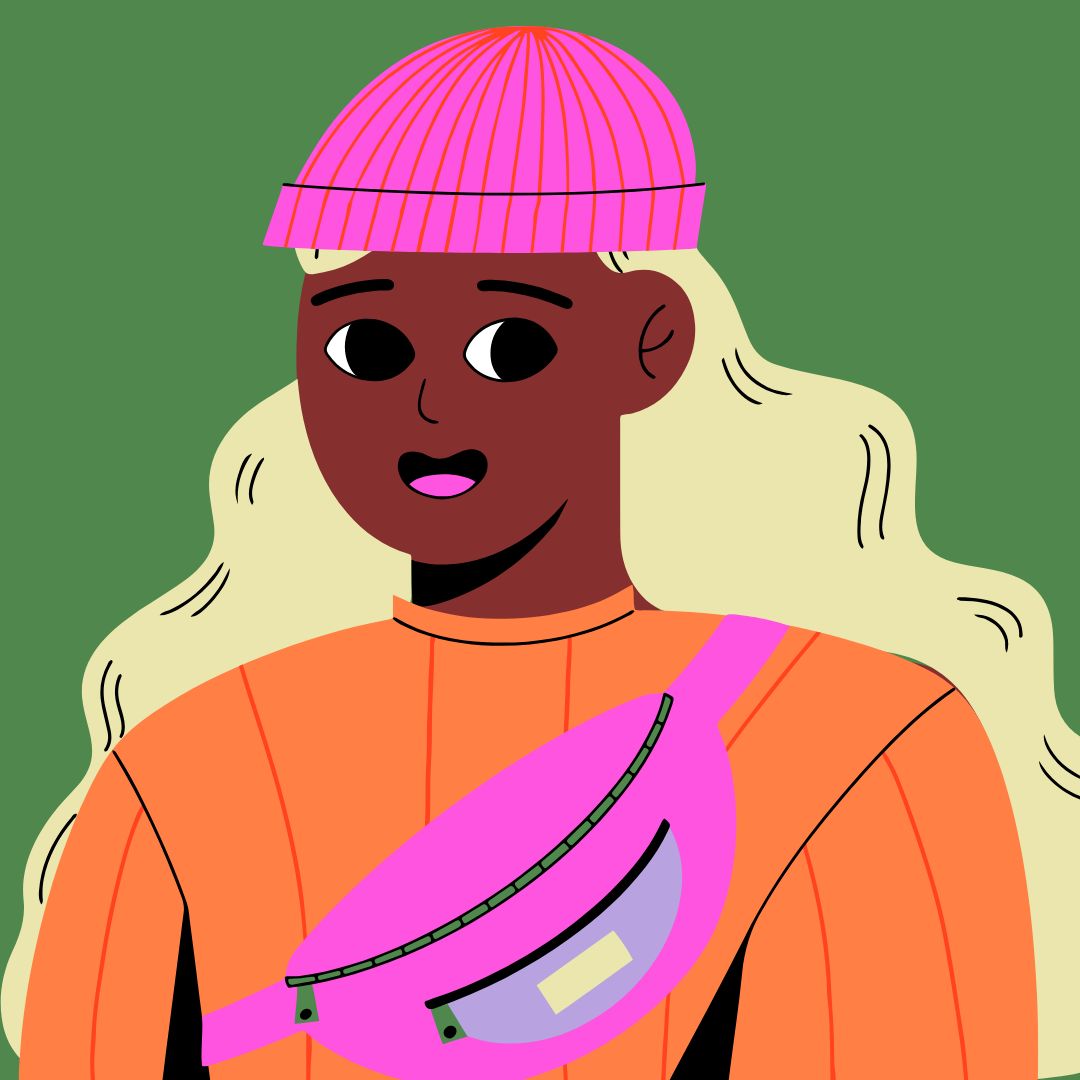
Sourav Pan
Transcript
Concentration gradients are fundamental to understanding facilitated diffusion.
A concentration gradient exists when the concentration of molecules differs between two adjacent areas.
