What is Cytoplasmic or Extra-Nuclear Inheritance?
Cytoplasmic inheritance, also known as extrachromosomal or extranuclear inheritance, refers to the transmission of genetic material that is not found within the nucleus but rather in the cytoplasm of a cell. This type of inheritance contrasts with Mendelian inheritance, which involves nuclear genes. In cytoplasmic inheritance, the genetic information is typically carried in organelles such as mitochondria or chloroplasts. Since these organelles contain their own DNA, the traits they govern are passed from one generation to the next through the cytoplasm of the cell, often following a maternal pattern of inheritance.
During cell division, nuclear chromosomes divide with precision, ensuring accurate genetic distribution between daughter cells. However, the cytoplasm, which houses organelles like mitochondria and chloroplasts, does not follow the same strict segregation. This results in the female gamete contributing the majority of the cytoplasm to the zygote, and thus, the traits inherited through cytoplasmic factors often come from the mother. For example, if two strains of an organism with different cytoplasmic types are crossed reciprocally, the resulting offspring may show different traits depending on the cytoplasm they inherit. This maternal effect leads to offspring resembling the cytoplasmic characteristics of the mother rather than the nuclear genotype.
The first documented case of cytoplasmic inheritance was reported by Carl Correns in Mirabilis jalapa in 1909, where leaf color followed a non-Mendelian pattern. Similarly, other researchers such as Baur and Jenkins contributed to our understanding of cytoplasmic inheritance in plants like Pelargonium zonale and maize, respectively. For instance, Jenkins described variegation in maize leaves, and Rhoades later studied cytoplasmic male sterility (CMS) in maize, which became a pivotal example of this form of inheritance.
In addition to plants, cytoplasmic inheritance has been observed in other organisms. For example, Sonneborn discovered kappa particles in Paramecium in 1943, which demonstrated cytoplasmic transmission of these particles from one generation to the next. Furthermore, advancements in molecular biology confirmed that organelles like chloroplasts and mitochondria contain their own DNA. Ris and Plaut demonstrated the presence of DNA in chloroplasts in 1962, while Nass and colleagues proved the existence of mitochondrial DNA in 1963. These findings solidified the role of cytoplasmic inheritance in governing certain traits.
One notable example of cytoplasmic inheritance in microorganisms is Ruth Sager’s work in 1954, where she described the cytoplasmic inheritance of streptomycin resistance in Chlamydomonas. This research highlighted the fact that not all inheritance is governed by nuclear genes, and some traits can be passed through cytoplasmic factors, further expanding the understanding of how genetic material is transmitted across generations.
Characteristics of Cytoplasmic Inheritance
The characteristic features of cytoplasmic inheritance distinguish it from typical Mendelian inheritance, emphasizing the unique role of organelle-based genes. Below are the primary features that define this form of inheritance:
- Reciprocal Difference:
Reciprocal crosses involving traits governed by cytoplasmic genes, or plasmagenes, often result in marked differences. Typically, plasmagenes are transmitted from the female parent only, making cytoplasmic inheritance a form of uniparental inheritance. This contrasts with nuclear inheritance, where genes from both parents are equally involved. - Lack of Segregation:
Unlike nuclear genes that exhibit segregation in F1, F2, and subsequent generations, traits governed by cytoplasmic inheritance do not show such segregation. Since plasmagenes are generally passed down from the female parent alone, the offspring consistently inherit these genes without recombination or segregation. - Somatic Segregation:
Plasmagenes often display their effects in somatic tissues, such as in cases of leaf variegation. This type of somatic segregation is uncommon in nuclear genes, highlighting the distinct behavior of cytoplasmic inheritance in influencing traits at the cellular level. - Association with Organelle DNA:
Many plasmagenes are closely associated with the DNA found in organelles such as chloroplasts and mitochondria. For example, cytoplasmic male sterility (CMS) in crops like sorghum and maize is linked to mitochondrial DNA. These associations indicate the critical role of organelle genomes in certain traits. - Nuclear Transplantation:
In cases where nuclear transplantation (the removal and replacement of the nucleus) reveals that a trait is controlled by the cytoplasmic genotype rather than the nuclear genotype, it serves as clear evidence of cytoplasmic inheritance. This process further illustrates the role of the cytoplasm in governing certain traits. - Mutagenesis:
Specific mutagens, such as ethidium bromide, are known to act exclusively on plasmagenes without affecting nuclear genes. When mutations are induced by these agents, it indicates that the affected genes are located in the cytoplasm, providing another way to identify cytoplasmic inheritance. - Lack of Chromosomal Location:
In organisms with well-developed linkage maps for nuclear genes, any gene that cannot be mapped to these linkage groups is unlikely to be a nuclear gene. This absence of chromosomal location further supports the idea that the gene is a plasmagene. - Transfer of Nuclear Genome Through Backcrossing:
The nuclear genome of one variety or species can be transferred into the cytoplasm of another through repeated backcrossing. This technique produces what are known as alloplasmic lines, where the cytoplasm originates from one species and the nuclear genome from another, showcasing the influence of cytoplasmic inheritance. - Lack of Association with Parasites or Symbionts:
Only those traits that are not linked to cytoplasmic parasites, symbionts, or viruses are considered to be truly governed by plasmagenes. This distinction is important in identifying genuine cases of cytoplasmic inheritance versus those influenced by external factors.
Evidence for Cytoplasmic Factors in Inheritance
The following points outline the key evidences supporting the role of cytoplasmic factors in genetic inheritance:
- Uniparental or Maternal Inheritance
- Mechanism: In many organisms, the female gamete (egg) provides nearly all the cytoplasm to the zygote, while the male gamete (sperm or pollen) contributes primarily genetic material. This difference in contribution can lead to inheritance patterns that are not explained by traditional Mendelian genetics.
