What is ATP Synthase?
ATP synthase is an essential enzyme complex responsible for the production of adenosine triphosphate (ATP), the primary energy currency in living cells. Often referred to as Complex V in the oxidative phosphorylation pathway, ATP synthase plays a critical role in cellular respiration and photosynthesis, utilizing the proton gradient generated by the electron transport chain to facilitate ATP synthesis from adenosine diphosphate (ADP) and inorganic phosphate (Pi).
Structurally, ATP synthase is a multiprotein complex composed of two main domains: F₀ and F₁. The F₀ component resides in the membrane, while the F₁ component protrudes into the mitochondrial matrix or the chloroplast stroma, depending on the organism. The F₀ domain functions as a proton channel, allowing protons (H⁺ ions) to flow down their concentration gradient from the intermembrane space into the mitochondrial matrix. This movement of protons generates a rotational force, a process known as the proton motive force.
As protons move through the F₀ domain, they drive the rotation of the F₁ domain, which contains the active sites for ATP synthesis. The mechanical energy produced by this rotation catalyzes the phosphorylation of ADP, allowing it to combine with inorganic phosphate to form ATP. The overall reaction can be summarized as follows:

This process is highly efficient and demonstrates the intricate coupling of biochemical energy conversion.
ATP synthase is not only found in mitochondria of eukaryotic cells but is also present in the thylakoid membranes of chloroplasts and in the cytoplasmic membranes of bacteria. This conservation across diverse organisms indicates its fundamental role in energy metabolism. In chloroplasts, during photosynthesis, ATP synthase utilizes the proton gradient established by the light-dependent reactions to produce ATP, which is then utilized in the Calvin cycle to synthesize glucose.
The importance of ATP synthase extends beyond its enzymatic function; it serves as a prime example of how cellular structures have evolved to efficiently harness energy. By coupling the flow of protons to ATP synthesis, this enzyme complex exemplifies the principles of bioenergetics and highlights the sophistication of cellular mechanisms that sustain life. Understanding the intricacies of ATP synthase not only deepens our knowledge of cellular respiration and photosynthesis but also provides insights into potential therapeutic targets for diseases associated with mitochondrial dysfunction.
Structure of ATP Synthase
The structure of ATP synthase is crucial for its function in synthesizing adenosine triphosphate (ATP), the primary energy currency in biological systems. This enzyme complex comprises two main components: the membrane-bound F₀ region and the soluble F₁ region, which together facilitate the conversion of energy stored in a proton gradient into chemical energy.
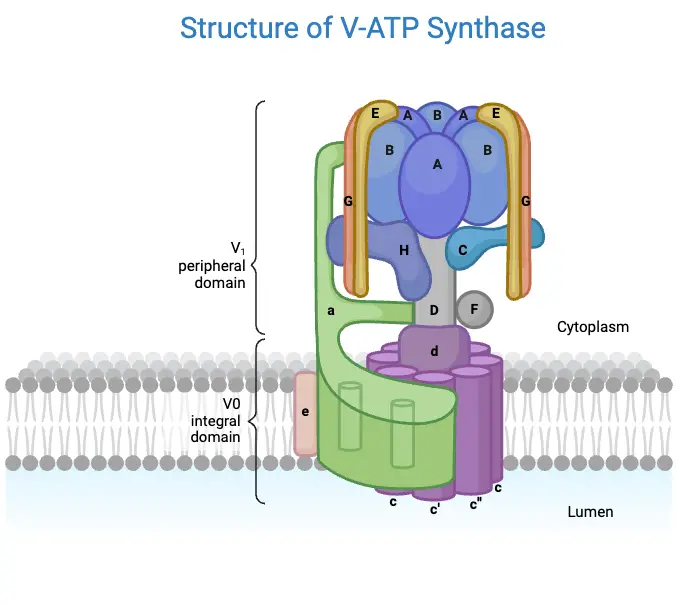
- F₀ Region
- The F₀ component is hydrophobic and embedded within the inner mitochondrial membrane.
- It consists of three subunits: one α-subunit, two β-subunits, and 9 to 12 c-subunits arranged in a circular configuration.
- The c-subunits contain two alpha helices that extend into the thylakoid membrane, forming a regulated proton channel.
- This region serves as a channel for protons (H⁺ ions), enabling their passage across the membrane and playing a vital role in ATP synthesis.
- The α-subunit acts as a mediator, facilitating the transfer of protons between the c-ring and the external environment.
