What is DNA Recombination?
The main thing that happens in recombination is the exchange of genetic information.
- Genetic recombination is the process of changing the order of genetic information within and between DNA molecules. This can happen in a number of ways.
- Now, scientists are looking into how DNA rearrangements can be used to change the genomes of a growing number of organisms.
- At least three main types of genetic recombination events can be found. Homologous genetic recombination, also called “general recombination,” is the exchange of genes between any two DNA molecules (or parts of the same molecule) that share a long stretch of nearly identical sequence. It doesn’t matter what order the bases are in as long as they are in the same order in both DNAs.
- In site-specific recombination, exchanges only happen at a certain sequence of DNA.
- DNA transposition is different from the other two types because it involves a short piece of DNA that can move from one place in a chromosome to another.
- Barbara McClintock was the first person to notice these “jumping genes” in maize in the 1940s.
- There are also a lot of strange changes to genes for which no mechanism or reason has yet been suggested. Here, we’ll talk about the three main types.
- Genetic recombination systems have as many different functions as they do ways of working.
- They have roles in specialised DNA repair systems, specialised activities in DNA replication, regulating the expression of certain genes, helping the correct separation of chromosomes during the division of eukaryotic cells, keeping genetic diversity, and carrying out programmed genetic rearrangements during embryonic development.
- Most of the time, genetic recombination is closely linked to other processes in DNA metabolism, and this will be a big part of what we talk about.
Types of DNA Recombination
Recombination is mainly of four types:
- Homologous recombination –happens when a piece of a chromosome is swapped between two homologous chromosomes. This happens during meiosis.
- Non-homologous recombination – exchanging genetic information between chromosomes that are not the same.
- Site-specific recombination – With the help of restriction enzymes, DNA is cut at a specific site and a new piece of DNA, like plasmid, bacteriophage, etc., is added.
- Replicative recombination – For example, a new copy of a transposable element can be made at a different spot on the same chromosome.
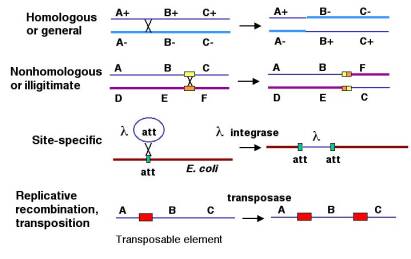
There are two other kinds of recombination that are used in recombinant DNA technology. It is well known that restriction endonucleases and DNA ligases can cut and join different DNA molecules in the lab. Once they are made, these recombinant DNA molecules are put into a host organism, which is usually a bacterium. If the recombined DNA is a plasmid, phage, or some other type of molecule that can copy itself in the host, it will stay outside of the chromosomes.
But the recombinant DNA molecule can be put into a host where it can’t copy itself, such as a plant, a cultured animal cell, or a fertilised mouse egg. For the host to be changed in a stable way, the DNA must be taken up by a host chromosome. This can happen quite often through homologous recombination in bacteria and yeast.
But neither plant cells nor animal cells do this. On the other hand, some of these introduced DNA molecules are sometimes added to the chromosomes of the host cell in random places. So, random recombination in chromosomes can make stable transfected cells as well as plants and animals that have been genetically changed. Even though it is used a lot in the lab, no one really knows how this recombination happens during transformation or transfection.
1. Homologous recombination
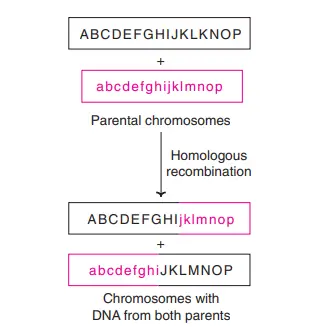
- This is also called “general recombination,” and it happens between chromosomes that are the same or almost the same (DNA sequences).
- This process is also called “general recombination.” It is a natural part of eukaryotes, bacteria, and viruses. It is very useful in techniques for changing genes.
- The best example is the mixing of chromosomes from the father and the mother.
- It is known that chromosomes do not stay the same from one generation to the next. They are instead passed down from both parents.
- Because of homologous recombination, this is possible. There have been three ideas about how homologous recombinations happen.
