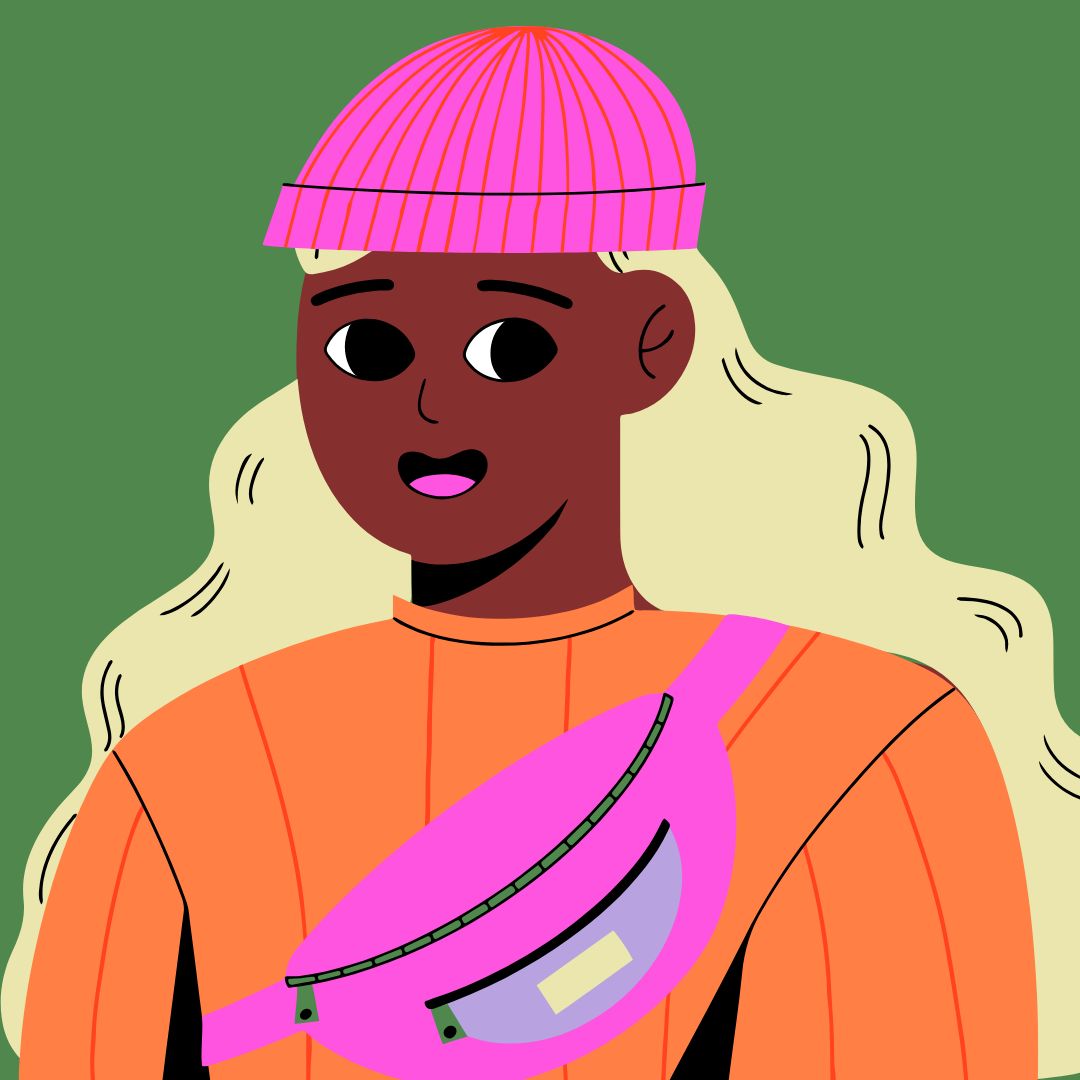
Sourav Pan
Transcript
Welcome to our introduction to feedback mechanisms.
Feedback mechanisms are regulatory systems that respond to changes in an environment or process.
At their core, all feedback mechanisms follow the same basic pattern: they detect a change, process the information, and then respond accordingly.
These mechanisms operate in a continuous cycle, constantly monitoring and adjusting to maintain balance.
Feedback mechanisms are found in diverse domains beyond biology, including technology, economics, and social systems.
These regulatory systems are fundamental to maintaining stability, enabling essential life processes, helping systems adapt to changes, and preventing system failure.
In summary, feedback mechanisms are essential components of virtually all stable, functional systems, from the human body to complex technological and social structures.
Homeostasis is the maintenance of stable internal conditions necessary for an organism’s survival.
This stability of internal conditions is crucial even when the external environment undergoes significant changes.
The external environment constantly changes – temperature fluctuates, pH levels vary, and nutrient availability shifts.
Despite these external changes, organisms maintain stable internal conditions through feedback mechanisms.
Let’s look at three key examples of homeostasis in the human body.
Body temperature is maintained around 37 degrees Celsius, even in varying environmental temperatures.
Blood pH is tightly regulated between 7.35 and 7.45, which is critical for enzyme function and oxygen transport.
Blood glucose levels are maintained between 70 and 100 milligrams per deciliter, ensuring a constant energy supply to cells.
Homeostasis is vital for multiple reasons. It ensures proper cell function, optimal enzyme activity, efficient metabolic processes, and ultimately survival in changing environments.
Negative feedback is a mechanism that counteracts or reverses changes to restore equilibrium in a system.
When a variable moves away from its set point, negative feedback works to bring it back.
Negative feedback has several key characteristics that make it essential for biological systems.
A typical negative feedback loop consists of three main components: a sensor that detects changes, a control center that processes the information, and an effector that responds to correct the deviation.
Negative feedback promotes stability and is the more common type in biological systems, serving as the primary mechanism for maintaining homeostasis in living organisms.