- Example: In Limnaea peregra, a type of snail, the pattern of shell coiling is inherited maternally, suggesting that the trait is linked to factors located in the cytoplasm of the egg.
- Reciprocal Crosses and Inheritance Patterns
- Observation: Differences in inheritance patterns observed between reciprocal crosses (crossing two organisms and then reversing the sexes in a second cross) can indicate extranuclear factors if these differences are not attributable to chromosomal linkage.
- Example: Chloroplast inheritance in Mirabilis jalapa, a type of flowering plant, demonstrates how traits linked to chloroplasts exhibit different patterns in reciprocal crosses, pointing to cytoplasmic inheritance.
- Uniparental Inheritance with Non-Nuclear Factors
- Consideration: Traits that exhibit uniparental inheritance but do not align with unequal contributions of cytoplasm from parental gametes might involve cytoplasmic factors.
- Example: Streptomycin resistance in Chlamydomonas, a type of green algae, shows uniparental inheritance patterns that are attributed to cytoplasmic factors rather than unequal gametic contributions.
- Deviation from Classical Segregation Patterns
- Insight: When traits do not follow standard Mendelian segregation ratios, it may indicate a cytoplasmic mode of inheritance rather than chromosomal factors.
- Example: Mitochondrial inheritance in yeast often deviates from classical Mendelian ratios, suggesting that mitochondria, which have their own DNA, play a role in this inheritance.
- Independent Assortment from Nuclear Genes
- Indicator: Traits that do not link to known nuclear linkage groups and assort independently from nuclear genes are likely to be governed by cytoplasmic factors.
- Analysis: This independent assortment supports the presence of extranuclear inheritance mechanisms that are separate from the nuclear genome.
- Segregation During Mitotic Division
- Observation: Many mutants exhibiting cytoplasmic inheritance patterns show segregation during mitotic divisions, particularly in plants with variegated leaves or tissues containing multiple types of plastids.
- Example: Variegated plants often display different plastid types within the same cell, leading to a pattern of inheritance linked to cytoplasmic factors.
Examples of Cytoplasmic Inheritance
Pigment in flour moth (Ephestia kuhniella)
The pigment inheritance in the flour moth (Ephestia kuhniella) presents a clear example of the maternal effect, first studied by Caspari in 1936. This case involves the inheritance of eye and body pigmentation, which is influenced by the gene responsible for producing a pigment precursor, kynurenine.
- Genetic Basis:
Pigmentation in the flour moth is controlled by a dominant gene, A, which facilitates the production of kynurenine. Individuals with a homozygous recessive genotype (aa) lack this precursor, leading to the absence of pigmentation. As a result, moths with this genotype exhibit red-colored eyes instead of the dark brown characteristic of pigmented moths. - Cross Between Heterozygote and Homozygous Recessive:
When a pigmented heterozygote (Aa) male is crossed with a non-pigmented homozygous recessive (aa) female, the expected genetic ratio of the offspring is 1:1, with half being heterozygous (Aa) and the other half homozygous recessive (aa). Phenotypically, this leads to a 1:1 ratio of pigmented to non-pigmented offspring. - Reciprocal Crosses:
In the reciprocal cross, where a pigmented heterozygote (Aa) female is crossed with a non-pigmented (aa) male, the initial larvae all show pigmentation. This happens because the early larvae inherit kynurenine from the cytoplasm of the mother’s eggs, temporarily displaying pigmentation. However, as the larvae mature, only the heterozygous (Aa) individuals retain pigmentation, while the homozygous recessive (aa) offspring lose pigmentation and develop red eyes. - Maternal Effect:
The maternal effect is evident in this case because the early presence of pigment in the homozygous recessive (aa) larvae is not due to their own genetic makeup but rather the kynurenine they inherit from their mother’s cytoplasm. Once they deplete this maternally provided precursor, they cannot produce their own pigment due to the absence of the dominant allele (A), resulting in their non-pigmented phenotype in adulthood. - Functional Significance:
The functional importance of this study lies in understanding how maternal cytoplasmic factors, such as the inheritance of metabolites like kynurenine, can temporarily affect phenotypic expression in early development. It illustrates how cytoplasmic inheritance can interact with nuclear genes to influence traits in a non-Mendelian fashion.
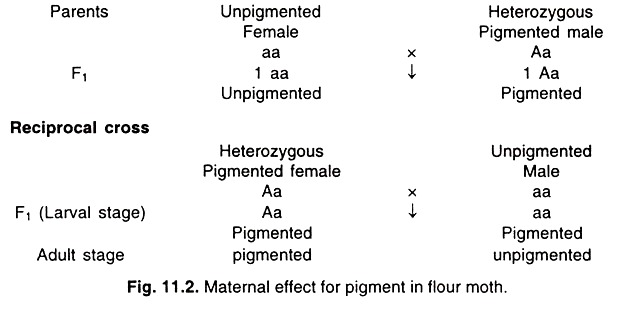
This phenomenon in Ephestia kuhniella highlights the complexity of genetic inheritance and the significant role maternal contributions play in early developmental stages, particularly through non-nuclear factors like cytoplasmic content.
Coiling of shell in snail (Limnaea peregra)
The coiling of the shell in the snail Limnaea peregra offers an intriguing case of genetic inheritance, where the direction of shell coiling, either dextral (right-coiling) or sinistral (left-coiling), is determined by the genetic composition rather than the immediate phenotype of the parents.