Let’s visualize a cell membrane with a high concentration of molecules on one side and a low concentration on the other.
The membrane contains transport proteins that allow specific molecules to pass through in a process called facilitated diffusion.
According to the concentration gradient, molecules naturally move from areas of high concentration to areas of low concentration.
This movement follows the second law of thermodynamics, which states that systems tend toward maximum entropy or disorder.
A key feature of facilitated diffusion is that it requires no energy input. The movement is driven by the concentration gradient alone, making it a passive transport process.
Transport proteins are the key components that enable facilitated diffusion across cell membranes.
The cell membrane consists of a phospholipid bilayer that forms a barrier to most molecules.
Transport proteins have several important features that allow them to facilitate the movement of specific molecules.
These specialized proteins are embedded directly within the cell membrane.
Each transport protein has specific binding sites that recognize and bind to particular molecules.
After binding, transport proteins undergo a conformational change, altering their shape to move substances across the membrane.
This elegant mechanism allows molecules to pass through the membrane without disrupting its structural integrity, which is crucial for cell survival.
Transport proteins are essential components that enable cells to precisely control which molecules can enter and exit, making facilitated diffusion possible.
Channel proteins are specialized transport proteins that create water-filled passageways through the cell membrane.
Unlike carrier proteins, channel proteins form permanent pores or tunnels that allow molecules to flow through the membrane.
Channel proteins are highly selective, allowing only specific types of molecules to pass through.
This selectivity is based primarily on the size of the molecules. Small molecules can pass through, while larger ones cannot.
Additionally, many channels are selective based on the electrical charge of molecules.
Let’s look at two important examples of channel proteins found in cell membranes.
Aquaporins are specialized channel proteins that allow water molecules to pass through the membrane efficiently.
These channels are so efficient that billions of water molecules can pass through a single aquaporin each second.
Ion channels are another important type of channel protein that allow specific ions to pass through the membrane.
These channels are highly selective for specific ions such as potassium, sodium, calcium, or chloride. For example, a potassium channel allows potassium ions to pass while blocking other ions.
It’s important to note that many channel proteins are not always open. They can be regulated by gates that respond to different stimuli.
Carrier proteins facilitate the movement of specific molecules across the cell membrane through a unique mechanism.
Unlike channel proteins, carrier proteins undergo significant conformational changes during transport.
Let’s examine how glucose transporters, or GLUT proteins, move glucose across the membrane.
First, the carrier protein has a specific binding site that recognizes glucose molecules.
After glucose binds, the carrier protein undergoes a conformational change, rotating to expose the binding site to the opposite side of the membrane.
The glucose molecule is then released into the intracellular environment due to the lower affinity of the binding site in this new conformation.
Finally, the empty carrier protein returns to its original conformation, ready to transport another glucose molecule.
GLUT proteins are a family of glucose transporters with several key features.
Glucose transport across cell membranes is a classic example of facilitated diffusion in action.
Glucose is a vital energy source for cells, but it faces a challenge: it’s a relatively large, polar molecule that cannot cross the hydrophobic cell membrane on its own.
Without assistance, glucose would be unable to enter cells efficiently, despite the concentration gradient driving it inward.
This is where GLUT proteins come in. GLUT stands for glucose transporter, a family of specialized transmembrane proteins that create channels specifically for glucose to pass through.
The transport mechanism involves a series of steps. First, glucose binds to a specific site on the GLUT protein. This causes the protein to change shape, moving the glucose through the membrane. Finally, glucose is released into the cell, and the protein returns to its original conformation.
Different cell types have specialized GLUT proteins based on their glucose needs. GLUT1 is abundant in red blood cells and the brain, ensuring a constant supply of glucose. GLUT2 in the liver and pancreas helps with glucose sensing. GLUT4, found in muscle and fat cells, is unique because it responds to insulin, moving to the cell membrane only when insulin is present.