Let’s examine the process of negative feedback, which is fundamental to maintaining homeostasis in biological systems.
Negative feedback operates as a cycle with four key steps that work together to counteract changes and maintain stability.
Let’s break down these four steps of the negative feedback process.
Step one: A stimulus creates change. This occurs when a regulated variable deviates from its normal range or set point.
Step two: Receptors detect this change. Specialized sensory receptors monitor the variable and identify when it moves away from the ideal range.
Step three: A control center processes this information. This could be the brain, endocrine glands, or other regulatory systems that interpret signals from the receptors.
Step four: Effectors produce a response that counteracts the initial change. This critical step returns the variable back toward its normal range.
This completes the negative feedback loop. The process continues cyclically to maintain homeostasis, with the effector response reducing the initial stimulus.
The key characteristic of negative feedback is that it opposes or negates changes, which is essential for maintaining stable internal conditions.
Positive feedback loops amplify changes within a system, pushing it away from its initial state.
The process of positive feedback follows four distinct steps.
Step one: A stimulus creates an initial change in the system.
Step two: Unlike negative feedback, the system responds by amplifying this change rather than counteracting it.
Step three: The amplified change triggers even further amplification, intensifying the original deviation.
Step four: This cycle continues, with each iteration increasing the magnitude of the response, until an endpoint or limit is finally reached.
This escalating cycle creates a reinforcing loop, pushing the system further and further from its original state.
Unlike negative feedback, which maintains stability, positive feedback amplifies change in the same direction as the initial stimulus.
Positive feedback loops can be found in many biological processes, including blood clotting, childbirth contractions, and fruit ripening.
An important characteristic of positive feedback is that it must have an endpoint. Without a stopping mechanism, the system would continue to amplify until it overloads or breaks down.
The human body maintains its temperature through a highly effective negative feedback system.
Temperature is detected by two types of receptors: thermoreceptors in your skin that sense external temperature changes, and receptors in your hypothalamus that monitor your internal body temperature.
This temperature information is sent to the brain, specifically the hypothalamus, which acts as your body’s thermostat. The hypothalamus compares your current body temperature to the normal setpoint of approximately 37 degrees Celsius.
If your body temperature is too high, the brain activates cooling mechanisms. Sweat glands produce sweat to cool the skin through evaporation, and blood vessels dilate to bring warm blood to the surface where heat can be released.
Conversely, if your body temperature is too low, the brain triggers warming mechanisms. Muscles rapidly contract causing shivering to generate heat, and blood vessels constrict to keep warm blood closer to vital organs.
These responses continue until body temperature returns to normal, completing the negative feedback loop. This stabilizing mechanism ensures that your core temperature stays within the narrow range needed for optimal biological function.
Body temperature regulation exemplifies the key characteristics of negative feedback systems: it counteracts deviations from normal, is self-limiting, and maintains stability through opposing responses.
This temperature regulation system is a perfect example of how negative feedback mechanisms are essential for maintaining homeostasis in biological systems.
Fruit ripening provides an excellent example of a positive feedback loop in biological systems.
As fruit ripens, it undergoes various changes in color, texture, and chemical composition.
A key component of this process is the production of ethylene gas, a plant hormone that triggers and accelerates ripening.
What makes fruit ripening a positive feedback process is that as fruit begins to ripen, it produces ethylene, which triggers even more ripening.
This creates a circular process where more ripening leads to more ethylene production, which in turn leads to even more ripening.
This positive feedback mechanism explains the famous saying that ‘one bad apple spoils the bunch.’
When one apple in a group begins to overripen, it releases large amounts of ethylene gas. This ethylene triggers ripening in the surrounding fruit.
As the surrounding apples ripen, they too produce more ethylene, creating a cascade effect that accelerates ripening throughout the entire bunch.
From an evolutionary perspective, this positive feedback system ensures that fruits ripen fully and simultaneously, which has important benefits for seed dispersal.
When fruit is optimally ripe, it becomes more attractive to animals through changes in color, sweetness, aroma, and texture.
Animals that eat the ripe fruit help disperse the seeds through their digestive systems, depositing them in new locations with their droppings.
The synchronized ripening triggered by the positive feedback loop ensures that seeds are dispersed at the optimal time, when they’re fully developed and ready to germinate in a new location.
Fruit ripening is thus a perfect example of how positive feedback mechanisms in nature can serve important biological functions, in this case ensuring effective seed dispersal and plant reproduction.
The nervous system uses complex feedback mechanisms to maintain balance and respond to changes.
The nervous system relies on two key feedback mechanisms: excitation and inhibition.
Excitatory feedback amplifies neural signals, increasing activity in a pathway. Inhibitory feedback reduces neural signals, dampening activity.
Reflexes represent one of the simplest feedback loops in the nervous system.
In a reflex arc, sensory receptors detect a stimulus and send signals through sensory neurons to interneurons in the spinal cord.
The interneurons relay the signal to motor neurons, which activate effector organs like muscles to produce a response.
This creates a negative feedback loop that automatically adjusts the response without conscious thought.
Beyond simple reflexes, the nervous system uses complex feedback networks for higher functions.
Lateral inhibition is a key example of neural feedback. When one neuron is activated, it inhibits its neighbors.
This creates contrast enhancement in sensory processing, sharpening our perception of edges and boundaries.
To summarize, neural feedback mechanisms occur at all levels of the nervous system, from simple reflexes to complex cognitive networks.
Both simple reflexes and complex networks rely on feedback for proper functioning. This enables the nervous system to adapt, learn, and maintain stability.
Feedback mechanisms are fundamental to technological systems, adapting principles found in biological systems.
Technological feedback systems, like their biological counterparts, consist of three key components: sensors, processors, and effectors.
Sensors detect changes in the environment or system. Processors analyze this information and make decisions. Effectors then respond by making adjustments to the system.