- Genetic Control of Coiling:
The direction of coiling is determined by a simple genetic system involving two alleles. A dominant allele (D) leads to dextral coiling, while a recessive allele (d) results in sinistral coiling. Therefore, a snail with a genotype of either DD or Dd will exhibit dextral coiling, while a snail with the dd genotype will show sinistral coiling. - Influence of the Maternal Genotype:
One of the most significant aspects of shell coiling in Limnaea peregra is the role played by the maternal genotype, rather than the phenotype, in determining the coiling direction of the offspring. In reciprocal crosses between dextral (DD) and sinistral (dd) snails, it becomes apparent that the genotype of the female parent dictates the coiling pattern in the progeny. For instance, when a dextral (DD) female is crossed with a sinistral (dd) male, the offspring will exhibit dextral coiling due to the maternal genotype. - Delayed Genotypic Effect:
This phenomenon represents a delayed effect of the genotype, whereby the appearance of the offspring is influenced by the maternal genotype in the previous generation rather than the immediate phenotype. This delayed influence underscores the importance of understanding how genetic information in the maternal lineage can affect development. - Reciprocal Crosses:
In crosses where the female parent is heterozygous (Dd), the offspring may display either dextral or sinistral coiling, depending on the specific genetic combination passed from the mother. For example, a Dd female crossed with a dd male will produce a mix of dextral and sinistral offspring, despite the mother herself being dextral in appearance. - Absence of Phenotypic Influence:
A key observation is that the phenotype of the female parent has no direct influence on the offspring’s coiling pattern. Instead, the genotype carried by the female, specifically whether it possesses the dominant allele (D), determines whether the progeny will be dextral or sinistral. Thus, while a female snail may appear dextral, her offspring may still inherit sinistral coiling if her genotype contains the recessive d allele. - Importance in Evolutionary Biology:
This delayed genetic effect on coiling direction is of particular interest in evolutionary studies. It provides insight into how genetic traits may be passed through generations without immediate expression, highlighting the complexity of inheritance beyond Mendelian genetics.
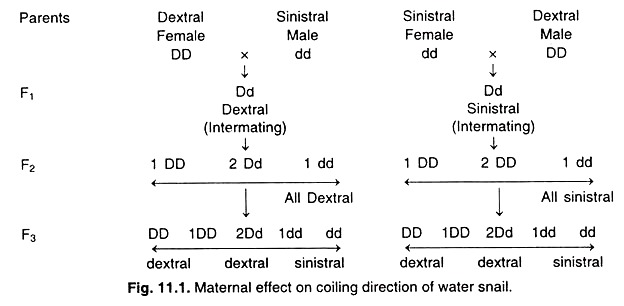
Leaf variegation in plants
Leaf variegation is a fascinating phenomenon in plants where leaves exhibit distinct color patterns, typically appearing as a mosaic of green and white patches. This condition is not only visually striking but also biologically intriguing due to its inheritance patterns and underlying mechanisms. One of the most studied cases of leaf variegation was discovered by Carl Correns in the four-o’clock plant (Mirabilis jalapa), providing key insights into cytoplasmic inheritance.
- Observation in Four-O’Clock Plant:
Correns’ work on Mirabilis jalapa revealed that variegated plants could have branches with either all-green leaves, all-white leaves, or a mixture of green and white patches. This variegation is maternally inherited, meaning the progeny always resembled the female parent in terms of leaf color. For example, seeds produced by flowers on green branches consistently gave rise to plants with normal green leaves, regardless of the phenotype of the pollen donor. - Effect of the Maternal Parent:
In his experiments, Correns found that the phenotype of the offspring was entirely dictated by the maternal parent. When seeds were taken from white branches, the resulting progeny were always white, regardless of the pollen donor’s phenotype. Since white leaves lack chlorophyll, these progeny typically died in the seedling stage, being unable to carry out photosynthesis. - Variegated Progeny:
When seeds were collected from flowers on variegated branches, the offspring displayed a range of phenotypes—green, white, and variegated—in varying proportions. Again, this pattern occurred irrespective of the male parent’s contribution. The critical finding here was that the male parent’s genotype had no impact on the inheritance of the variegation trait, further emphasizing the role of maternal cytoplasmic factors in controlling this characteristic. - Mechanism of Variegation:
The mechanism underlying leaf variegation is closely linked to the presence of plastids, which are cytoplasmic organelles responsible for photosynthesis. In variegated plants, some plastids may be functional, producing chlorophyll and giving rise to green tissue, while others are defective, leading to white patches. The inheritance of plastids occurs maternally through the cytoplasm of the egg cell, which explains why the progeny’s phenotype matches that of the female parent. - Reciprocal Crosses:
Correns also conducted reciprocal crosses to confirm his findings. In these experiments, regardless of whether the female parent had green, white, or variegated leaves, the offspring always reflected the phenotype of the mother. This stark difference in the outcomes of reciprocal crosses illustrated the non-Mendelian inheritance pattern typical of cytoplasmic inheritance, where the male parent’s contribution is negligible. - Significance of the Findings:
These observations provided early evidence of cytoplasmic inheritance, where genetic material outside the nucleus—specifically in the cytoplasm—plays a crucial role in determining certain traits. In the case of Mirabilis jalapa, the plastids in the cytoplasm of the egg determined the leaf color of the progeny. This discovery was significant in shifting the understanding of inheritance beyond Mendelian nuclear genes to include cytoplasmic elements. - Practical Implications:
Understanding leaf variegation and its inheritance is not only of academic interest but also holds practical value in horticulture and plant breeding. Variegated plants are often prized for their ornamental value, and the ability to predict and control variegation through maternal inheritance allows breeders to cultivate specific plant varieties with desirable aesthetic traits.