The efficient transport of glucose is critical for cellular energy production. Once inside the cell, glucose enters the glycolysis pathway, eventually producing ATP, the energy currency of cells. This energy powers everything from muscle contraction to neuron firing to cell division.
Understanding glucose transport has important clinical implications. In diabetes, GLUT4 proteins fail to respond properly to insulin, preventing glucose uptake in muscle and fat tissues. GLUT1 deficiency can cause seizures and developmental delays due to insufficient glucose in the brain. Cancer cells often increase GLUT expression to fuel their rapid growth.
In summary, glucose transport via GLUT proteins is a perfect illustration of facilitated diffusion in action, demonstrating how cells have evolved elegant solutions to move essential molecules across membrane barriers.
Ion channels are specialized membrane proteins that facilitate the diffusion of specific ions across the cell membrane.
These channels are highly selective, allowing only specific ions such as sodium, potassium, or calcium to pass through.
Many ion channels are gated, meaning they can open and close in response to specific stimuli.
There are three main types of gated channels: voltage-gated channels respond to electrical signals, ligand-gated channels respond to chemical messengers, and mechanically-gated channels respond to physical forces.
One of the most important examples of ion channels in action is the generation of action potentials in neurons.
Neurons contain voltage-gated sodium and potassium channels that open and close at different times to generate electrical signals.
During an action potential, sodium channels open first, allowing sodium ions to rush into the cell, causing depolarization.
Then potassium channels open, allowing potassium ions to exit the cell, causing repolarization and a return to the resting state.
To summarize, ion channels are specialized proteins that facilitate the diffusion of specific ions across cell membranes. They’re critical for neuronal signaling, muscle contraction, and many other biological functions.
Several key factors influence the rate of facilitated diffusion across cell membranes.
Let’s examine the four main factors that determine how quickly molecules move through transport proteins.
The steepness of the concentration gradient is a primary factor affecting diffusion rate.
A steeper concentration gradient – meaning a greater difference in molecule concentration between the two sides of the membrane – results in faster diffusion.
The second factor is the number of transport proteins in the membrane.
Membranes with more transport proteins allow for faster facilitated diffusion.
With more proteins, more molecules can pass through simultaneously, increasing the overall rate of diffusion.
Temperature is the third major factor affecting facilitated diffusion rates.
Higher temperatures increase the kinetic energy of molecules, causing them to move more rapidly.
This increased molecular motion leads to more frequent collisions with transport proteins, accelerating the rate of facilitated diffusion.
The fourth factor is molecular specificity, which refers to how well molecules match the specific transport proteins.
Transport proteins are highly selective, only allowing molecules with the correct size and shape to pass through.
Molecules that match the transport protein’s binding site can pass through, while others cannot, regardless of the concentration gradient.
To summarize, four main factors determine the rate of facilitated diffusion across cell membranes.
First, the steepness of the concentration gradient drives the direction and speed of diffusion.
Second, the number of transport proteins in the membrane determines how many molecules can pass through simultaneously.
Third, temperature affects molecular kinetic energy and movement speed.
And fourth, molecular specificity ensures only compatible molecules can utilize specific transport proteins.
Understanding these factors helps explain how cells regulate the movement of essential molecules across their membranes.
pH plays a crucial role in facilitated diffusion by affecting the structure and function of transport proteins.
Most transport proteins have an optimal pH range where they function most efficiently. This is typically around neutral pH for many cellular proteins.
At optimal pH, the protein’s structure allows molecules to pass through efficiently via facilitated diffusion.
Transport efficiency follows a bell curve relationship with pH, with maximum efficiency at the protein’s optimal pH range.
When pH changes, it affects the ionization state of amino acids in the protein, altering hydrogen bonds and electrostatic interactions.
In acidic environments, the increased concentration of hydrogen ions can protonate negatively charged amino acids, changing the protein’s shape.
pH changes directly affect binding sites by altering their shape and chemical properties. Acidic or basic conditions can disrupt the precise configuration needed for substrate recognition.
In basic environments, hydroxide ions can deprotonate positively charged groups, again disrupting the protein’s structure and function.