A home thermostat is a classic example of negative feedback in technology. The thermometer constantly monitors room temperature.
The control circuit compares the measured temperature with the desired setpoint. When the temperature deviates from the setpoint, the heating or cooling system activates.
This creates a negative feedback loop, as the system works to counteract any deviation from the setpoint, maintaining a stable temperature.
Cruise control in cars is another example of negative feedback. The speedometer constantly monitors the vehicle’s speed.
The car’s computer compares actual speed with the set speed. The throttle control then adjusts engine power to maintain the desired speed.
This negative feedback system automatically compensates for hills, wind resistance, or other factors that would otherwise change the car’s speed.
Automated manufacturing systems incorporate both negative and positive feedback loops. Quality control systems use negative feedback to detect and correct defects.
Meanwhile, process optimization systems use positive feedback to identify successful operations and amplify them, continuously improving efficiency.
Technological feedback systems parallel their biological counterparts, using similar components to achieve control and stability.
Where biological systems use receptors and nerve endings as sensors, technological systems employ devices like thermometers and cameras.
The brain and glands process information in biological systems, while computers and circuits serve this function in technology.
Finally, muscles and organs act as biological effectors, compared to motors and valves in technological systems.
When feedback mechanisms fail, critical biological systems become dysregulated.
In normal feedback systems, sensors detect changes, control centers process information, and effectors respond appropriately.
However, when feedback mechanisms fail, the system breaks down. This often occurs at the control center or the effector response.
When feedback mechanisms fail, homeostasis is disrupted, leading to various disease states.
Diabetes is a prime example of feedback failure. In normal glucose regulation, the pancreas detects high blood glucose and releases insulin.
Insulin signals body cells to take up glucose from the bloodstream, reducing blood glucose levels in a classic negative feedback loop.
In diabetes, this feedback loop breaks down. There are two main types of diabetes, each representing a different failure point in the feedback mechanism.
In Type 1 diabetes, the pancreas fails to produce sufficient insulin. The control center itself is damaged, often due to autoimmune destruction of insulin-producing cells.
In Type 2 diabetes, the body’s cells become resistant to insulin signals. Despite normal or even increased insulin production, the effectors fail to respond properly.
Both types result in chronically elevated blood glucose, causing damage to blood vessels, nerves, and organs over time.
Cancer provides another clear example of feedback failure. In normal cells, multiple checkpoints control cell division.
These checkpoints verify DNA integrity and ensure cells only divide when necessary. They act as a negative feedback system to prevent excessive proliferation.
In cancer, genetic mutations disable these checkpoint systems. The negative feedback that normally prevents excessive cell division fails.
Without functional checkpoints, cells proliferate uncontrollably, forming tumors and potentially spreading throughout the body.
Understanding how feedback mechanisms fail is crucial for developing effective medical treatments.
For diabetes, treatment strategies aim to restore proper glucose regulation. Insulin therapy directly replaces the missing feedback signal in Type 1 diabetes.
For cancer, modern targeted therapies aim to restore cellular growth control by inhibiting specific pathways that have lost their normal regulatory function.
The key principle in treating feedback disorders is to either restore the regulatory mechanisms or compensate for their absence with external interventions.
Feedback mechanisms have evolved over billions of years, becoming increasingly sophisticated as organisms adapted to diverse environments.
Early single-celled organisms developed primitive feedback mechanisms around four billion years ago. These simple systems regulated basic cellular processes like metabolism.
As eukaryotic cells evolved, their compartmentalized structure allowed for more complex, multiple feedback systems controlling different cellular functions.
The emergence of multicellular life enabled coordinated feedback mechanisms across specialized cells, creating system-level regulation.
Complex animals developed organ systems with dedicated feedback loops. These systems could operate independently but also coordinate with each other.
Modern mammals have highly integrated feedback networks across multiple systems, allowing precise regulation in varied environments.
Natural selection plays a critical role in the evolution of feedback mechanisms. Organisms with more effective regulatory systems have better survival rates in changing environments.
Feedback mechanisms have increased in complexity through distinct evolutionary stages, from simple on/off responses to sophisticated predictive systems.
These evolved feedback systems allow organisms to adapt to varied environments. More sophisticated regulation enables species to colonize new habitats and exploit diverse resources.
The evolutionary history of feedback mechanisms shows a clear trend toward increasing complexity and integration, enabling organisms to thrive in ever more challenging environments.
Social systems use feedback mechanisms to regulate behaviors and maintain social order.
Within groups, individuals influence each other’s behaviors through complex feedback networks.
Social reinforcement serves as a feedback mechanism that shapes behavior.
It involves responses from others that influence whether we repeat certain behaviors.
Positive reinforcement includes praise, social media likes, and acceptance into groups.
While negative reinforcement involves criticism, social rejection, and group ostracism.
Positive feedback in social systems amplifies behaviors, often leading to conformity.
This creates a self-reinforcing cycle that strengthens group norms and encourages conformity.
We see examples of positive feedback in fashion trends, viral content, mob behavior, and peer pressure.
Negative feedback in social systems detects deviations from norms and implements corrections.
This creates a stabilizing cycle that maintains social order by bringing behaviors back in line with expectations.
Examples include social disapproval for norm violations, legal punishment, parental correction, and workplace feedback.
Let’s compare the different types of social feedback mechanisms.
Positive feedback tends to amplify changes, creating conformity and trends, but can lead to system instability.
While negative feedback reduces change, maintaining stability and social order through homeostasis.
The key concept is that healthy social systems require both types of feedback – positive feedback for innovation and change, and negative feedback for stability and order.
Engineering feedback systems are artificial control mechanisms that monitor and adjust based on system outputs.
These systems apply concepts from biology to technology, helping to maintain stability and optimize performance in various applications.
Control theory is the mathematical foundation of engineering feedback systems.
A typical control system has four key components: a setpoint that defines the desired state, a controller that processes error signals, the system itself, and sensors that measure outputs.