Kappa particles in Paramecium
Kappa particles play a crucial role in the biology of certain strains of Paramecium, particularly those identified as “killer strains.” These particles are responsible for producing a toxic substance called paramecin, which is harmful to sensitive strains that do not possess kappa particles. The inheritance and behavior of kappa particles in Paramecium offer a clear example of cytoplasmic inheritance, and their presence is linked to specific genetic factors.
- Kappa Particles and Paramecin:
Kappa particles are cytoplasmic symbionts found in killer strains of Paramecium. These particles produce paramecin, a substance that is toxic to other sensitive strains that lack kappa particles. Sensitive strains are vulnerable to the toxic effects of paramecin, making them susceptible to killer strains during interactions. - Genetic Control by the Dominant Allele K:
The production and maintenance of kappa particles in Paramecium are governed by the dominant allele K. Killer strains, which possess kappa particles, are either homozygous dominant (KK) or heterozygous (Kk). In contrast, sensitive strains are homozygous recessive (kk), and as a result, they do not have kappa particles.
It is important to note that while the dominant allele K is essential for the presence of kappa particles, the allele alone cannot produce them. Kappa particles must already be present in the cytoplasm for their continued existence and reproduction. - Absence of Kappa Particles in Certain Strains:
Although the dominant allele K allows the maintenance of kappa particles, it is possible to have strains with the KK or Kk genotype that lack kappa particles. This happens when kappa particles are lost during rapid asexual reproduction. If the rate of cell division is faster than the division of kappa particles, the particles can be lost, and the resulting progeny, despite having the dominant allele, will no longer possess kappa particles. However, killer strains with the genotype kk cannot exist, as they lack the genetic ability to maintain kappa particles. - Conjugation and Kappa Particle Transfer:
When killer (KK) and sensitive (kk) strains conjugate, the outcome depends on the duration of the conjugation process. After conjugation, the exconjugants (the two cells separating after conjugation) will all have the genotype Kk. However, the phenotype of these exconjugants varies based on whether cytoplasmic exchange, including the transfer of kappa particles, has occurred.- If conjugation is brief and does not last long enough for cytoplasmic exchange, the exconjugants will retain their original phenotypes. Killers will remain killers, and sensitive strains will remain sensitive, even though their genotypes will be Kk.
- If conjugation persists long enough for kappa particles to be transferred, the previously sensitive strain will acquire kappa particles and become a killer. Thus, all exconjugants will be killers with the genotype Kk, capable of producing paramecin (Figure B).
- Cytoplasmic Inheritance:
The behavior of kappa particles in Paramecium is a classic example of cytoplasmic inheritance, where genetic information outside the nucleus—in this case, the kappa particles within the cytoplasm—plays a crucial role in determining the phenotype. The dominant allele K is necessary for the maintenance of kappa particles, but without cytoplasmic transfer, sensitive strains cannot acquire them.
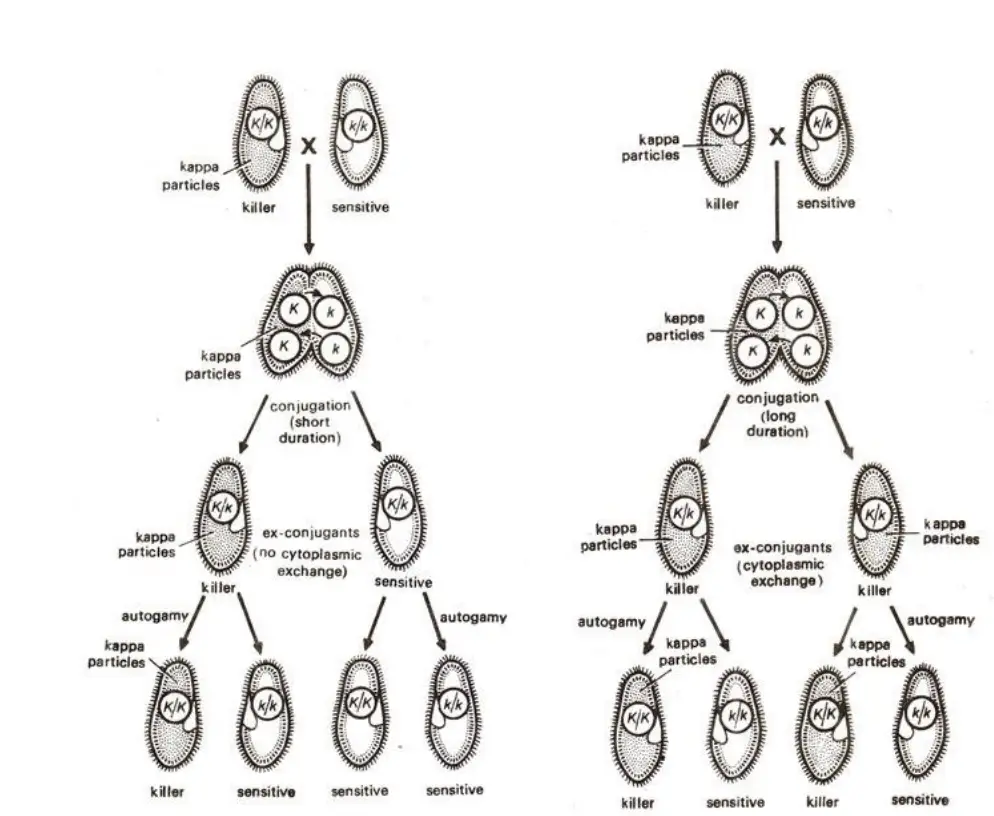
Milk factors in mice
The concept of “milk factors” in mice, identified by J.J. Bittner, highlights a significant example of how non-genetic factors can influence susceptibility to diseases. These factors are particularly notable for their role in transmitting susceptibility to mammary cancer through maternal milk. The study of these factors provides insights into the mechanisms of disease transmission and the impact of maternal influences on offspring health.