- The F₀ component is also connected to several subunits of the F₁ region through a peripheral stalk.
- Importantly, F₀’s activity can be inhibited by the antibiotic oligomycin, which completely blocks the phosphorylation of ADP to ATP, demonstrating its regulatory significance.
- F₁ Region
- The F₁ component is hydrophilic and resides in the mitochondrial matrix, functioning as the catalytic site for ATP synthesis.
- It comprises five subunits: three α-subunits, three β-subunits, and one each of γ, δ, and ε subunits.
- The arrangement of the α and β subunits forms a hexameric ring structure known as α₃β₃, which is crucial for ATP synthesis.
- Among these subunits, only the β-subunits are capable of catalyzing the phosphorylation of ADP, leading to ATP production.
- The γ, δ, and ε subunits form a central stalk that connects the F₁ region to the F₀ region, facilitating communication between the two domains.
- Functional Components
- The assembly of c-subunits (9-12) with the γ and ε subunits creates the rotor, which is the moving unit of ATP synthase. As protons traverse the thylakoid membrane, the rotor undergoes rotational motion, driving the synthesis of ATP.
- The stator is comprised of the β and α subunits, along with the α₃β₃δ complex, which remains stationary during the enzymatic activity.
Mechanism of ATP Synthesis
ATP synthesis, crucial for energy production in living organisms, is explained by the “rotational catalysis” model, which was proposed by Paul Boyer and recognized with the Nobel Prize in 1997. The primary energy-requiring process in ATP synthesis is not the formation of ATP itself but the change in affinity of the β-subunits for ADP, Pi, and ATP. This process is driven by conformational changes in protein subunits, coupled with the rotation of a shaft in the enzyme complex. The synthesis mechanism, believed to be the same for both chloroplasts and mitochondria, can be described through the rotation of the γ- and ε-subunits in response to proton movement, generating an electrochemical gradient.
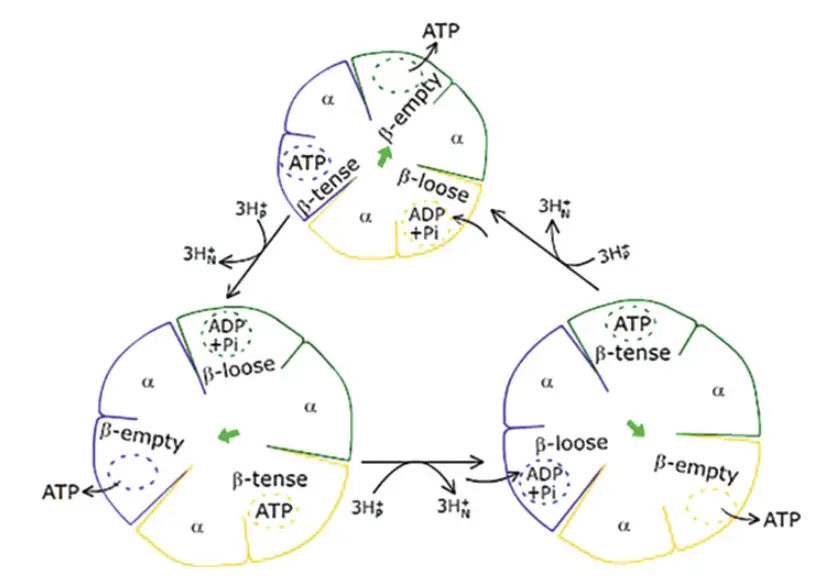
- F1 and Binding Change Mechanism
- The F1 portion of the ATP synthase complex is a hexamer, composed of alternating α and β subunits (α3β3). The β-subunits are catalytic and interact with ADP, Pi, and ATP.
- Each β-subunit exists in one of three conformations:
- β-ADP (Loose): Binds ADP and Pi.
- β-ATP (Tense): Synthesizes ATP.
- β-empty (Open): Releases ATP.
- The β-subunits cycle through these conformations in sequence. One β-subunit in the loose state is adjacent to one in the tense state and another in the open state, ensuring that at any given time, adjacent subunits are in different conformations.
- Conformational changes are driven by the rotation of the γ-subunit, which causes ATP to be released from the β-empty subunit. This rotation occurs in discrete steps of 120°, and with each complete 360° rotation, all β-subunits cycle through the three conformations.
- The direction of γ-subunit rotation is critical: in the absence of a proton gradient, the enzyme catalyzes ATP hydrolysis. The Japanese researchers Masasuke Yoshida and Kazuhiko Kinoshita experimentally demonstrated this model using video microscopy to show γ-subunit rotation during ATP hydrolysis.
