for mitosis). This timing ensures that the genetic material is accurately duplicated before the cell enters mitosis or meiosis, which is when the cell divides to produce two daughter cells.
DNA replication takes place during the S phase of the cell cycle. The S phase occurs after the G1 phase, where the cell prepares for replication, and before the G2 phase, where the cell prepares for mitosis. During the S phase, the cell's entire DNA is replicated to ensure each daughter cell receives a complete set of genetic information during division.
DNA polymerase plays a central role in DNA replication by catalyzing the addition of nucleotides to the growing DNA strand. It reads the template strand in the 3' to 5' direction and synthesizes a new complementary strand in the 5' to 3' direction. DNA polymerase also has proofreading abilities, allowing it to detect and correct errors during replication. It works in conjunction with other enzymes, such as helicase, to unwind the DNA and primase to add RNA primers, providing the foundation for replication to proceed.
The result of DNA replication is the production of two identical DNA molecules, each consisting of one old (parental) strand and one newly synthesized (daughter) strand. This process ensures that genetic information is accurately copied and passed on to daughter cells during cell division. As part of the semi-conservative nature of replication, each new DNA molecule is a hybrid, with one strand being from the original DNA molecule and the other newly synthesized.
Helicase is an enzyme responsible for unwinding the DNA double helix during replication. It breaks the hydrogen bonds between the complementary nitrogenous bases, separating the two strands of DNA and creating the replication fork. This unwinding process is essential because it makes the single-stranded DNA templates available for DNA polymerase to synthesize new complementary strands. Without helicase, the DNA strands would remain in their double-helix form, preventing the replication process from proceeding.
DNA polymerase is a key enzyme in DNA replication that catalyzes the addition of nucleotides to the growing DNA strand. It reads the template strand in the 3' to 5' direction and synthesizes a new complementary strand in the 5' to 3' direction. DNA polymerase also has proofreading ability, allowing it to detect and correct errors during replication, enhancing the accuracy of the process. Additionally, it works with primase to extend the RNA primer on the leading and lagging strands during elongation.
The role of DNA polymerase in DNA replication is to synthesize new DNA strands by adding nucleotides that are complementary to the template strand. DNA polymerase reads the template strand in the 3' to 5' direction and assembles a new strand in the 5' to 3' direction. It also has a proofreading function that allows it to correct errors in base pairing, improving the accuracy of the replication process. DNA polymerase works with primase to add nucleotides to the RNA primer and extend the new strand.
The role of DNA helicase in DNA replication is to unwind the DNA double helix at the replication fork. It separates the two strands of the DNA molecule by breaking the hydrogen bonds between complementary base pairs. This unwinding is essential for creating single-stranded regions of DNA that serve as templates for replication. DNA helicase works in conjunction with other proteins, such as single-strand binding proteins (SSBs), to stabilize the separated strands during replication.
DNA replication does not occur during mitosis itself. Instead, it occurs during the S phase of interphase, which precedes mitosis. Mitosis is the process by which the cell's duplicated chromosomes are segregated into two daughter cells. The DNA replication that takes place in the S phase ensures that, by the time mitosis begins, each chromosome has been replicated, and the genetic material is ready for distribution to the daughter cells.
DNA replication is necessary to ensure that when a cell divides, each daughter cell receives an identical copy of the genetic material. This process is crucial for maintaining the stability of an organism's genome, as it prevents the loss of genetic information during cell division. Without DNA replication, the cells would not have enough genetic material to function properly, leading to errors in cell function and potential diseases. Replication is vital for growth, repair, and reproduction.
In eukaryotic cells, DNA replication occurs in the nucleus, where the DNA is located. In prokaryotic cells, which lack a membrane-bound nucleus, replication occurs in the cytoplasm. The process begins at specific locations on the DNA called origins of replication and proceeds bidirectionally.
- Parker, N., Schneegurt, M., Thi Tu, A-H., Lister, P., Forster, B.M. (2019) Microbiology. Openstax. Available at: https://opentextbc.ca/microbiologyopenstax/
- Principles of Biochemistry/Cell Metabolism I: DNA replication. (2017, August 6). Wikibooks, The Free Textbook Project. Retrieved 19:07, October 31, 2019 from https://en.wikibooks.org/w/index.php?title=Principles_of_Biochemistry/Cell_Metabolism_I:_DNA_replication&oldid=3259729.