- Identification of Milk Factors:
J.J. Bittner discovered that certain factors in the milk of female mice (dams) could influence the susceptibility of their offspring to mammary cancer. This factor is not a genetic trait but rather an extra-nuclear element that behaves similarly to a virus. It is transmitted through the maternal milk and can affect the health of the young mice. - Transmission and Effects:
- Milk Transmission: The milk factor is transmitted from mother to offspring during lactation. Mice that are nursed from mothers with this factor are more prone to developing mammary cancer compared to those nursed from foster mothers whose milk lacks this factor.
- Other Body Fluids: Besides milk, this factor is also transmitted through other body fluids, such as saliva and semen. This broad mode of transmission indicates that the factor can be disseminated through various means, potentially influencing multiple aspects of maternal-offspring interactions.
- Influence of Maternal Nourishment:
During gestation, mothers nourish their embryos for several months before birth. This extended period of maternal care allows for significant influence over the developing offspring. The presence of the milk factor in maternal body fluids means that the mother’s condition and the factors she transmits can have lasting impacts on her progeny’s health. - Experimental Observations:
Studies have shown that the progeny of mothers with the milk factor exhibit increased susceptibility to mammary cancer compared to those from foster mothers without the factor. This suggests that the milk factor has a substantial effect on the likelihood of developing the disease, independent of genetic predispositions. - Scientific Implications:
The concept of milk factors challenges the traditional view that disease susceptibility is solely a result of genetic inheritance. It underscores the importance of maternal factors and environmental influences in determining disease outcomes. The behavior of these factors, akin to viral agents, adds complexity to our understanding of disease transmission and inheritance.
Male Sterility in Plants
Male sterility in plants refers to the inability of a plant to produce functional pollen, while the ovules remain unaffected. This trait plays a crucial role in plant breeding and hybrid seed production, as it facilitates cross-pollination by preventing self-fertilization. The phenomenon of male sterility is often controlled by factors beyond the nuclear genome, specifically within the cytoplasm, making it a key example of cytoplasmic inheritance. While much remains to be understood about the exact elements responsible for this type of sterility, certain mechanisms have been identified in various plant species.
Types of Male Sterility
- Cytoplasmic Male Sterility (CMS)
- Definition: Cytoplasmic male sterility is a condition where male sterility is inherited through the cytoplasm of the female parent. In this case, the trait is not influenced by the nuclear DNA but is instead transmitted through extranuclear elements in the cytoplasm.
- Mechanism: The sterility arises due to the interaction between mitochondrial genes and nuclear genes. However, specific cytoplasmic organelles, such as plastids, have not been definitively identified as the cause of male sterility. It is assumed that mitochondria might play a significant role, as they are involved in energy production for various cellular processes, including pollen development.
- Example: In corn and onions, cytoplasmic male sterility has been observed, where the plant produces sterile pollen but retains functional ovules. This form of male sterility is maternally inherited, meaning it is passed down through the female line.
- Genetic Male Sterility
- Definition: In genetic male sterility, the sterility is caused by nuclear genes. This form of sterility is different from cytoplasmic male sterility because it is not influenced by the maternal cytoplasm but instead depends on specific mutations or genetic factors present in the nuclear DNA.
- Mechanism: Mutations in nuclear genes can interfere with pollen development, resulting in the abortion of pollen grains. However, since this form of sterility is controlled by nuclear genes, it follows Mendelian patterns of inheritance.
- Example: In rice and other crops, nuclear genes can cause male sterility, although this form is typically more manageable than cytoplasmic male sterility for breeders.
Classical Example: Male Sterility in Maize
One of the most well-known examples of cytoplasmic male sterility was discovered by M.M. Rhoades in maize (corn). Rhoades conducted experiments in which he replaced all the chromosomes of a male-sterile plant with those from a normal plant. Despite this genetic replacement, the male sterility persisted, leading to the conclusion that this trait is rooted in the cytoplasm rather than the nuclear genome. The fact that male sterility continued to be expressed after the replacement of chromosomes provides compelling evidence of cytoplasmic inheritance.
- Pollen Abortion: In this case, male sterility manifests through the complete abortion of pollen, while the ovules remain functional. This type of sterility allows for cross-pollination, which is essential for creating hybrids in plant breeding.
- Inheritance: The trait is inherited entirely through the maternal line, indicating that elements within the cytoplasm, possibly mitochondrial genes, are responsible for the sterility.
Applications in Agriculture
Male sterility, particularly cytoplasmic male sterility, has significant applications in agriculture and plant breeding:
- Hybrid Seed Production: Male sterility allows for the controlled production of hybrids, as it prevents self-pollination and encourages cross-pollination. This is especially useful in crops like maize, rice, and sunflower, where hybrid vigor can improve yield and disease resistance.
- Elimination of Hand-Pollination: In large-scale agricultural practices, male-sterile plants eliminate the need for labor-intensive hand-pollination, which was traditionally used to prevent self-fertilization.
Extra-nuclear inheritance in eukaryotes
A. Maternal Inheritance
Maternal inheritance is a genetic phenomenon where specific traits observed in progeny are not solely determined by the progeny’s own genotype but are influenced by the genotype of the mother. This form of inheritance highlights the impact of maternal contributions on the early development and phenotypic characteristics of offspring.