- Fo Subunit and Proton Translocation
- The movement of the γ- and ε-shaft is linked to the rotation of the c-ring within the Fo portion of ATP synthase. This rotation is powered by proton movement across the membrane in response to an electrochemical gradient.
- Fo contains two primary subunit types: a single a-subunit and multiple c-subunits (8 to 15, depending on the organism). The c-ring functions like a nanomotor, rotating as protons are transported through the membrane.
- The rotation of the c-ring drives the movement of the γ- and ε-subunits, which in turn induces conformational changes in the F1 β-subunits.
- The a-subunit, part of the stator along with two b-polypeptides, anchors the structure. Two proton channels, embedded within the a-subunit, facilitate proton movement from one side of the membrane to the other. Protons travel from the high-concentration side (P side) to the membrane’s middle and then to the low-concentration side (N side).
- A key component in this process is the conserved carboxyl group on the c-subunits (Asp61 in E. coli), which protonates during translocation. This reduces electrostatic interactions between the protonated c-subunit and the a-subunit’s arginine residue (Arg210), causing the c-ring to rotate.
- The c-ring’s rotation is unidirectional, possibly due to the presence of two proton half channels and repulsion between Arg210 and the proton-loaded c-subunit. Each complete rotation of the c-ring, consisting of 10 c-subunits, translocates 10 protons, driving the γ- and ε-shaft rotation by 360° and enabling the synthesis of three ATP molecules.
- Energy Conservation and Proton Gradient
- The proton gradient generated by the electron transport chain provides the driving force for ATP synthesis. A complete cycle of proton translocation—moving 10 protons—synthesizes three ATPs.
- Transport of electrons from NADH to ½ O2 releases 214 kJ/mol of free energy, which creates a gradient sufficient for ATP synthesis. The synthesis of one ATP molecule requires around 50 kJ/mol of energy, with excess energy likely dissipated as heat.
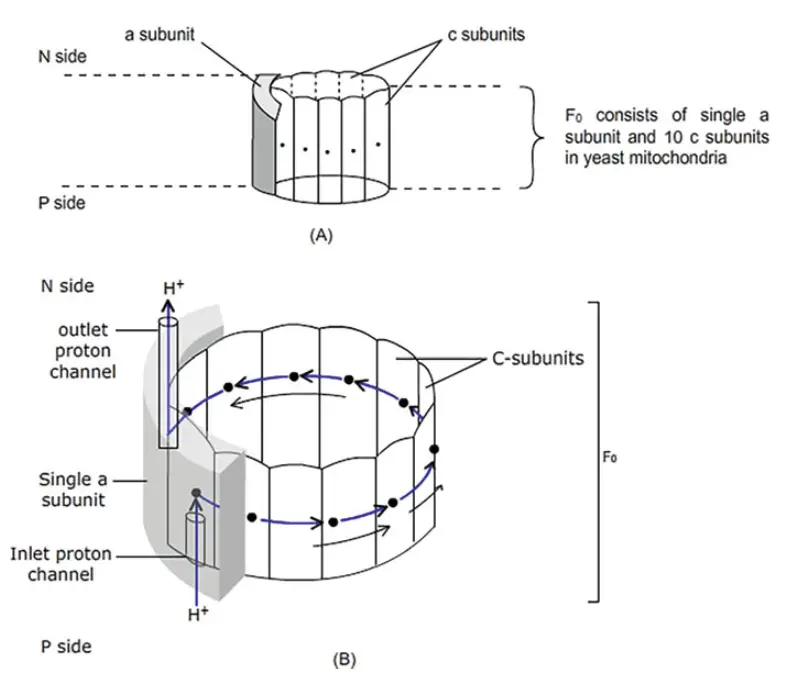
Significances of ATP Synthase
ATP synthase plays a vital role in cellular metabolism, primarily as the enzyme responsible for synthesizing adenosine triphosphate (ATP), which serves as the energy currency of the cell. The significance of ATP synthase extends beyond its catalytic function; it is also integral to several fundamental biological processes.