- Holliday model
- Meselson-Radding model
- Double-strand break model.
Holliday model
- Robin Holliday devised a model in 1964 that accounted for the creation of heteroduplexes and gene conversion during recombination.
- Although it has been replaced by the double-strand break model (at least for recombination in yeast and higher organisms), it is still a good starting point.
- It depicts the essential processes of pairing of homologous duplexes, development of a heteroduplex, construction of the recombination joint, branch migration, and resolution.
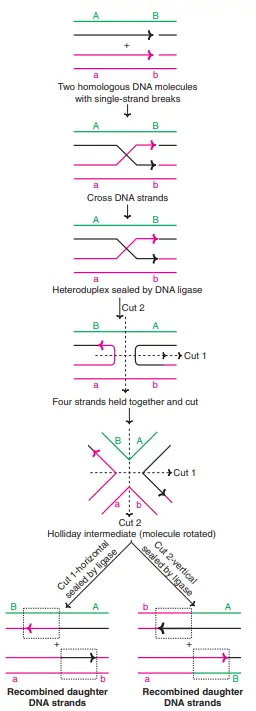
Steps of Holliday model
- Two homologous chromosomes, each made of duplex DNA, are paired with neighbouring comparable sequences.
- An endonuclease nicks homologous areas of the paired duplexes’ homologous strands. This is depicted for the strands to the right of the arrow in the illustration.
- The nicked ends detach from their complementary strands, and each single strand invades the opposing duplex. This occurs in a reciprocal fashion to generate a heteroduplex area consisting of one strand from each parental duplex.
- The nicks are sealed by DNA ligase. One strand of each parental duplex crosses over into the other parental duplex, yielding a stable joint molecule. The name for this X-shaped joint is a Holliday intermediate or Chi structure.
- Branch migration then enlarges the heteroduplex area. The stable joint can move along the paired duplexes, allowing more of each invading strand to enter and expanding the heteroduplex zone.
- The intermediate of recombination is then eliminated by nicking a strand from each duplex and ligation.
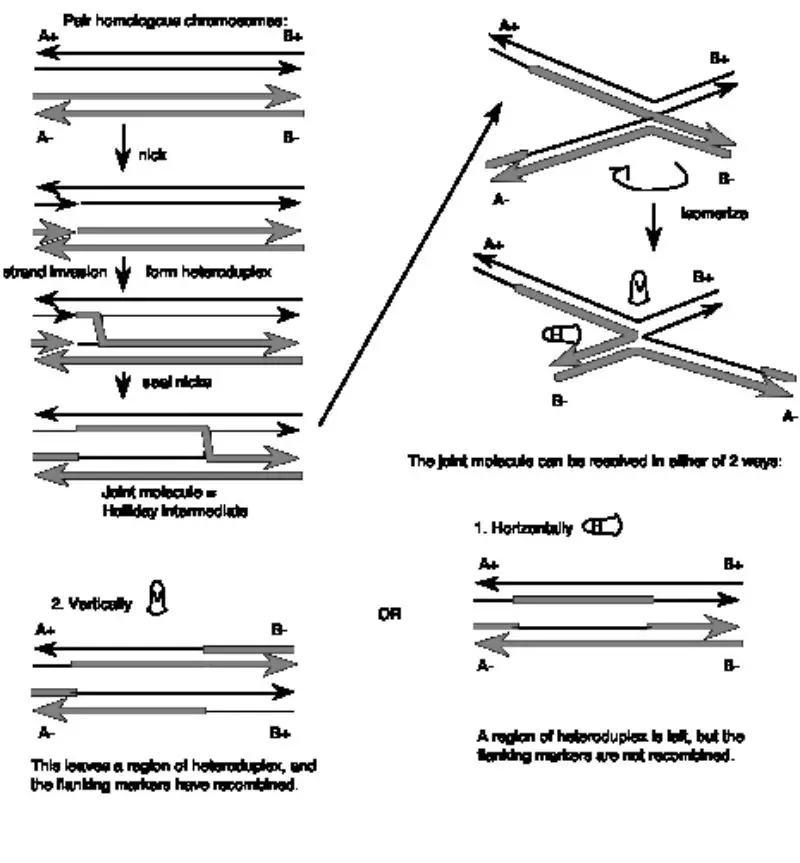
Only one of the two methods of resolution results in the exchange of flanking markers following recombination. The two forms of resolution can be depicted by rotating the duplexes so that none of the strands cross in the figure. In the “horizontal” method of resolution, the identical DNA strands that were initially nicked in the parental duplexes are nicked again.