Maternal inheritance involves the transmission of traits from the mother through substances present in the ooplasm of the egg cell, rather than through direct genetic contributions from both parents. These substances include:
- Transcriptional Products: Maternal mRNA, rRNA, and tRNA are synthesized during oogenesis and stored in the ooplasm of unfertilized eggs. These molecules can affect the development of the embryo by influencing early developmental processes before the zygote’s own genes become active.
- Inactive Forms: These maternal transcripts are often present in an inactive form and may include protein-coated or late-translating mRNA molecules, as well as inactivated rRNA and tRNA.
- Early Developmental Influence: During the initial stages of cleavage and blastulation, the transcriptional activity of the embryo is minimal. Consequently, maternal products have a pronounced effect on early developmental events and phenotypic traits.
Examples of Maternal Inheritance
- Shell Coiling in Snails (Limnaea peregra):
- Phenotypic Expression: In snails, the direction of shell coiling (dextral or sinistral) is a classic example of maternal effect. Dextral coiling, which is clockwise, and sinistral coiling, which is counterclockwise, are controlled by allelic variations of a single gene.
- Genetic Mechanism: The gene for sinistrality (S) is recessive, while dextral coiling (S+) is dominant. When eggs from a homozygous sinistral snail (SS) are fertilized by sperm from a dextral snail (S+S+), the resulting F1 progeny exhibit sinistral coiling despite possessing the genotype S+S.
- Maternal Effect: The F1 progeny express the coiling pattern determined by maternal genotype, reflecting the influence of maternal egg organization on the direction of shell coiling. In subsequent generations, segregation of the genotypes is observed, revealing the impact of maternal effects on early developmental stages.
- Eye Pigmentation in Water Fleas and Flour Moths:
- Maternal Influence on Pigmentation: In water fleas (Gammarus) and flour moths (Ephestia kuhniella), the eye color is influenced by maternal effects involving kynurenine, a substance crucial for pigment synthesis.
- Genetic Variants: Dominant genes (AA or KK) direct kynurenine production, while recessive mutants (aa or kk) lack this pigment. When aa or kk females are crossed with heterozygous males (Aa or Kk), half of the larvae exhibit dark eyes due to maternal kynurenine. However, progeny with aa or kk genotypes eventually develop light eyes due to the depletion of kynurenine.
- Ephemeral Maternal Effect: This example demonstrates how maternal substances can have a temporary impact on progeny traits. The maternal contribution ensures dark eye pigmentation in the larvae, but as kynurenine is not continuously supplied, the effect fades in the adults.
Mechanisms and Implications
- Maternal Effects on Development: Maternal inheritance underscores the importance of maternal genotype in establishing initial developmental conditions. These effects can persist through early developmental stages, influencing traits even in the absence of active maternal genes in later stages.
- Genotype vs. Phenotype: Maternal effects highlight a divergence between genotype and observable phenotype in early generations. This divergence often results from maternal factors that dominate before the embryo’s own genes become active.
- Research and Applications: Understanding maternal inheritance is crucial for fields such as developmental biology and genetics. It helps elucidate mechanisms of gene expression and trait determination that are not solely based on Mendelian inheritance.
B. Extra-nuclear Inheritance by Cellular Organelles
Extra-nuclear inheritance refers to the transmission of genetic traits via mechanisms outside the nuclear genome, primarily through cellular organelles such as mitochondria and chloroplasts. These organelles, containing their own DNA and machinery for protein synthesis, contribute to inheritance patterns that deviate from Mendelian genetics. The origin of these organelles is linked to endosymbiotic theories, suggesting that they were once independent prokaryotic entities that have since evolved a symbiotic relationship with their host cells.
1. Chloroplast Inheritance
Chloroplasts, the organelles responsible for photosynthesis, exhibit unique patterns of inheritance due to their extrachromosomal DNA. The inheritance of chloroplast traits is predominantly maternal, meaning that the traits are passed through the egg cell’s chloroplasts rather than the sperm’s.
- Example: Mirabilis jalapa (Four O’Clock Plant)
- Phenotypic Variations:
- Green Leaves: Contain functional chloroplasts.
- White Leaves: Lack chloroplasts and thus cannot photosynthesize.
- Variegated Leaves: Contain a mixture of green (photosynthetic) and white (non-photosynthetic) plastids.
- Inheritance Patterns:
- Flowers from green branches produce only green offspring.
- Flowers from white branches produce only white offspring.
- Flowers from variegated branches yield a mix of green, white, and variegated progeny in varying ratios.
- Mechanism:
- The cytoplasm of the egg cell dictates the color of the progeny. Eggs derived from green plants contain only green plastids, while those from white plants contain only white plastids. Variegated plants produce eggs with a mix of plastid types.
- Mitotic Segregation: During cell division, plastids segregate into different cell lines, leading to variegated phenotypes in some progeny.
- Phenotypic Variations:
2. Maternal Inheritance by Mitochondria
Mitochondria, the powerhouses of the cell, also exhibit extra-nuclear inheritance patterns. They have their own DNA, and mutations in this DNA can affect cellular energy production.
- Example: Petite Mutants in Yeast (Saccharomyces cerevisiae)
- Characteristics:
- Petite mutants exhibit reduced growth on glucose, smaller colonies, and defective aerobic respiration.
- They are characterized by a lack of certain cytochromes and mitochondrial enzymes.
- Types of Petite Mutants:
- Neutral Petite: Lacks mitochondrial DNA (mtDNA) and does not transmit the petite trait.
- Suppressive Petite: Contains defective mtDNA and can transmit the trait to some offspring.
- Evidence for Mitochondrial DNA Role:
- Ethidium bromide-induced petite mutations cause mtDNA degradation.