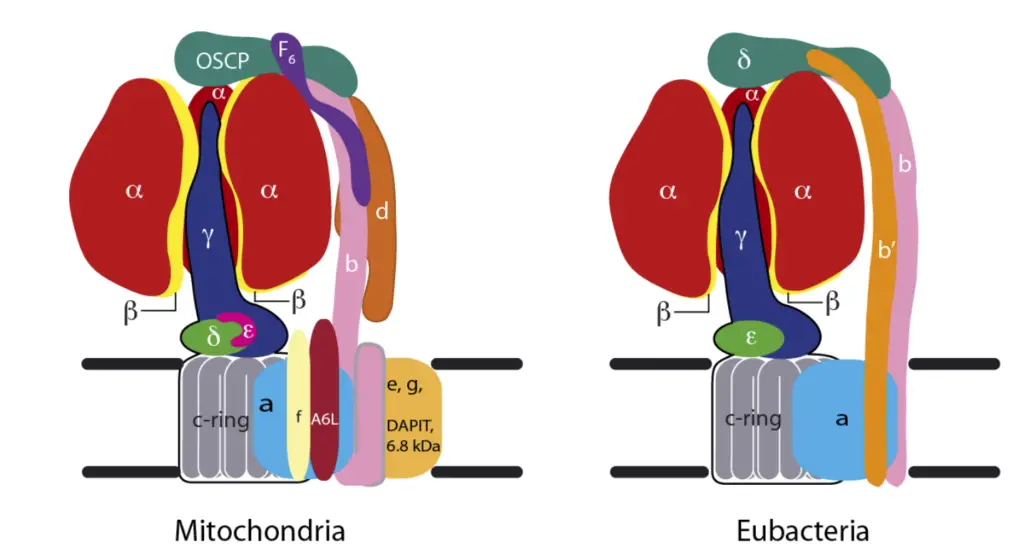
- Energy Production
- ATP synthase is essential for generating ATP, which is crucial for all cellular functions, including biosynthesis, motility, and signal transduction.
- By facilitating the conversion of ADP and inorganic phosphate (Pi) into ATP, ATP synthase ensures that cells have a constant supply of energy to sustain life.
- Maintenance of Electrochemical Gradient
- The enzyme maintains the electrochemical gradient formed by proton movement across the inner mitochondrial membrane.
- This gradient is not only critical for ATP synthesis but also supports other processes, such as nutrient transport and the operation of the electron transport chain.
- Evolutionary Conservation
- The structure and function of ATP synthase are highly conserved among diverse organisms, ranging from bacteria to higher eukaryotes.
- This conservation underscores its evolutionary significance, suggesting that ATP synthase is a fundamental component of cellular respiration that has been preserved throughout evolution due to its critical role in energy production.
- Clinical Relevance
- Understanding ATP synthase is crucial for investigating various diseases and disorders linked to dysfunctional ATP synthase, such as mitochondrial diseases, neurodegenerative disorders, and metabolic syndromes.
- Research into ATP synthase may lead to therapeutic interventions that can restore proper ATP synthesis in affected individuals.
- Role in Cellular Metabolism
- ATP synthase participates in oxidative phosphorylation, a process that is the final stage of cellular respiration, thereby connecting it to the overall metabolic network.
- The enzyme’s activity is directly influenced by the proton motive force generated during electron transport, illustrating the intricate relationship between energy production and cellular respiration.
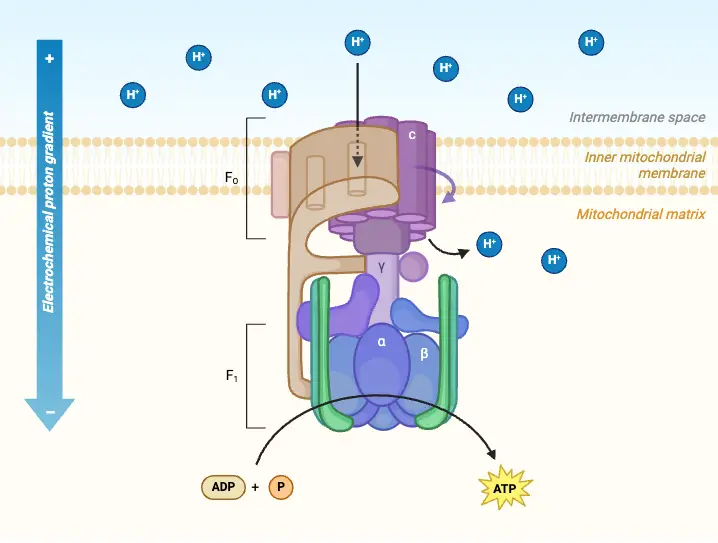
Chemiosmotic theory
Chemiosmotic theory, proposed by Peter Mitchell in 1961, represents a cornerstone in our understanding of energy production within cells, specifically regarding ATP synthesis. This theory earned Mitchell the Nobel Prize in Chemistry in 1978 for elucidating how energy derived from proton gradients across membranes can drive the formation of ATP.