This results in the formation of two duplex molecules with a patch of heteroduplex, but no flanking area recombination. In contrast, in the “vertical” method of resolution, the nicks are made in the other strands, i.e. those strands that were not nicked in the original parental duplexes. In addition to leaving a patch of heteroduplex, ligation of these two ends causes flanking regions to recombine.
Note that “horizontal” and “vertical” are merely convenient identifiers for the two modes based on our ability to create two-dimensional graphics. Whether the resolution steps target the originally cleaved strands versus the opposite strand is a crucial differential in terms of genetic fate. Visit http://www.wisc.edu/genetics/Holliday/index.html to see this model of general recombination in dynamic form. This video depicts the steps of the Holliday model, displaying the motions more vividly than schematics.
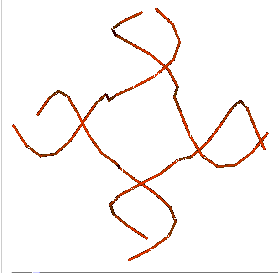
About Above Image: A look at a Holliday intersection. A. An electron micrograph of two DNA double strands in the middle of recombination. B. Holliday junction from X-ray crystallography of a RuvA-Holliday junction complex (from Hargreaves et al., 1998, Nature Structural Biology 5, pp. 441-460). For this view, the RuvA protein tetramer was taken away, and only the phosphodiester backbones of the two duplexes (four strands) are shown. Notice how the DNA in the middle of the structure is twisted. In B form DNA, these are the same as about three nucleotides on each strand that are not paired. The atomic coordinates were downloaded from the Molecular Structure database at NCBI and drawn in Cn3D v.3.0. Each nucleotide in each of the four strands is marked with a letter and a number to show where it is in the chain. On the website for the course, you can find the files you need to see the 3-D image on your own computer.
The recombinant junction proposed by Holliday has been observed in electron micrographs of DNA duplexes undergoing recombination. The proposed X form is present. This location would be ideal for an EM photo. Although this junction is depicted with a space between the duplexes in drawings, the duplexes are actually juxtaposed, and only a small number of base pairs are broken in the Holliday intermediate. The choice between “horizontal” and “vertical” resolution is presumably a random occurrence by the resolving nuclease given the symmetry of the structure. It selects two strands, but is unable to distinguish between those that were first cleaved and those that were not.
Studies of recombination between chromosomes with little similarity have revealed that the minimum length of the region required to connect the recombining duplexes is approximately 75 bp. If the homology area is shorter than this, recombination occurs at a much lower rate.
The heteroduplex patch can be duplicated or repaired to induce a gene conversion event (Figure 8.8). As demonstrated in Figure 8.8, replication across the horizontal resolution products (from Figure 8.6) will yield a duplex from each strand of the heteroduplex. If we consider the parental chromosomes to be A+C+B+ and A-C-B-, and the heteroduplex to be in gene C, then the products of replication can have the parental C+ transformed to a C- while still flanked by A+ and B+, or the parental C- converted to a C+ while still flanked by A- and B-. In both instances, gene C has transformed into a new allele without changing the flanking markers.