- Suppressive petites have altered mtDNA composition compared to wild types.
- Characteristics:
- Example: Poky Strain of Neurospora crassa
- Characteristics:
- Slow growth, maternal inheritance, and abnormal cytochrome profiles (lack of cytochromes a and b, excess of cytochrome c).
- Inheritance Patterns:
- Reciprocal crosses show that the poky trait is maternally inherited. Poky females produce all poky offspring, while wild type females produce all wild type progeny when crossed with poky males.
- Evidence for Mitochondrial Involvement:
- Slow growth correlates with deficient ATP production, a function of mitochondria.
- Altered cytochrome content supports a mitochondrial origin for the trait.
- Characteristics:
3. Male Sterility in Plants
Male sterility in plants can arise from interactions between nuclear genes and extra-nuclear elements, including plasmagenes (cytoplasmic genes).
- Types of Male Sterility:
- Genetic Male Sterility:
- Controlled by a recessive nuclear gene. In F1, all progeny are fertile, with segregation in the F2 generation.
- Cytoplasmic Male Sterility (CMS):
- Observed when the female parent possesses cytoplasmic factors causing male sterility. The F1 progeny will all be male sterile if derived from a male sterile female.
- Cytoplasmic Genetic Male Sterility:
- Involves both cytoplasmic factors and a nuclear restorer gene. The presence of a restorer gene in the male parent can restore fertility in F1 progeny.
- Genetic Male Sterility:
- Example: Maize:
- Cytoplasms:
- Normal (N) and three male sterile types: T, C, and S.
- Cytoplasmic factors responsible for CMS are located in mtDNA. The CMS-T type cannot be restored by nuclear restorer genes, unlike CMS-C and CMS-S.
- Cytoplasms:
C. Extra-Nuclear Inheritance by Endosymbionts
Extra-nuclear inheritance, or cytoplasmic inheritance, involves the transmission of genetic material located outside the nucleus. This form of inheritance can involve various intra-cellular symbionts, such as bacteria and viruses, which maintain a symbiotic relationship with their host cells. These symbionts often replicate independently and can influence the phenotypic traits of their hosts through transmission mechanisms. Here, we discuss notable examples of extra-nuclear inheritance mediated by endosymbionts.
1. Sigma Virus in Drosophila
- Overview:
- The sigma virus, an infectious DNA virus, is associated with carbon dioxide (CO2) sensitivity in Drosophila melanogaster.
- This sensitivity is a trait transmitted primarily through the maternal lineage, although paternal transmission can occasionally occur.
- Key Points:
- Transmission and Infection:
- Sigma virus particles are found in the cytoplasm of CO2-sensitive Drosophila.
- Transmission occurs predominantly through the egg cytoplasm, but sperm transmission is also possible.
- Normal Drosophila can be rendered CO2 sensitive through exposure to sigma particles from infected flies.
- Phenotypic Impact:
- The presence of sigma virus induces heightened CO2 sensitivity, which affects the coordination and overall health of the flies.
- Transmission and Infection:
2. Spirochaetes and Maternal Sex Ratio in Drosophila
- Overview:
- Spirochaete bacteria, specifically SR spirochaetes, influence sex ratios in Drosophila by affecting the survival of zygotes.
- Key Points:
- Impact on Zygotes:
- SR spirochaetes infect the eggs of female Drosophila, leading to the selective death of XY zygotes while XX zygotes survive.
- This results in a progeny predominantly composed of females.
- Transmission and Infection:
- The spirochaetes are infectious and can be transmitted to normal females, turning them into carriers.
- The exact mechanisms behind the selective sensitivity of XY zygotes and the reason for their elimination remain unclear.
- Impact on Zygotes:
3. Kappa Particles in Paramecium
- Overview: Kappa particles are cytoplasmic entities in the ciliate protozoan, Paramecium aurelia, which produce a toxin called paramecin.
- Key Points:
- Toxin Production:
- Paramecin, a diffusible and water-soluble substance, is lethal to sensitive strains of Paramecium.
- Kappa particles are essential for paramecin production and are maintained by a dominant nuclear gene K.
- Inheritance Patterns:
- Conjugation between killer (Kk) and non-killer (kk) Paramecia can result in progeny exhibiting varying traits based on the extent of cytoplasmic mixing.
- Prolonged conjugation allows for the transfer of kappa particles, leading to killer progeny.
- Toxin Production:
4. mμ Particles in Paramecium
- Overview: The mμ particles are involved in a killer trait known as mate killer in Paramecium.
- Key Points:
- Mate Killer Trait:
- Paramecia carrying mμ particles will kill mating partners that lack these particles.
- The presence of mμ particles is linked to dominant nuclear genes (M1 and M2) that facilitate their maintenance.
- Composition: mμ particles consist of DNA, RNA, and other cellular components, functioning as cytoplasmic symbionts.
- Mate Killer Trait:
5. Milk Factor in Mice
- Overview: The milk factor is a maternally transmitted trait in mice that increases susceptibility to mammary cancer.
- Key Points:
- Transmission and Influence:
- The trait is transmitted through milk, saliva, and semen.
- Mice from low-cancer strains, when nursed by females from high-cancer strains, show increased cancer incidence.
- Mechanism and Dependencies: The milk factor resembles a virus in its transmissibility and depends on nuclear genetic factors for its manifestation.
- Transmission and Influence:
D. Uniparental Inheritance in Chlamydomonas reinhardtii
Uniparental inheritance is a genetic phenomenon where only one parent contributes to the transmission of certain traits to offspring, contrasting with the typical biparental inheritance where both parents contribute. In the green alga Chlamydomonas reinhardtii, this concept is illustrated through the inheritance of mitochondrial traits, particularly in relation to its mating types and specific mutations.