- Fundamental Principles
- The chemiosmotic theory posits that the differences in ion concentration and electric potential across membranes generate a source of free energy that cells can harness.
- A high negative charge on one side of the membrane, juxtaposed with a positive charge on the opposite side, enables the formation of high-energy bonds between adenosine diphosphate (ADP) and inorganic phosphate (Pi), ultimately leading to ATP synthesis.
- Initial Evidence
- Early support for the chemiosmotic mechanism of ATP formation emerged from experiments conducted by André Jagendorf and colleagues.
- In a pivotal experiment, chloroplasts were placed in darkness to eliminate light absorption and electron transfer. The thylakoid vesicles were first incubated in a pH 4 medium, achieving uniform acidity, then quickly transferred to a pH 8 medium, creating a proton gradient.
- The addition of ADP resulted in ATP production, even in the absence of light, providing compelling evidence that a pH gradient correlates with ATP synthesis.
- Further Validation
- Efraim Racker contributed significantly by identifying and purifying Factor-1 (F1), an integral part of the ATP synthase enzyme. His work confirmed that ATP synthesis occurs not through a high-energy intermediate but rather via a transmembrane proton gradient.
- Racker’s experiments involved constructing an artificial system that included a bacterial proton pump activated by light and ATP synthase. They observed that light-induced proton pumping led to an increase in internal proton concentration, which powered ATP synthesis.
- Boyer’s Conformational Model
- Paul Boyer advanced the understanding of ATP synthesis by proposing the binding-change mechanism, which describes how ATP synthesis is coupled with proton transport.
- This mechanism involves three catalytic sites on the F1 subunit of ATP synthase, each capable of existing in one of three conformations: open (O), loose (L), and tight (T).
- ATP synthesis is not merely the reaction of ADP and Pi but also hinges on the release of ATP from the enzyme. The γ subunit’s rotation, driven by proton flow, facilitates these conformational changes, allowing substrates to bind and products to release.
- Rotary Motion of the γ Subunit
- The rotation of the γ subunit is central to ATP synthase’s function. Researchers, including Masasuke Yoshida, visualized this motion by attaching a fluorescently labeled actin filament to the γ subunit. When ATP was added, the filament swung around, demonstrating the subunit’s rotation.
- This rotary motion allows the γ subunit to act as a molecular crankshaft, interacting with the catalytic sites of the F1 component and driving ATP production.
- Structural Insights
- John E. Walker provided a three-dimensional view of ATP synthase through X-ray diffraction, validating Boyer’s model by showing the different rotational positions of the enzyme’s head during ATP synthesis.
- Together, Boyer and Walker shared the Nobel Prize in Physiology or Medicine in 1997 for their groundbreaking work on the mechanisms of ATP synthesis, alongside Jens Skou, who contributed to understanding Na+/K+-ATPase.
Rotational catalysis and ATP generation
Rotational catalysis is central to the mechanism of ATP synthesis, particularly through the enzyme ATP synthase. This process involves complex structural movements within ATP synthase, resulting in the generation of ATP through conformational changes driven by proton flow.
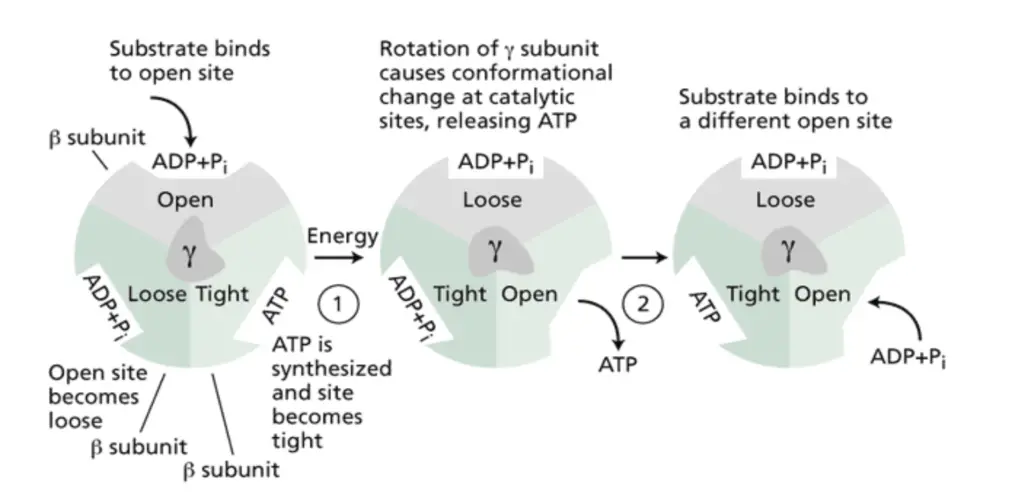
- Boyer’s Binding Change Mechanism: Paul Boyer proposed the binding change mechanism, which describes how ATP synthase uses a rotational mechanism to catalyze ATP synthesis. Each catalytic site within ATP synthase cycles through three different conformations during a 360° rotation. With every 120° rotation, ATP is generated at a different catalytic site. This mechanism involves nucleotide binding, a subsequent conformational change to tightly bind ATP, and finally, another change to release ATP.