Outside of the optimal pH range, transport efficiency decreases significantly. This is why maintaining proper pH in cellular compartments is essential for normal function.
Understanding pH effects on transport proteins is critical in both normal physiology and in pathological conditions where pH balance is disrupted.
Scientists use specialized laboratory techniques to study facilitated diffusion across cell membranes.
The first major technique is radioactive tracers. In this method, molecules are labeled with radioactive isotopes such as tritium or carbon-14. This allows scientists to track their movement across membranes and precisely measure transport rates.
The second technique is fluorescent tagging. Transport proteins are labeled with fluorescent molecules like Green Fluorescent Protein. Using fluorescence microscopy, scientists can visualize protein locations and track their movements in real time.
The third key technique is patch-clamping. A glass micropipette forms a tight seal with a small patch of cell membrane. This allows scientists to record electrical currents as ions move through individual channel proteins. This technique provides detailed functional data about how transport channels operate.
Scientists also use advanced imaging techniques. Methods like FRET can measure interactions between proteins. Total Internal Reflection Fluorescence, or TIRF, visualizes molecules near the membrane surface. Cryo-electron microscopy reveals detailed three-dimensional structures of transport proteins.
These experimental methods have profoundly advanced our understanding of facilitated diffusion. They’ve revealed detailed structures of transport proteins, identified key binding sites, and established connections between protein structure and function. This knowledge has also been crucial for developing treatments for diseases related to transport disorders.
Cellular respiration is a vital process that converts glucose into energy, but for this to occur, molecules must first enter the cell and move between different cellular compartments.
Facilitated diffusion plays a crucial role right from the start of cellular respiration. Glucose, the primary energy source, must first enter the cell.
Glucose molecules cannot easily pass through the cell membrane’s phospholipid bilayer. Instead, they rely on specific GLUT transporters embedded in the membrane.
Once glucose enters the cell, it undergoes glycolysis in the cytoplasm to form pyruvate. For cellular respiration to continue, pyruvate must enter the mitochondria.
Pyruvate molecules use specific mitochondrial pyruvate carriers, or MPCs, to cross the outer mitochondrial membrane through facilitated diffusion.
Beyond glucose and pyruvate, cellular respiration involves the transport of many other molecules through facilitated diffusion.
ADP and ATP move in and out of mitochondria via the adenine nucleotide translocator. Fatty acids, another energy source, enter mitochondria through the carnitine shuttle system. And electrons move through protein complexes in the electron transport chain.
These transport systems must be coordinated to maintain efficient cellular respiration. The rate of glucose entry must match metabolic demands.
Glucose enters the cell, pyruvate moves into mitochondria, and ATP is transported out to power cellular activities. This coordination ensures that energy production meets cellular needs.
Transport rates adjust to metabolic demands, and regulatory mechanisms control protein activity. Defects in any of these transport systems can disrupt energy production and lead to metabolic disorders.
Research on facilitated diffusion continues to advance in several exciting directions.
One major research frontier is the determination of transport protein structures. Advanced techniques like cryo-electron microscopy are revealing how these proteins change shape during transport.
Personalized medicine is another exciting frontier. Researchers are studying how genetic variations in transport proteins affect drug response, enabling tailored treatment approaches.
The development of artificial transport systems is a growing field. Scientists are creating synthetic membranes with engineered channels that mimic biological transport proteins.
These research directions will have significant impacts on medicine and biotechnology, from improved drug delivery systems to novel diagnostic technologies and treatment approaches.
As these interconnected research areas advance, we’re gaining deeper insights into facilitated diffusion and developing innovative applications that could transform medicine and biotechnology.
Facilitated diffusion is a critical process that allows specific molecules to cross cell membranes without using cellular energy.
This passive transport process enables molecules to move down their concentration gradient through specialized transport proteins.
Facilitated diffusion relies on two main types of transport proteins: channel proteins and carrier proteins.
Channel proteins form water-filled pores that allow specific ions or molecules to pass through, and can be always open or gated.