Overview of Chlamydomonas reinhardtii
- Mating Types:
- In Chlamydomonas reinhardtii, there are two primary mating types, designated as mt+ and mt—.
- These mating types are functionally distinct but morphologically identical, meaning that individuals of different mating types must mate to produce offspring.
- Heterothallic Nature:
- Chlamydomonas is a heterothallic species, meaning that successful sexual reproduction occurs only between individuals of different mating types.
Uniparental Inheritance in Chlamydomonas reinhardtii
- Identification of Uniparental Inheritance:
- Ruth Sager’s research in 1954 identified uniparental inheritance in Chlamydomonas through the study of a streptomycin-sensitive mutant (sm-s).
- The uniparental inheritance pattern was evident in the reciprocal crosses between strains with different mating types and streptomycin resistance or sensitivity.
- Experimental Observations:
- In crosses involving mt+ sm-r (streptomycin-resistant) and mt— sm-s (streptomycin-sensitive) strains, all progeny exhibited the streptomycin resistance phenotype of the mt+ parent.
- Conversely, when mt+ sm-s (streptomycin-sensitive) was crossed with mt— sm-r (streptomycin-resistant), all progeny displayed the streptomycin sensitivity phenotype of the mt— parent.
- Uniparental Contribution:
- The observed phenotype in progeny consistently mirrored the streptomycin sensitivity or resistance of the mt+ parent, regardless of the other parent’s phenotype.
- This indicates that the phenotype associated with the mt+ mating type is inherited exclusively through the mt+ parent, aligning with the concept of uniparental inheritance.
Implications of Uniparental Inheritance
- Maternal Analogy:
- Sager’s findings led to the analogy where the mt+ mating type is referred to as the “female” in this context.
- This terminology helps clarify that, similar to maternal inheritance observed in other organisms, the mt+ parent’s traits are passed on without contribution from the mt— parent.
- Functional Significance:
- The uniparental inheritance observed in Chlamydomonas reinhardtii underscores the unique role of the cytoplasmic genome in determining certain phenotypic traits.
- It illustrates how specific genetic elements, such as mitochondrial DNA, can exhibit inheritance patterns independent of the nuclear genome.
Significance of Cytoplasmic Inheritance
The significance of cytoplasmic inheritance is multifaceted and extends across various biological and agricultural domains. Below are the key points that highlight its importance:
- Understanding the Role of Cytoplasmic Organelles:
Cytoplasmic inheritance has been instrumental in demonstrating how cytoplasmic organelles, such as mitochondria and chloroplasts, contribute to the transmission of genetic traits. By studying this type of inheritance, scientists have been able to explore how non-nuclear DNA influences phenotypic characteristics across different organisms. - Mapping of Organelle Genomes:
Research into cytoplasmic inheritance has significantly contributed to the mapping of chloroplast and mitochondrial genomes in several species, including yeast, Chlamydomonas, maize, and humans. These maps provide a deeper understanding of organelle functions and their genetic makeup, further expanding our knowledge of non-nuclear inheritance. - Development of Cytoplasmic Male Sterility (CMS):
One of the most practical applications of cytoplasmic inheritance is the development of cytoplasmic male sterility (CMS) lines in various crops such as maize, pearl millet, sorghum, and cotton. CMS lines are critical for the production of hybrid seeds, allowing for the generation of superior crop varieties without the need for manual emasculation and pollination. This technique significantly reduces the cost of hybrid seed production, making it more economically viable. - Advancements in Hybrid Breeding:
Cytoplasmic male sterility can be transferred across different agronomic lines, enabling the development of superior hybrid crops. However, it is important to diversify the CMS sources to prevent genetic uniformity, which could make crops more susceptible to diseases and pests. - Role of Mitochondria in Heterosis:
Mitochondria have been increasingly recognized for their role in the manifestation of heterosis, or hybrid vigor. This phenomenon, where hybrids exhibit superior qualities compared to their parents, is of growing interest in plant and animal breeding. - Generation of New Variants:
Mutations in chloroplast DNA and mitochondrial DNA can lead to the creation of new genetic variants. Some of these mutations, particularly in ornamental plants, have significant value due to their unique phenotypic traits. Such mutations may contribute to the diversity and commercial appeal of these plants in horticulture.
- https://uomustansiriyah.edu.iq/media/lectures/6/6_2020_05_12!07_22_20_PM.pdf
- http://courseware.cutm.ac.in/wp-content/uploads/2020/05/L-14.pdf
- https://www.biologydiscussion.com/genetics/cytoplasmic-inheritance/cytoplasmic-inheritance-characteristics-and-examples/37155
- https://www.biologydiscussion.com/genetics/2-main-cases-of-cytoplasmic-inheritance-genetics/59770
- http://tumkuruniversity.ac.in/oc_pg/bot/MSc%20Botany%20CPT%202.2%20Cytoplasmic%20Inheritance.pdf
- http://eagri.org/eagri50/GBPR111/lec07.pdf
- https://www.davuniversity.org/images/files/study-material/EDU%20161,Chromosomal%20Theory%20of%20Inheritance.pdf
- https://www.gdcollegebegusarai.com/course_materials/hindi/zol24.pdf
- https://www.biosciproceedings.org/bp/0014/pdf/bp0014cpr3.pdf
- http://fatchiyah.lecture.ub.ac.id/files/2011/04/Lect-3-ok-Extranuclear-Inheritance.pdf
- https://www.biologydiscussion.com/genetics/cytoplasmic-inheritance/notes-on-cytoplasmic-inheritance-genetics/37872