- Subunit Functionality: ATP synthase is composed of multiple subunits, including 3α and 3β subunits arranged in a circle. Each of these subunits has a specific function and exists in different conformations. While the α subunit binds ATP, it does not release it nor participate in the reaction directly. The β subunits, however, have three catalytic sites—βT (bound to Mg·AMP-PNP1, an ATP analog), βD (bound to Mg·ADP), and βE (empty with no nucleotide). These sites exhibit different binding states, and the conformational changes within the β subunits are critical for ATP synthesis.
- Role of the γ Subunit: The γ subunit plays a pivotal role in rotational catalysis. Its rotation causes conformational changes in the β subunits, based on which part of the γ subunit is in contact with them. This rotation is driven by the proton motive force generated as protons flow into the mitochondrial matrix through the F0 subunit. As the γ subunit rotates by 120°, it interacts with a new β subunit, forcing it into the βE conformation, while the neighboring subunits assume the βD and βT forms. Each complete rotation of the γ subunit drives the β subunits through all three conformations, resulting in the synthesis of three ATP molecules.
- Proton Flow and Rotation: The flow of protons through the F0 subunit is essential for driving the rotation of ATP synthase. Protons passing through the F0 assembly cause the rotation of the c subunits, which are connected to the γ subunit. This rotational movement drives the γ subunit to rotate within the (αβ)₃ assembly, leading to conformational changes in the β subunits that enable ATP synthesis.
- Directional Rotation and ATP Hydrolysis: A key prediction of the binding-change model is that the direction of the γ subunit’s rotation differs depending on whether ATP is being synthesized or hydrolyzed. When ATP synthase is synthesizing ATP, the γ subunit rotates in one direction, driven by proton flow into the matrix. However, during ATP hydrolysis, the rotation reverses direction. This reversal occurs when the proton concentration is higher inside the mitochondrial matrix, prompting the F1 motor to reverse the F0 motor’s direction, leading to ATP hydrolysis and proton translocation across the membrane.
- Experimental Confirmation: The experiments of Masasuke Yoshida and Kazuhiko Kinosita, Jr. confirmed that the γ subunit rotates in opposite directions during ATP synthesis and hydrolysis. Further experimentation by a team of Japanese scientists demonstrated the rotational nature of ATP synthesis by attaching magnetic beads to F1-ATPase stalks in vitro. When exposed to a rotating magnetic field, these stalks rotated, and ATP synthesis occurred at a rate of approximately five molecules per second. Additionally, ATP was hydrolyzed when the rotation was reversed or when no rotation occurred.
ATP Synthase Diseases
ATP synthase is a crucial enzyme responsible for ATP production, and defects or mutations within its structure can lead to several severe diseases. The malfunction of ATP synthase, whether due to underexpression or mutations in its subunits, profoundly impacts cellular energy production and can result in life-threatening conditions.
- Initial Discovery of ATP Synthase Deficiency: The first reported defect in ATP synthase was documented by Houstek and colleagues in a child who suffered from severe lactic acidosis, cardiomyopathy, and hepatomegaly. The child passed away two days after birth. In this case, ATP synthase expression was found to be reduced by 70-80%, though no direct mutations in the enzyme itself were identified. It was hypothesized that defects in factors involved in the enzyme’s assembly could have been responsible for the condition.
- Mutations in Nuclear and Mitochondrial DNA: ATP synthase diseases may be caused by mutations in either nuclear or mitochondrial DNA. Kucharczyk et al. discussed how mutations in one or more ATP synthase subunits can lead to a range of disorders. These mutations impact the enzyme’s ability to synthesize ATP efficiently, leading to energy deficits in cells.