- Kaiser, G.E. (2015) Prokaryotic Cell Anatomy. Community College of Baltimore County. Available at: http://faculty.ccbcmd.edu/~gkaiser/SoftChalkBIOL230/ProkaryoticCellAnatomy/nucleoid/nucleoid/nucleoid3.html
- The RCSB PDB “Molecule of the Month”: Inspiring a Molecular View of Biology D.S. Goodsell, S. Dutta, C. Zardecki, M. Voigt, H.M. Berman, S.K. Burley (2015) PLoS Biol 13(5): e1002140. doi: 10.1371/journal.pbio.1002140
- Wikipedia contributors. (2020, May 7). Helicase. In Wikipedia, The Free Encyclopedia. Retrieved 13:38, June 9, 2020, from https://en.wikipedia.org/w/index.php?title=Helicase&oldid=955303097
- Windgassen, T.A., Wessel, S.R., Bhattacharyya, B., and Keck, J.L. (2017) Mechanisms of bacterial DNA replication restart. Nuc Acids Res 46(2):504-519. Available at: https://www.ncbi.nlm.nih.gov/pmc/articles/PMC5778457/
- Xu, Z-Q., Dixon, N.E. (2018) Bacterial Replisomes. Curr Opin Struct Biol 53:159-168. Available at: https://www.sciencedirect.com/science/article/pii/S0959440X18300952
- Liu, B., Eliason, W.K., and Steitz, T.A. (2013) Structure of a helicase-helicase loader complex reveals insights into the mechanism of bacterial primosome assembly. Nature Comm 4:2495. Available at: https://www.researchgate.net/publication/256764134_Structure_of_a_helicase-helicase_loader_complex_reveals_insights_into_the_mechanism_of_bacterial_primosome_assembly
- Xu, Z-Q., and Dixon, N.E. (2018) Bacterial Replisomes. Curr Op Struc Biol 53:159-168. Available at: https://www.sciencedirect.com/science/article/pii/S0959440X18300952
- Fernandez-Leiro, R., Conrad, J., Scheres, S.HW., and Lamers, M.H. (2015) cryo-EM structures of the E. coli replicative DNA polymerase reveal its dynamic interactions with the DNA sliding clamp, exonuclease and τ. eLife 4:e11134. Available at: https://elifesciences.org/articles/11134
- Ekundayo, B. and Bleichert, F. (2019) Origins of DNA replication. PLOS 15(12): e1008556. Available at: https://journals.plos.org/plosgenetics/article?id=10.1371/journal.pgen.1008320
- Leman, A.R., and Noguchi, E. (2013) The replication fork: understanding the eukaryotic replication machinery and the challenges to genome duplication. Genes 4(1): 1-32. Available at: https://www.ncbi.nlm.nih.gov/pmc/articles/PMC3627427/
- Doublié, S. and Zahn, K.E. (2014) Structural insights into eukaryotic DNA replication. Front. Microbiol. 5:444. Available at: https://www.frontiersin.org/articles/10.3389/fmicb.2014.00444/full
- Billard, P., and Poncet D.A. (2019) Replication stress at telomeric and mitochondrial DNA: Common origins and consequences on ageing. Int J. Mol Sci 20(19):4959. Available at: https://www.mdpi.com/1422-0067/20/19/4959/htm
- Yeh, J-K., and Wang, C-Y. (2016) Telomeres and telomerase in cardiovascular diseases. Genes 7(9)58. Available at: https://www.mdpi.com/2073-4425/7/9/58/htm
- Boccardi, V. and Herbig, U. (2012) Telomerse gene therapy: a novel approach to combat aging. EMBO Mol Med 4:685-687. Available at: https://www.embopress.org/doi/epdf/10.1002/emmm.201200246
- Wikipedia contributors. (2020, April 26). Cyclin-dependent kinase. In Wikipedia, The Free Encyclopedia. Retrieved 18:52, June 30, 2020, from https://en.wikipedia.org/w/index.php?title=Cyclin-dependent_kinase&oldid=953307433
- Falkenberg, M. (2018) Mitochondrial DNA replication in mammalian cells: overview of the pathway. Essays Biochem 62(3):287-296. Available at: https://www.ncbi.nlm.nih.gov/pmc/articles/PMC6056714/
- Folwer, S., et. al. (2013) Concepts of Biology. Openstax. Available at: https://openstax.org/details/books/concepts-biology?Bookdetails
- Aleem, E. and Arceci, R.J. (2015) Targeting cell cycle regulators in hematologic malignancies. Frontiers in Cell and Developmental Biology 3(16). Available at: https://www.researchgate.net/publication/275354547_Targeting_cell_cycle_regulators_in_hematologic_malignancies
- https://www.khanacademy.org/science/biology/dna-as-the-genetic-material/dna-replication/a/dna-proofreading-and-repair
- https://www.davuniversity.org/images/files/study-material/DNA%20proofreading,EDU161.pdf
- https://en.wikipedia.org/wiki/Proofreading_(biology)
- https://geneticeducation.co.in/how-dna-proofreading-occurs-during-replication-4-ways-it-happens/
- https://www.expii.com/t/dna-proofreading-definition-role-in-replication-10208