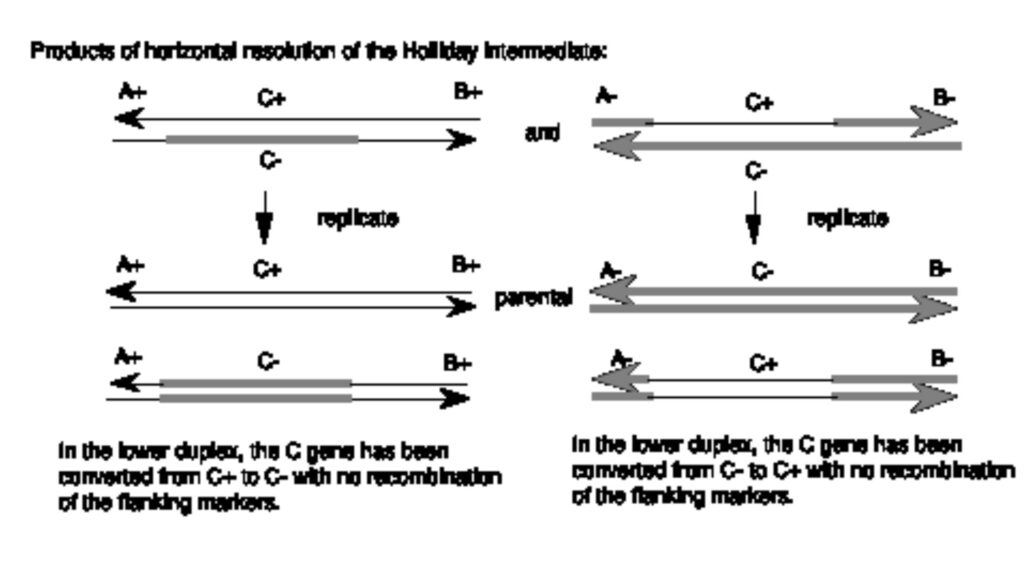
Although the original Holliday model accounted for many essential characteristics of recombination (everything known at the time), some new information necessitates modifications to the model. The Holliday model, for instance, treats both duplexes equally; both both the invader and the target of the strand invasion.
In addition, the Holliday model necessitates no DNA synthesis. However, additional research revealed that one of the duplex molecules is favoured as the genetic information giver. Consequently, additional models, such as one by Meselson and Radding, incorporated fresh DNA synthesis at the point of the nick and degradation of a strand of the other duplex to induce asymmetry in the two duplexes, with one duplex serving as the DNA donor and the other as the DNA recipient. These and other concepts have been combined into a novel model of recombination utilising DNA double-strand breaks.
Meselson-Radding model
- The Meselson–Radding model is a recombination model that permits hybrid DNA to develop on only one chromatid.
- It was developed in reaction to the discovery from Saccharomyces cerevisiae that a significant proportion of gene conversion is asymmetrical, or restricted to one chromatid.
- On a single chromatid, heteroduplex DNA (hybrid DNA containing one or more mismatched base pairs) has occurred.
- The model generates an unequal distribution of heteroduplex by initiating recombination on only one of the two DNA molecules involved. As illustrated in Figure 1 (a).
- The 3′ hydroxyl at the nick then initiates DNA synthesis, which displaces the same DNA strand while a new strand forms.
- As seen in Figure 1, the 5′ single-stranded tail formed by this reaction invades a homologous molecule by forming a D-loop (b).
- The D-loop continues to expand as a result of DNA polymerase’s production of a new strand and displacement of the old strand. Eventually, this polymerization will halt.
- The structure depicted in Figure 1 is the result of endo- and exonucleolytic degradation of the open portion of the D-loop (c).
- At this stage, there is a stretch of asymmetrical hybrid DNA between the site of recombination initiation and the location where DNA synthesis has ceased.
- It is hypothesised that the structure depicted in Figure 1(c) will transform into a Holliday junction upon isomerization.
- Figure 1 illustrates the product of isomerization (d). As in Holliday’s concept, the Holliday junction can now migrate, allowing the propagation of symmetrical hybrid DNA.
- Consequently, the structure depicted in Figure 1(e) has a length of asymmetrical hybrid DNA close to the initiation site and lengths of symmetrical hybrid DNA on the predicted side of the Holliday junction.
- Wherever allelic differences exist between the two parental molecules, mismatched base pairs will exist in the hybrid molecule.
- As with other recombination models based on the production of heteroduplex DNA, these mismatched base pairs are corrected by a mismatch repair system that excises one or the other strand along a length that includes the mismatch.
- The conversion and postmeiotic segregation patterns found in recombination data can be generated by repairing the excision gap by replicating the remaining strand.