- Mutation in the α Subunit: Mutations affecting the α subunit of ATP synthase have been linked to several serious conditions, including neuropathy, ataxia, retinitis pigmentosa syndrome, and familial bilateral striatal necrosis. Additionally, one form of Leigh syndrome—a severe neuromuscular disorder with a survival rate of only 50% for children beyond the age of three—has been associated with α subunit mutations. These mutations not only impair ATP production but also contribute to a decline in intermediary metabolism and kidney function, due in part to increased free oxygen radicals.
- Alzheimer’s Disease and Subunit Imbalance: The improper expression of ATP synthase subunits can lead to neurodegenerative conditions. Low expression of the β subunit, along with cytosolic accumulation of the α subunit, has been connected to Alzheimer’s disease. In this case, the imbalance in subunit expression disrupts the enzyme’s function, contributing to the progressive loss of neurological function observed in Alzheimer’s patients.
- Selective ATPase Deficiency: Dysfunction of specific nuclear-encoded assembly factors involved in ATP synthase assembly can result in selective ATPase deficiency. This condition is marked by the impaired assembly of ATP synthase, leading to a significant reduction in ATP production. Newborns with this defect often have a very short life expectancy, typically dying within a few months to a year.
- Mitochondrial F-ATP Synthase Deficiency: Inborn defects in mitochondrial F-ATP synthase, also known as ATP synthase deficiency, result in early mortality, with affected newborns usually surviving only a few months. This condition highlights the essential role of ATP synthase in cellular energy production and the devastating consequences of its malfunction during early development.
ATP Synthase Inhibition
ATP synthase, one of the most ancient and conserved enzymes, plays a vital role in cellular energy production by facilitating ATP synthesis. Inhibition of ATP synthase has been explored as a therapeutic strategy for various diseases. The enzyme’s complex structure presents multiple potential inhibition sites, making it a prime target for drug development. Below, the key inhibitors of ATP synthase and their modes of action are detailed:
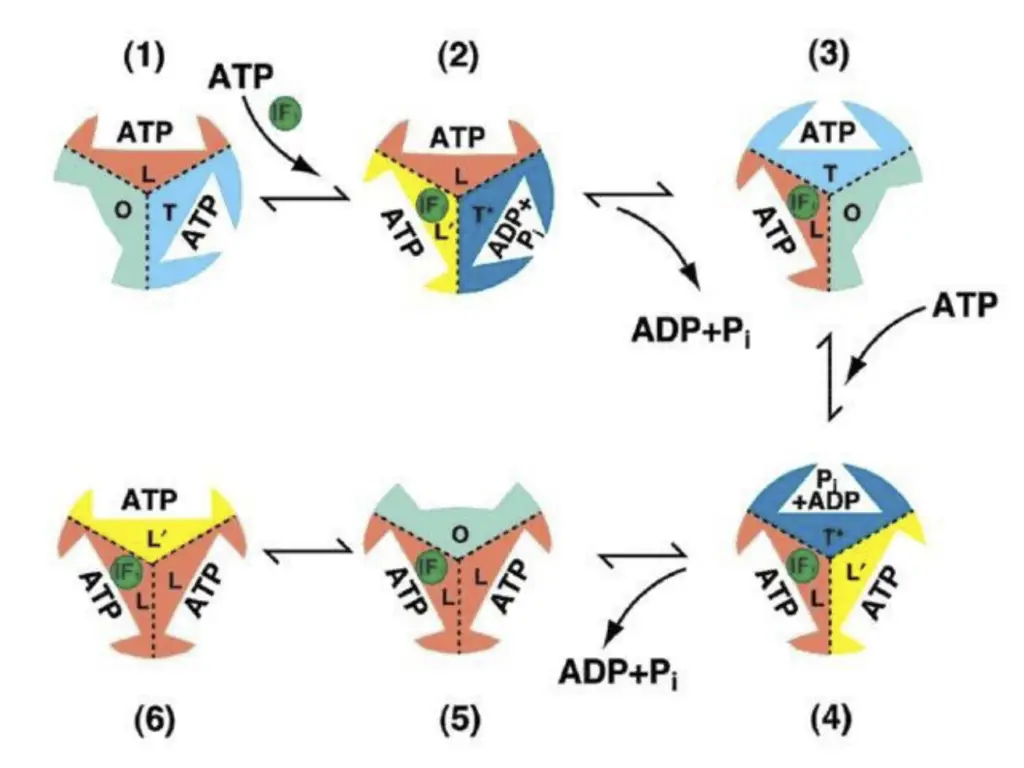
- Diarylquinolines:
- TMC207 (Bedaquiline): Developed for tuberculosis treatment, this drug inhibits ATP synthase by targeting subunit c of the enzyme in Mycobacterium tuberculosis, blocking ATP production.