These systems continuously monitor outputs and adjust inputs to maintain the desired state. They use mathematical models to detect and correct errors, forming the basis for all feedback control.
PID controllers are the most widely used feedback controllers in engineering systems.
PID stands for Proportional, Integral, and Derivative – three mathematical operations that work together to achieve precise control.
The Proportional component responds to current error. The Integral component addresses accumulated error over time. And the Derivative component anticipates future error based on rate of change.
PID controllers are essential in many applications including temperature control systems, drone flight stabilization, and precise robotic movements.
Understanding biological feedback mechanisms has inspired numerous technological innovations.
In robotics, engineers use feedback systems inspired by human balance and movement to create machines that can navigate complex environments.
Artificial intelligence systems employ neural networks with feedback mechanisms similar to those in the brain, allowing them to learn from experience.
Sustainable design incorporates principles from natural ecosystems, using negative feedback to maintain balance and efficiency.
These bio-inspired technologies have led to real-world applications including self-balancing robots based on the human vestibular system, machine learning algorithms that improve through reinforcement feedback, and sustainable building systems that mimic natural homeostasis.
Looking to the future, engineering feedback systems are evolving toward greater autonomy and sophistication.
Engineers are developing self-adaptive systems with minimal human intervention, more sophisticated bio-inspired algorithms, and integrated feedback mechanisms that combine multiple control strategies.
Perhaps most exciting is the emergence of quantum feedback systems, which promise unprecedented precision in controlling complex processes.