- The Holliday junction depicted in Figure 1(e) is vulnerable to rapid isomerization, therefore both the structures depicted in Figures 1(e) and 1(f) are equally likely to occur.
- If an endonuclease (resolvase) cuts the crossed strands in the structure seen in Figure 1(e), it will, upon ligation, produce two molecules with a reciprocal crossing.
- Figure 1(f) depicts that endonucleolytic cleavage of the crossed strands in the other structure will result in a noncrossover recombination event.
- Consequently, according to this model, crossover and noncrossover products will be equally prevalent and are predicted to exhibit the same conversion and postmeiotic segregation patterns.
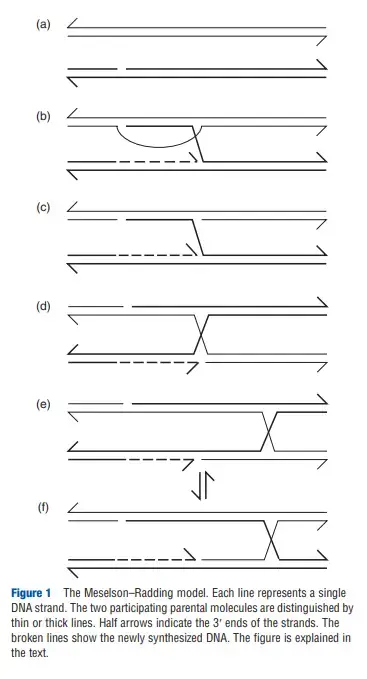
The Double Strand Gap Repair Model
- Multiple lines of evidence, especially from investigations of recombination in yeast, suggested that the exchange of DNA chains initiated at single-strand nicks must be modified.
- In contrast to the equal exchange envisaged by the original Holliday model, one DNA duplex tended to be the donor of information, while the other was the recipient.
- Also in yeast, double-strand breaks can induce recombination. In mating type switching in yeast, both DNA strands are broken (by the HO endonuclease) to induce recombination between the MAT and HML(R) loci.
- Using yeast-transformed plasmids, it was demonstrated that a double-strand gap in the “aggressor” duplex can be utilised to trigger recombination, with the gap being repaired during recombination.
- In this instance, the gap in one duplex was filled by DNA from the second substrate. In 1983, Jack Szostak and colleagues introduced a significant new model for recombination that encompassed all of this evidence.
- The model is known as the double-strand-break model. In contrast to the Holliday model, this model includes novel aspects such as initiation at double-strand breaks, nuclease digestion of the aggressor duplex, novo synthesis, and gap repair.
- Nonetheless, the core Holliday junction, branch migration, and resolution are preserved, albeit with a bit more complexity due to the increased number of Holliday junctions.
- Although numerous facets of the recombination mechanism are dissimilar
Steps of The Double Strand Gap Repair Model
- The diagrams below show the steps of the double-strand-break model up to the point where the joint molecules form;
- The thin blue lines show how an endonuclease cuts both strands of one of the homologous DNA duplexes. This duplex is the aggressor because it starts the recombination process. As we go through the model, it will become clear that it also gets genetic information.
- An exonuclease widens the cut to make a gap with 3′ single-stranded ends on the strands.
- One of the free 3′ ends moves into a similar area on the other duplex, which is shown as thick red lines. This area is called the donor duplex. When heteroduplex forms, it also makes a D-loop, also called a displacement loop, in which one of the strands of the donor duplex is moved.
- The invading 3′ end starts repair synthesis, which makes the D-loop longer. The D-loop grows until it can cover the whole gap on the aggressor duplex, which is the one that the endonuclease first cut. The DNA from the invaded DNA duplex (the thick red line) is used as a template for the new DNA, so the new DNA has the same sequence as the invaded DNA.
- When the red strand from the donor moves to the other side of the gap on the thin blue recipient, it will join with the other 3′ single-stranded end at that end of the gap. Now, the strand that was moved has filled the hole on the aggressor duplex by giving its sequence to the duplex that was broken in the first place. DNA polymerase helps repair synthesis change the donor D-loop into duplex DNA. During steps 4 and 5, the duplex that was first invaded serves as the donor duplex. This means that it gives genetic information during this phase of repair synthesis. On the other hand, genetic information is given to the aggressor duplex. Note that the single strand invasion models predict the opposite, which is that the first strand to invade is the one that gives away its genetic information.