- Bz-423:
- A pro-apoptotic 1,4-benzodiazepine derivative, Bz-423 binds the oligomycin sensitivity conferring protein (OSCP), promoting superoxide generation and triggering apoptosis.
- Melittin:
- A cationic, amphiphilic polypeptide, melittin inhibits the catalytic activity of ATP synthase in mitochondria and chloroplasts, disrupting energy metabolism.
- IF1 (Endogenous Inhibitor):
- IF1 locks ATP in the catalytic site of ATP synthase, preventing its hydrolysis and effectively reducing ATP production during conditions of low energy demand.
- Oligomycin:
- An antibiotic that inhibits ATP synthase by blocking the F0 subunit, which halts proton re-entry into the mitochondria, disrupting the electron transport chain and ceasing proton pump activity.
- Polyphenolic Phytochemicals:
- Resveratrol and Genistein: These non-competitive inhibitors of the F0F1-ATPase act by inhibiting ATP synthesis without affecting oxidative phosphorylation.
- Quercetin: Inhibits multiple ATPases, including Na+/K+-ATPase and Ca2+-ATPase, with a notable inhibitory effect on mitochondrial ATPase at lower concentrations.
- Plant-derived Inhibitors:
- Polyphenols and flavones are effective inhibitors of the F0F1-ATPase in bovine and porcine hearts.
- Efrapeptins:
- These peptides, produced by Tolypocladium fungi, inhibit mitochondrial ATPase by targeting the α, β, and γ subunits of F1 particles, competitively inhibiting both ATP hydrolysis and synthesis.
- Piceatannol:
- A stilbenoid compound, piceatannol inhibits F-type ATPase, specifically targeting the F1 subunit to prevent ATP synthesis.
- Bicarbonate:
- Bicarbonate enhances ATP hydrolysis but inhibits ATP synthesis by increasing ATP’s affinity for the catalytic site, demonstrating competitive inhibition relative to ADP and phosphate.
- Other Inhibitors:
- Tentoxin: A phytotoxin that binds between the α and β subunits of chloroplast ATP synthase, inhibiting its activity.
- Leucinostatins: These bind to the F0 portion of ATP synthase, inhibiting oxidative phosphorylation in mitochondria and photophosphorylation in chloroplasts.
- Fluoro-aluminate: Inhibits ATPase by binding with ADP, freezing the enzyme in an intermediate state of ATP hydrolysis/synthesis.
- Dicyclohexylcarbodiimide (DCCD): Reacts with the conserved acidic amino acids in subunit c of F0 ATPase, blocking its function, and also modifies carboxyl groups in F1 ATPase at lower pH.
- Azide: Inhibits mitochondrial F1 ATPase by binding with MgADP, preventing ADP release without affecting ATP synthesis.
- Additional Inhibitors:
- Substances such as magnesium, bismuth subcitrate, omeprazole, ethidium bromide, adenylyl imidodiphosphate, arsenate, and angiostatin are also known to inhibit ATP synthase. These compounds may act through various mechanisms, affecting enzyme activity, proton gradients, or electron transport processes.
- Jonckheere AI, Smeitink JA, Rodenburg RJ. Mitochondrial ATP synthase: architecture, function and pathology. J Inherit Metab Dis. 2012 Mar;35(2):211-25. doi: 10.1007/s10545-011-9382-9. Epub 2011 Aug 27. PMID: 21874297; PMCID: PMC3278611.
- Neupane, Prashant, Bhuju, Sudina, Thapa, Nita and Bhattarai, Hitesh Kumar. “ATP Synthase: Structure, Function and Inhibition” Biomolecular Concepts, vol. 10, no. 1, 2019, pp. 1-10. https://doi.org/10.1515/bmc-2019-0001
- https://biologyreader.com/atp-synthase-in-photosynthesis.html
- https://www.deshbandhucollege.ac.in/pdf/resources/1586196122_BOT(H)VI_Sem-Week-3.pdf
- https://wou.edu/chemistry/files/2020/03/ATP-Synthase-Enzyme-PDF-with-Notes.pdf