Understanding the balance between feedback mechanisms is crucial for biological systems.
Balanced feedback systems incorporate both negative and positive feedback mechanisms. This balance is critical for optimal functioning.
Systems need both stability through negative feedback and adaptability through positive feedback. Stability maintains consistent functioning, while adaptability enables necessary changes when conditions demand it.
Understanding feedback balance helps us comprehend, predict, and potentially control complex systems in biology, technology, and society. This knowledge allows us to design more effective systems and anticipate their behaviors.
Remember, balance is the key to effective feedback systems. Neither type alone is sufficient for optimal functioning.
Study Materials
No study materials available for this video.
Helpful: 0%
Related Videos
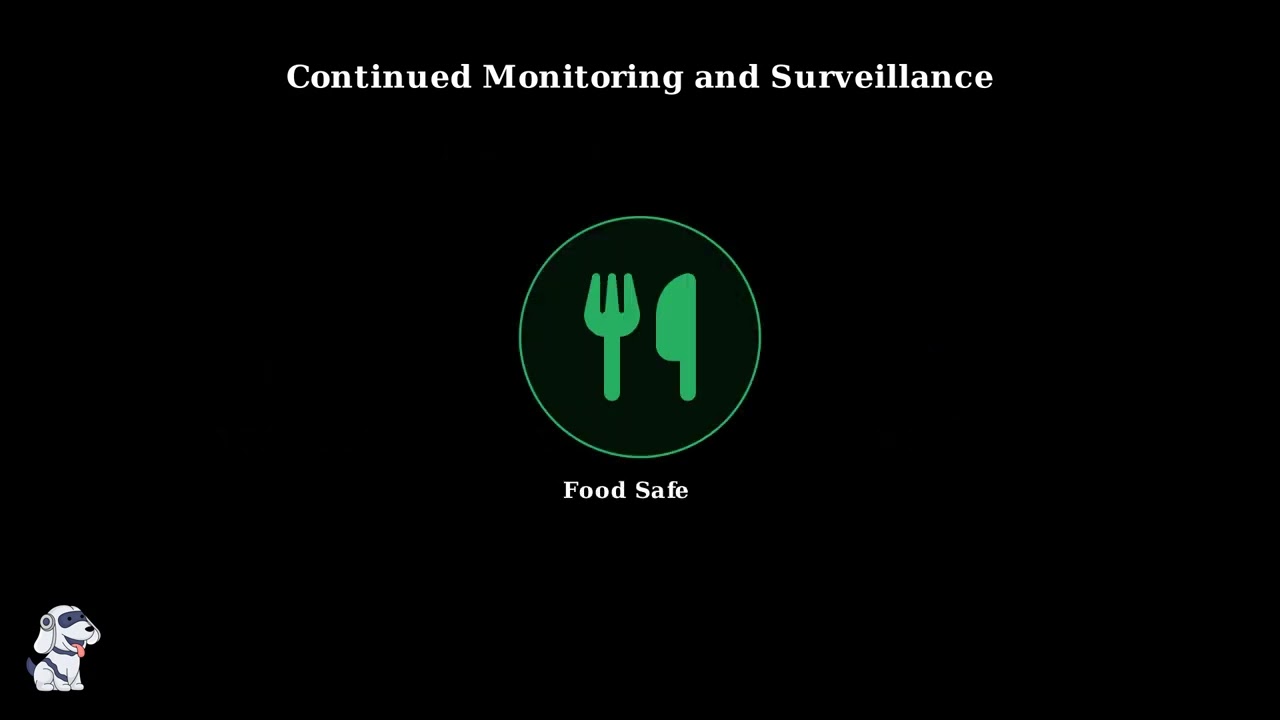
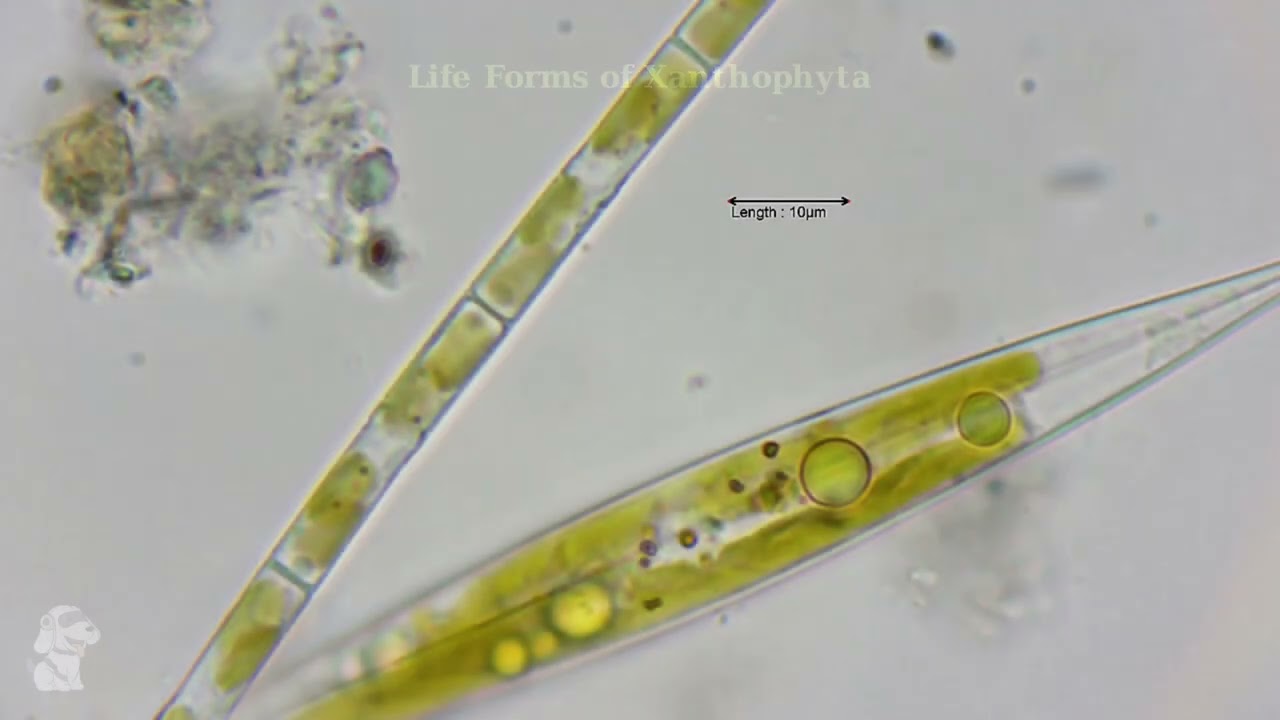
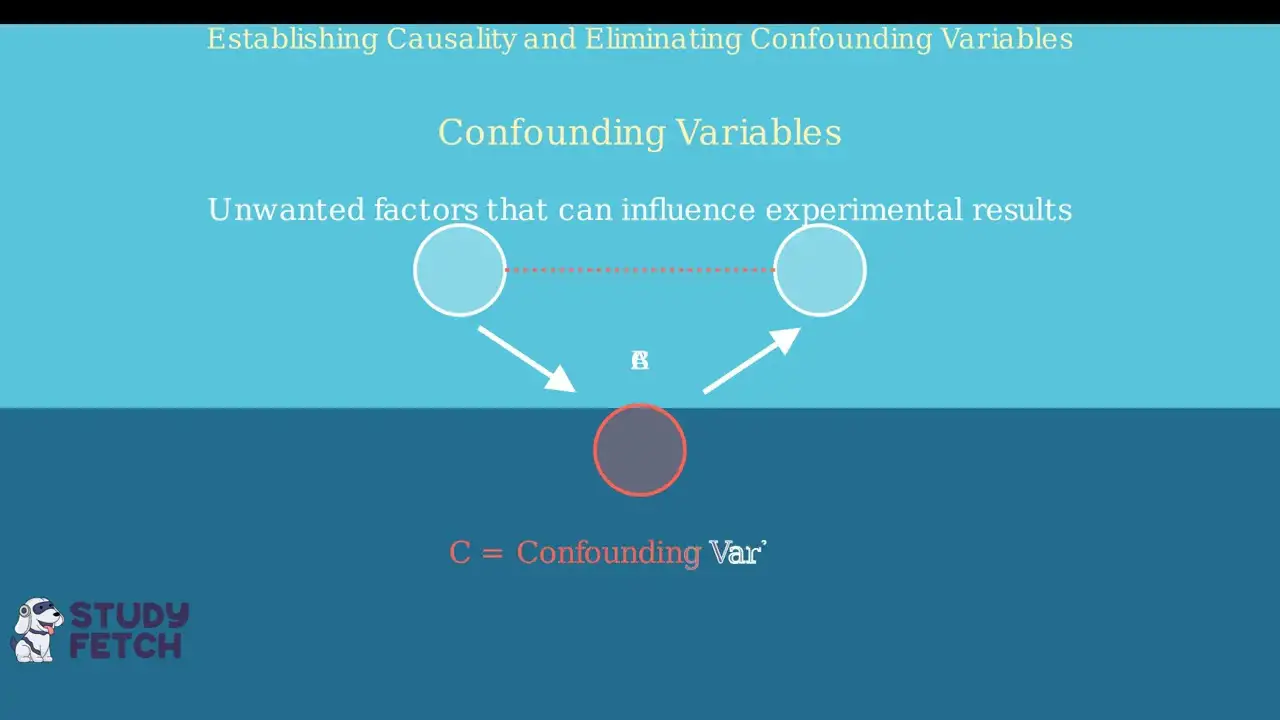
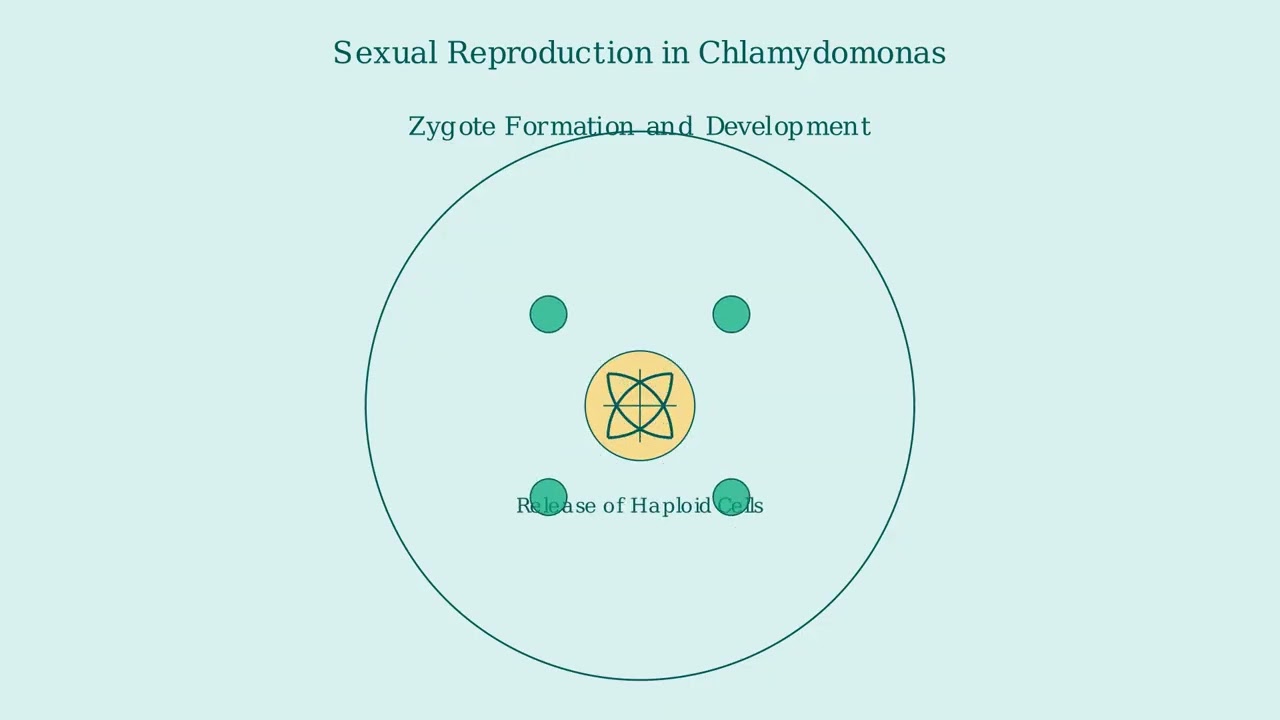
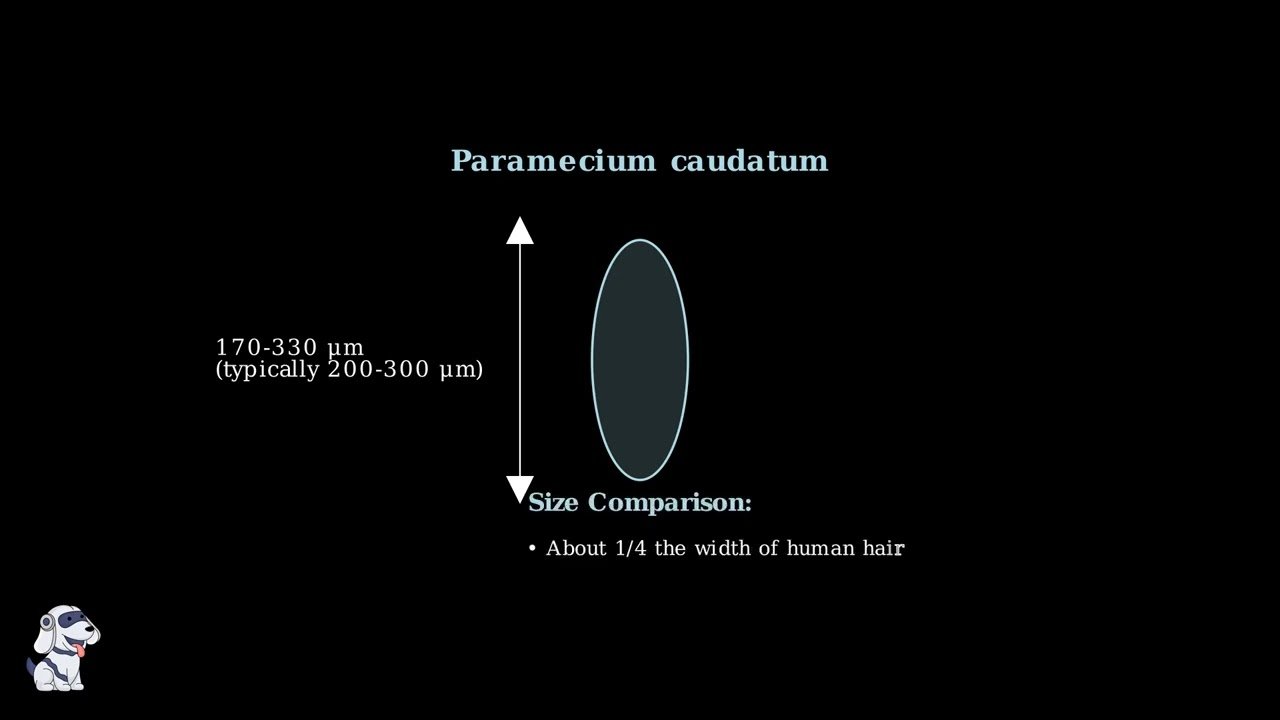
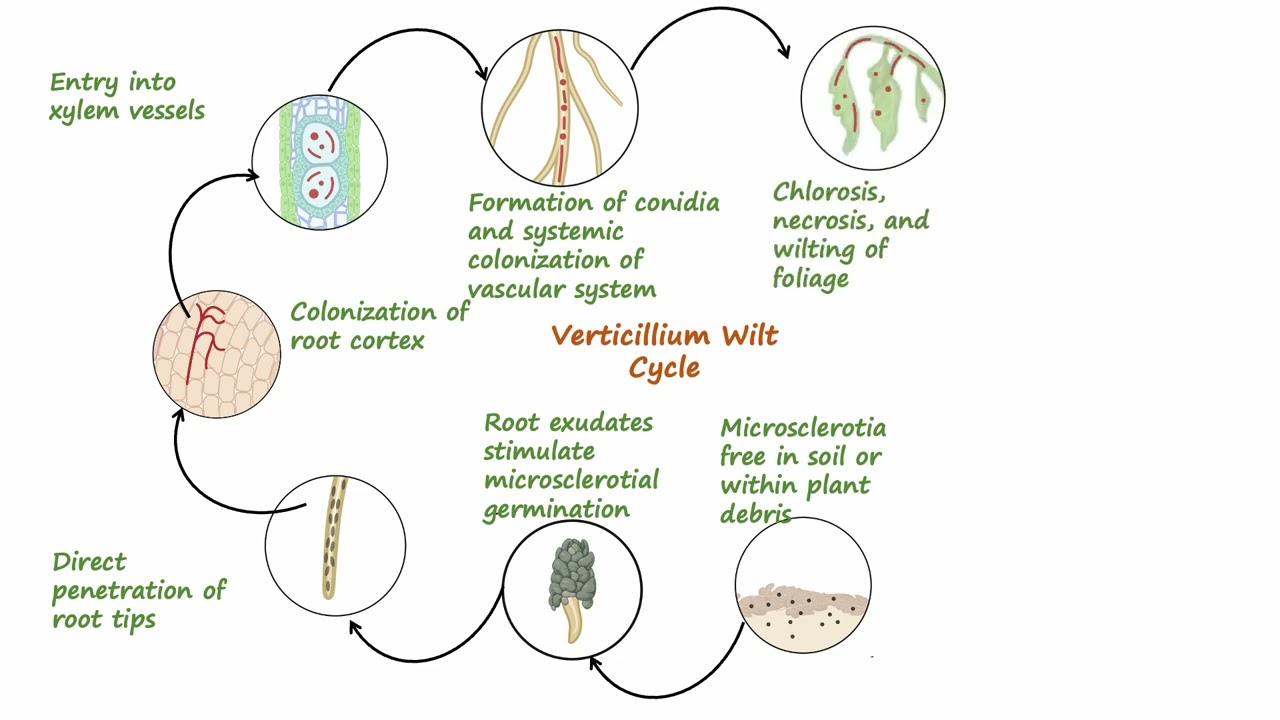
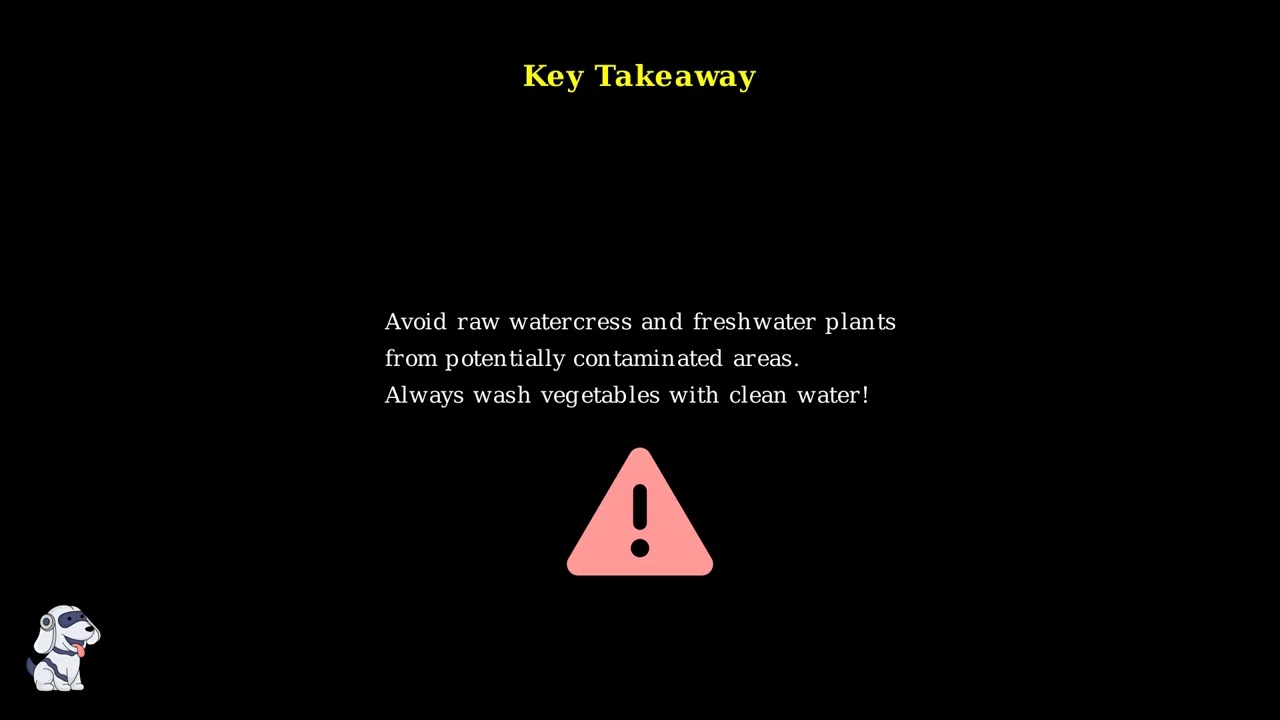
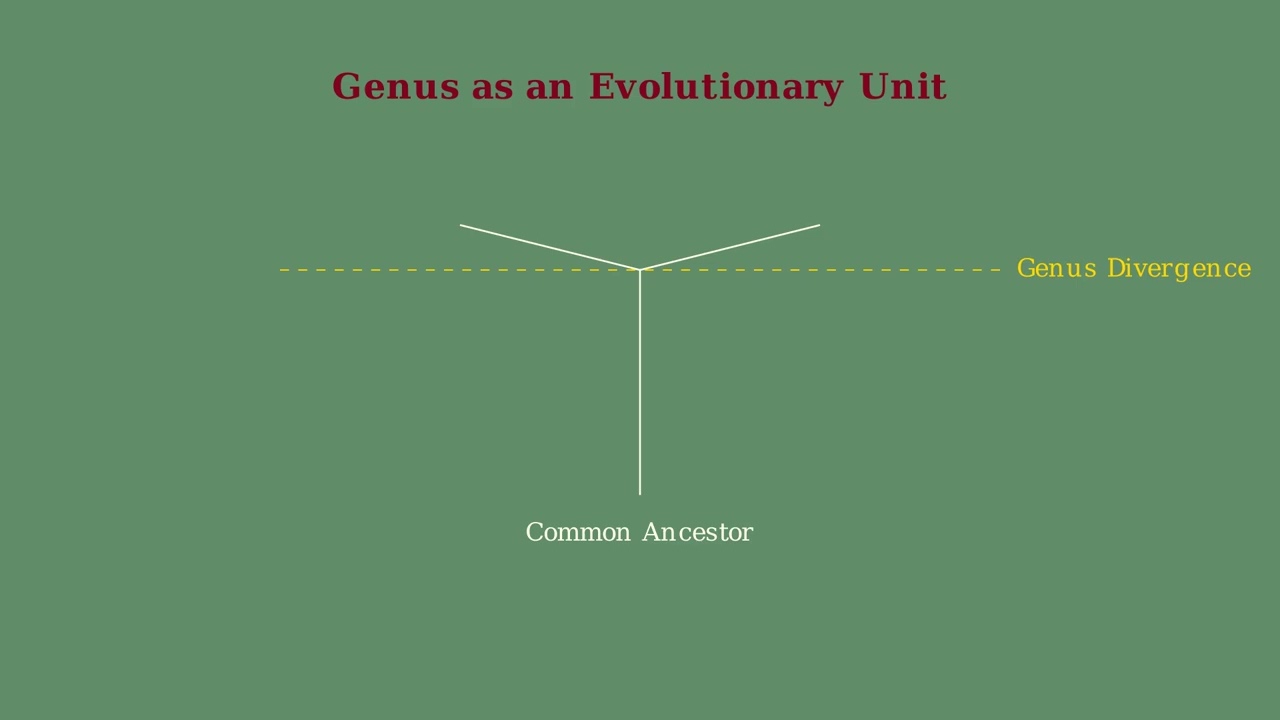
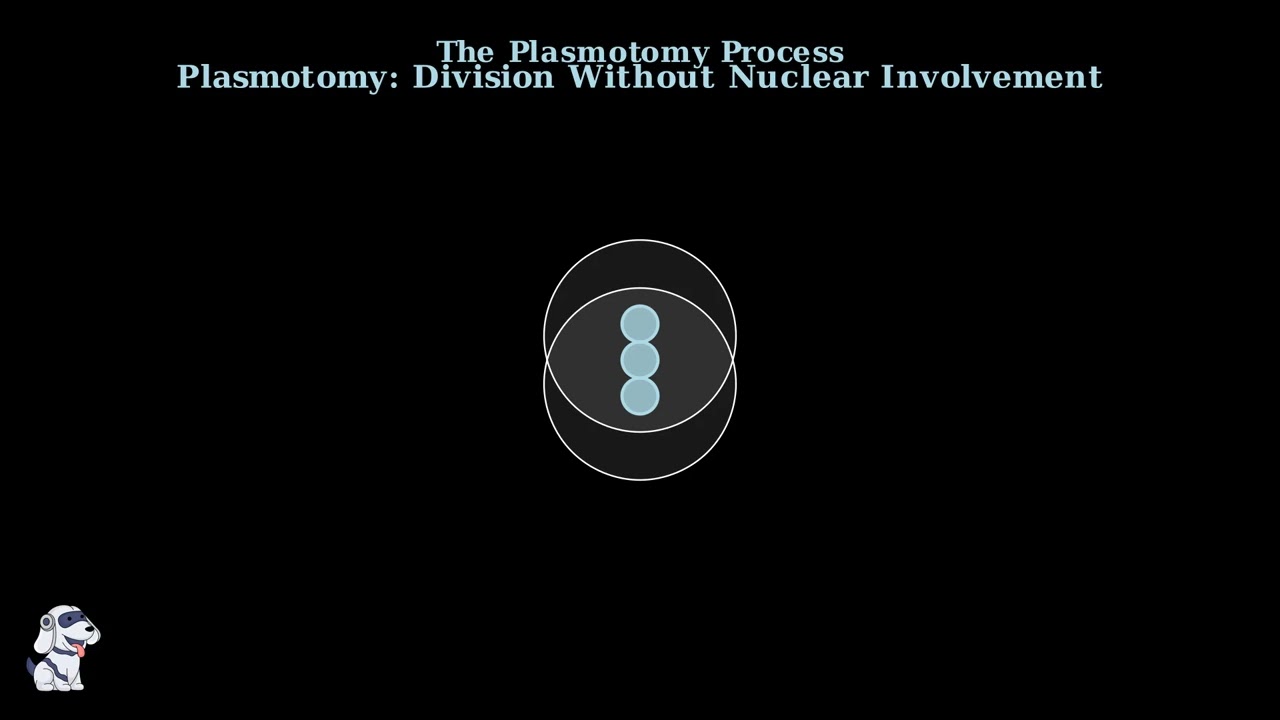