- DNA ligase will close the nicks, one on the left side of Fig. 8.9 and the other on the right. Even though it is between a strand on the bottom duplex and a strand on the top duplex, it is the same as the ligation in the first nick (the apparent physical separation is an artefact of the drawing). In both cases, closing the nick makes what is called a Holliday junction.
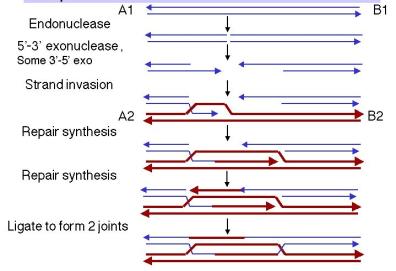
At this point, there are two recombinant joints in the recombination intermediate (Holliday junctions). The invaded duplex gave DNA to the aggressor duplex to fill in the hole that was there before. Heteroduplex is now on both sides of the filled gap. One heteroduplex is to the left of the filled gap on the aggressor duplex, and the other is to the right of the filled gap on the donor duplex. From each Holliday junction, the areas of heteroduplex can be made bigger by branch migration.
Now, the recombination intermediate can be gotten rid of. The fact that there are two recombination joints makes the process a little more complicated, but it is still mostly the same as what was talked about for the Holliday model. Each joint can be fixed in either the horizontal or vertical direction. The most important thing is whether or not the joints are solved in the same way (both horizontally or both vertically) or in different ways.
If both joints are resolved in the same way, the original duplexes will be released, each with a region of changed genetic information that is a “footprint” of the exchange event. This area of changed information is the original gap, plus or minus the areas where branch migration happened. For example, if both joints are fixed by cutting the strands that were originally split (“horizontally” in our Holliday model diagram), then there is no crossover at either joint. If you can fix both joints by cutting the strands that weren’t cut the first time (“vertically” in our Holliday model diagram), you have a crossover at both joints. Because this double crossover is so close together, there will be no recombination of flanking markers.
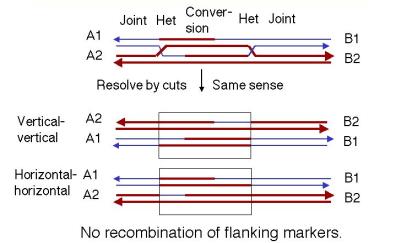
If, on the other hand, each joint is resolved in a different direction, flanking markers will recombine. That is, one joint won’t let a crossover happen, but the other will.
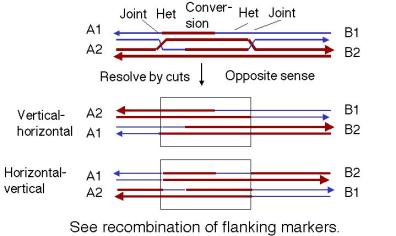
The single-strand nick model that Holliday first came up with is different from the double-strand break model in several ways. In the double-strand-break model, the sequence of the donor duplex is now in the part of both molecules that used to be the gap. At each end, one heteroduplex is connected to each duplex. So, heteroduplex is set up in an asymmetric way, which means that each duplex molecule has a different heteroduplex. Part of the sequence of one duplex molecule has been added to the other (the recipient, initiating duplex has been converted to the sequence of the donor). In the single-strand invasion model, each DNA duplex has symmetric heteroduplex material covering the area from the exchange site to the branch that is moving. In versions of the Meselson-Radding model in which some DNA is broken down and made again, the genetic information comes from the chromosome that starts the process.
There are also many important things that these models have in common. All of the models have steps in common, such as making a single strand of DNA at one end, looking for homology, strand invasion or strand exchange to make a single molecule, branch migration, and resolution. We have found and studied the enzymes that speed up each of these steps.
Recombination Models and Segregation Patterns
The models should be applied to the four chromatid stage of the meiotic cell. Remember that there are two chromosomes containing red DNA and two chromosomes containing green DNA in the region of interest. As depicted in the models, we consider the exchange between a red DNA duplex and a green DNA duplex and derive the effects of the exchange on the segregation pattern.
- Holliday model———-> Symmetric heteroduplex ———> Two colonies with half-sectors in yeast by germination of the four spores from a meiotic event; two pairs of non-identical sister spores in Ascobolus. 4: 4 aberrant segregation.
- Meselson-Radding model———-> Asymmetric heteroduplex———-> One sectored colony in yeast; one pair of non-identical sister spores in Ascobolus. 5:3 aberrant segregation.
- Double strand gap repair model———-> Conversion of green DNA to red DNA in the region of repair. 6:2 aberrant segregation.
Homologous Recombination in Eukaryotes
DNA Repair
- Homologous recombination is essential for mending DNA damage.
- During mitosis, DNA strands that have been damaged by ionising radiation or chemicals undergo recombination to overcome the damage.
- If broken DNA is not repaired, it can amplify and produce mutations in the genome.
Genetic Diversity
- The sex cells go through meiosis to make ovum and sperm, which are specialised cells.
- During meiosis, there is an exchange of genetic material between homologous chromosomes. This is called chromosomal crossover.
- Every time an organism reproduces, this process of recombination creates a new set of genes.
Homologous Recombination in Bacteria
Horizontal Gene Transfer
- Horizontal gene transfer is when DNA from one organism moves to another that it is not related to.
- The incoming DNA is very similar to the DNA of the receiver, and the two go through a process called homologous recombination to add the foreign DNA.
- Transformation is when DNA from one organism of the same species moves to another organism of the same species.
- They use a process called homologous recombination to take in the donor DNA. They do this by binding to a few proteins, which makes the cells ready to take in the DNA.
- Like in eukaryotes, homologous recombination helps bacteria fix their DNA and makes their genes more diverse.
Homologous Recombination in Viruses
- Homologous recombination can happen in both the DNA and RNA strands of a virus.
- When two different viral genomes with harmful mutations come together to make a fully functional genome, this helps viral evolution.
- Homologous recombination is also a main way that human herpesvirus 6 gets into human telomeres, which causes rashes, fever, and diarrhoea in babies and young children.
- Covid-19, which is caused by the SARS-Cov-2 virus, is also a result of homologous recombination, a way that viruses change over time.
Homologous Recombination and Genetic Engineering
- Genetic engineering uses homologous recombination to get rid of faulty or mutated genes. When the mutated genes are put into a cell, the cell finds a similar sequence in the foreign gene, goes through recombination, knocks out the bad sequence, and copies itself to make a functional gene. It can also be used to target genes in knockout mice models, where you can remove any sequences you want.
Disadvantages of Homologous Recombination
- When recombination is done wrong, it can bring out many problems in a genome. During the process of making sperm and eggs, chromosomes can line up wrong, leading to too many or too few chromosomes in the sperm and eggs.
- Failure of homologous recombination leads to Down’s syndrome, which is caused by a change in chromosome 21.
- Chromosomal recombination going wrong is also linked to cancer.
Applications of Homologous Recombination
- Gene targeting, which is shown in models of knockout mice, is a way to make genetically modified organisms with recombinant DNA.
- Protein engineering means putting together two genes that code for different proteins to make a new protein. It changes the way the protein sequence looks.
- It can also help treat cancer by repairing the damage to the DNA.
2. Non-homologous recombination
- This is called “illegal recombination,” and it doesn’t need any special homologous sequences to happen.
- Nonhomologous recombination is shown by the process of transposition.
- Another example is when outside genes are randomly added to the chromosomes of mammals.
- Non-homologous recombination is the process of recombining genes when there are no homologous DNA sequences.
Transposition
- Transposition is mostly about moving certain pieces of DNA around in the genome.
- Transposons or transposable elements are the pieces of DNA that can move around.
- Barbara McClintock found them in maize in 1950, but other workers didn’t see what was important about them for about 20 years.
- Transposons can move around in the target chromosome and go almost anywhere.
- There are two ways to change something. One uses an RNA intermediate, and the other doesn’t.
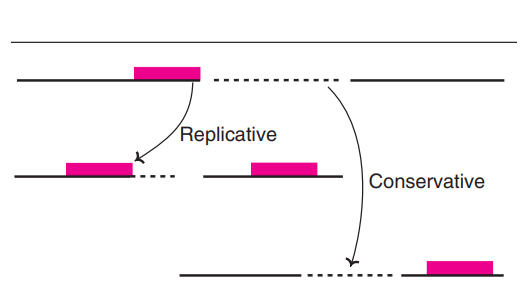
Retrotransposition
- Retrotransposition is a type of gene transfer that involves an RNA intermediate.
- A copy of RNA was made from a transposon through the normal process of transcription (also called as retrotransposon).
- The RNA is then copied into DNA by the enzyme reverse transcriptase.
- The copy of the transposon that was made from the new DNA is added to the genome.
- This integration can happen randomly on the same chromosome or on a different one.
- Because of the retrotransposition, the transposon is now found at two different places on the genome.
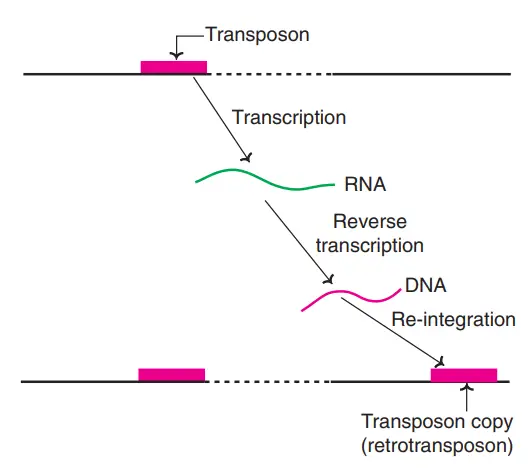
DNA transposition
- Some transposons can move DNA directly from one piece of DNA to another piece of DNA.
- This can happen through either conservative or replicative transposition.
- Both of these processes need enzymes, which are mostly written by the genes in transposons.
- In eukaryotes, DNA transposition happens less often than retrotransposition. But DNA transposons are more important for prokaryotes than RNA transposons.
Significance of transposition
- Transposons have changed a lot of the human genome over time. This is now a widely accepted fact.
- Short interspersed elements, or SINEs, are repeats of DNA sequences. There are about 500,000 copies of each SINE in a haploid human genome, for example. Alu sequences.
- Long interspersed elements (LINEs) are also repeated DNA sequences. About 50,000 copies of LINEs are found in the human genome. L1 elements.
- Some diseases that are caused by mutations are caused by transposons that get into genes.
References
- (2008). Meselson-Radding Model Of Recombination (1975 Proc Natl Acad Sci USA 72:358). In: Encyclopedia of Genetics, Genomics, Proteomics and Informatics. Springer, Dordrecht. https://doi.org/10.1007/978-1-4020-6754-9_10182
- Hastings, P. J. (2013). Meselson–Radding Model. Brenner’s Encyclopedia of Genetics, 364–365. doi:10.1016/b978-0-12-374984-0.00927-x
- Gibb, Bryan. (2010). Requirements for Catalysis in Cre Recombinase.
- https://www.slideshare.net/rahulmanjunath2/site-specific-recombination
- https://m.blog.naver.com/pcshgu98/221670950029
- https://www.web-books.com/MoBio/Free/Ch8D7.htm
- https://www.slideshare.net/RoshanParihar2/site-specific-recombination-124785829
- https://www.bx.psu.edu/~ross/workmg/RecombineDNACh8.htm
- https://sites.cns.utexas.edu/sites/default/files/bio-366/files/bio-366-web-horecom-models-sep4-2018.pdf?m=1536084235
- https://microbiology.ucdavis.edu/heyer/wordpress/research/homologous-recombination
- https://www.creative-diagnostics.com/non-homologous-end-joining-pathway.htm
- http://genesdev.cshlp.org/content/19/22/2715.short
- https://www.cureffi.org/2014/10/06/molecular-biology-13/
- https://en.wikipedia.org/wiki/Non-homologous_end_joining
- http://www.sbs.utexas.edu/jayaram/Bio-366/BIO-366-Homologous%20Recombination/BIO-366-Recombination%20Models.pdf