Carrier proteins bind specific molecules and undergo conformational changes to transport them across the membrane.
Facilitated diffusion has several key characteristics that distinguish it from other transport mechanisms.
It’s a passive process requiring no energy, follows concentration gradients, demonstrates high specificity for molecules, exhibits saturation kinetics, and is sensitive to environmental factors like temperature and pH.
The biological significance of facilitated diffusion cannot be overstated.
It’s essential for nutrient uptake, ion homeostasis, cell signaling, water balance, and even has implications for drug delivery and therapeutic design.
In conclusion, facilitated diffusion is fundamental to life, enabling cells to maintain their internal environment while exchanging materials with their surroundings.
Facilitated diffusion serves as the essential bridge between cells and their environment, allowing life as we know it to exist.
Study Materials
No study materials available for this video.
Helpful: 0%
Related Videos
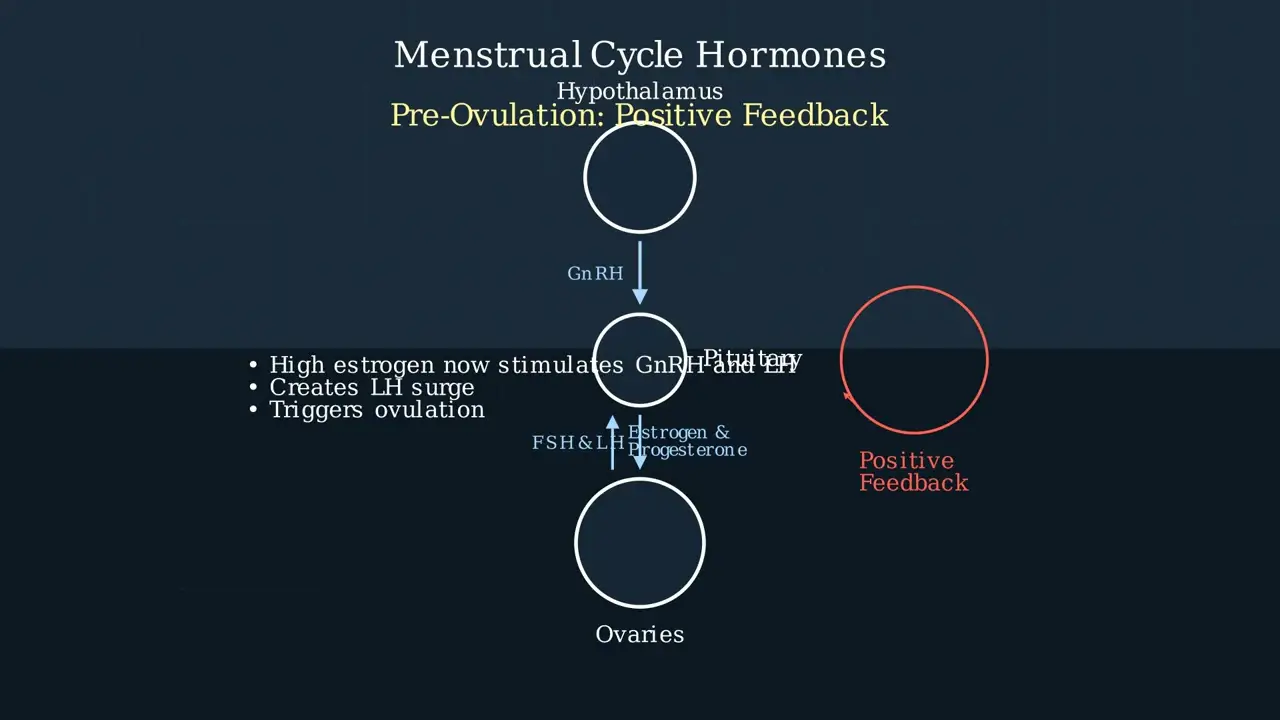
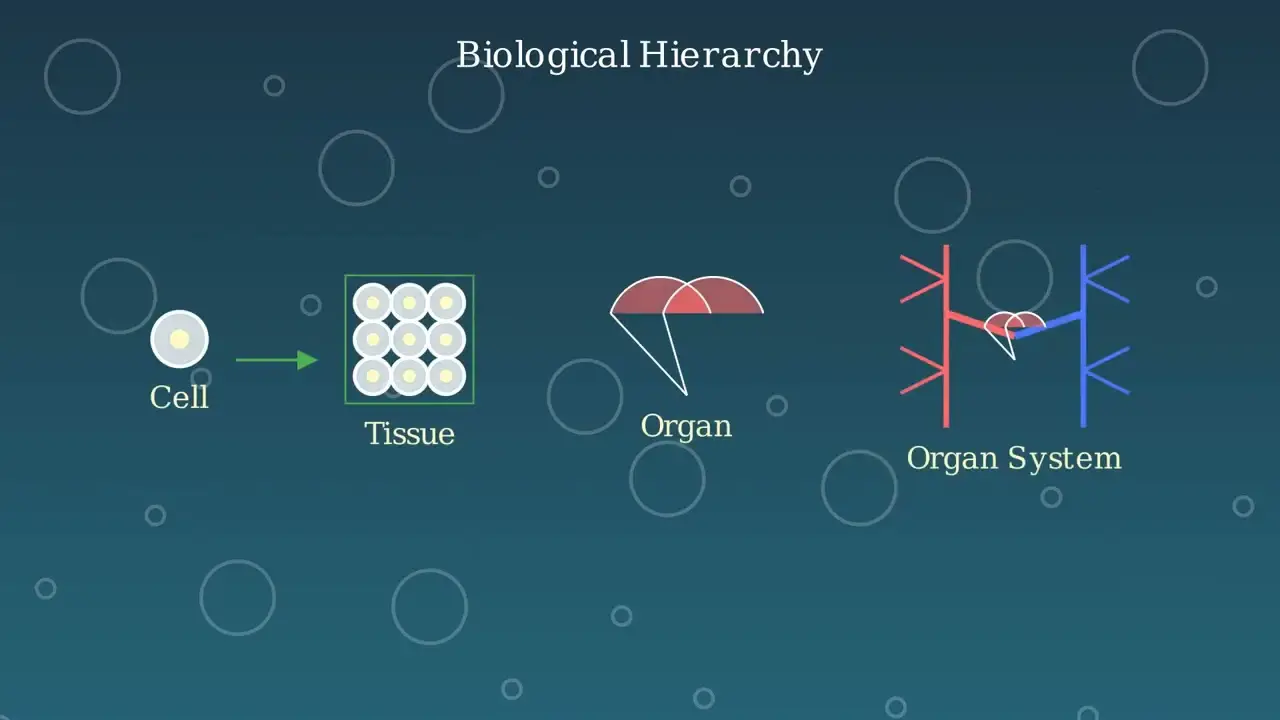
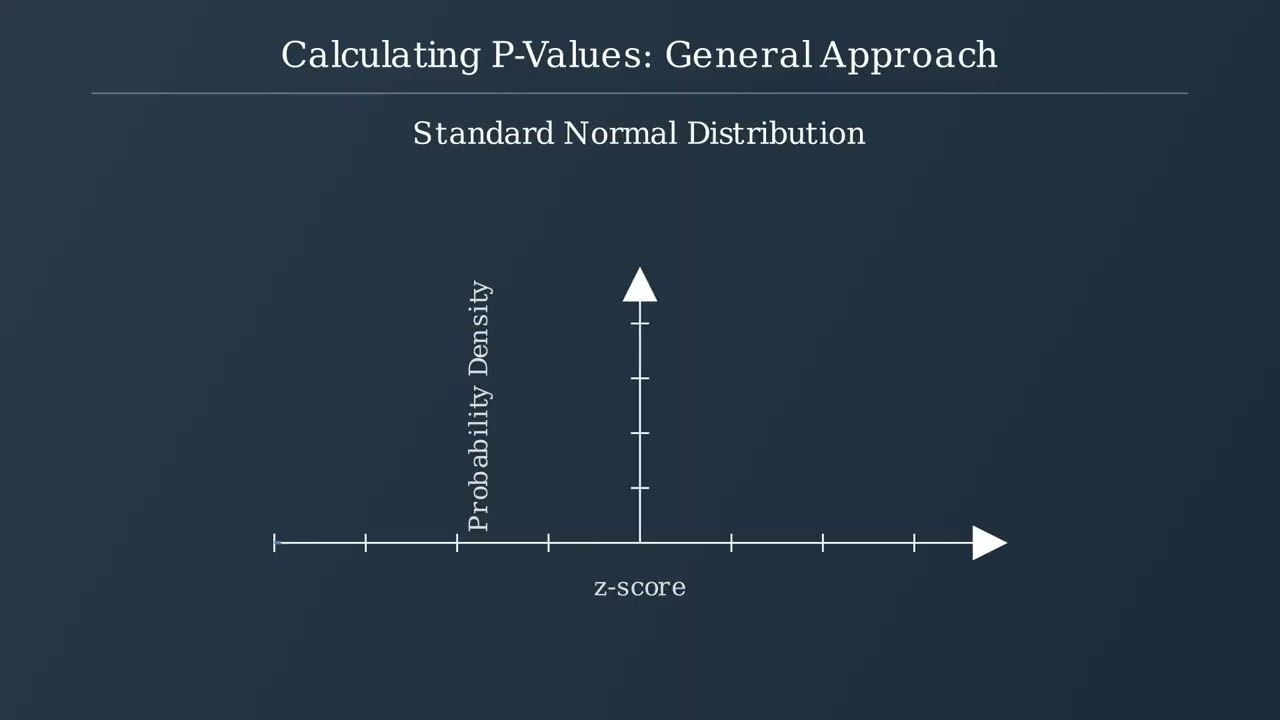
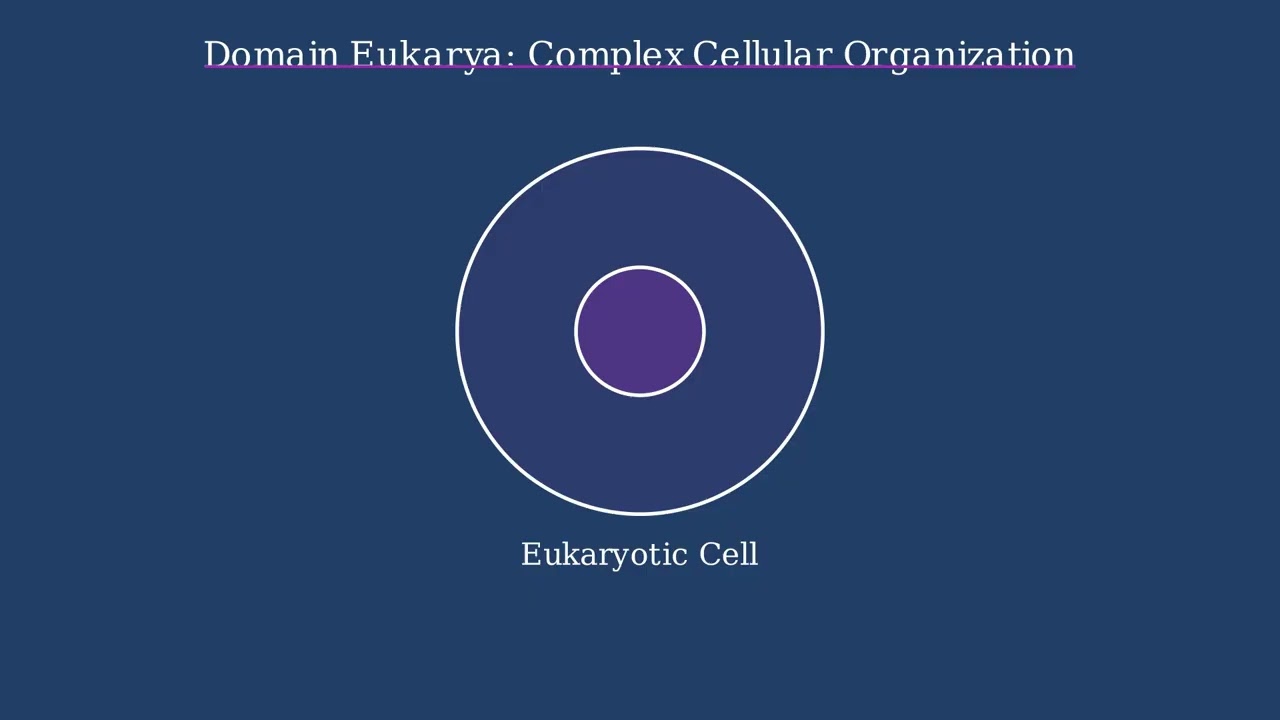
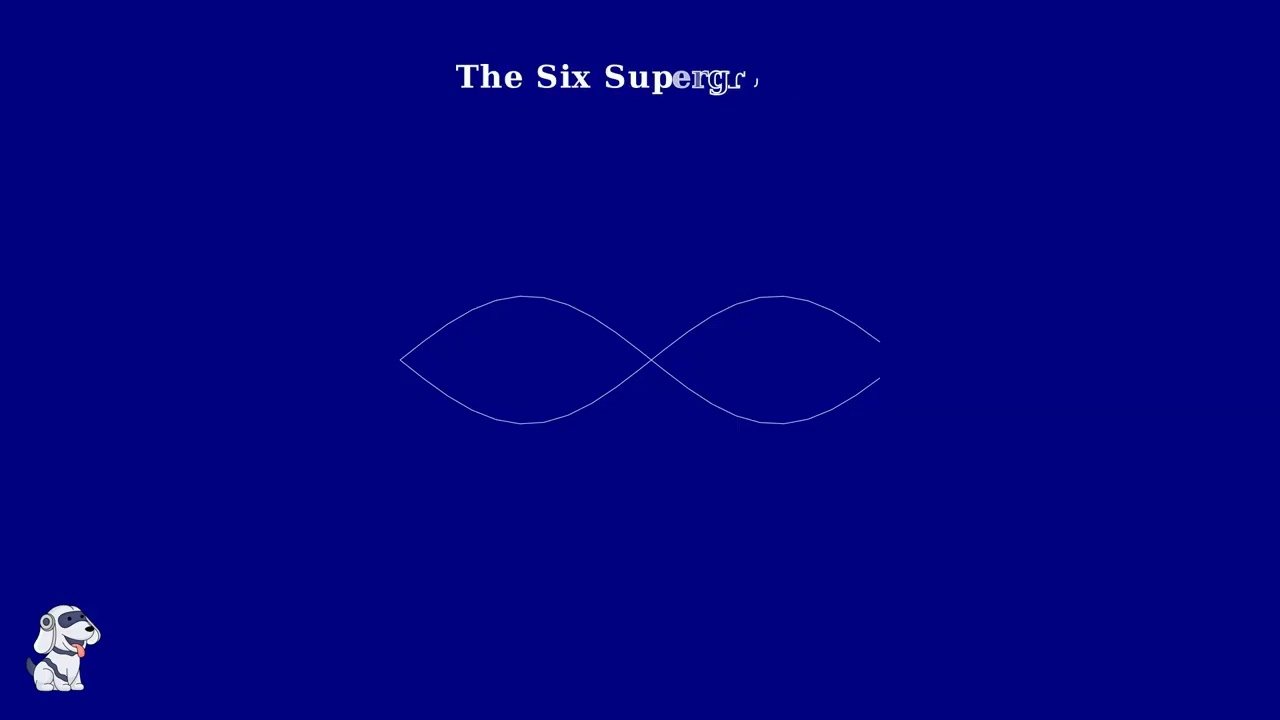
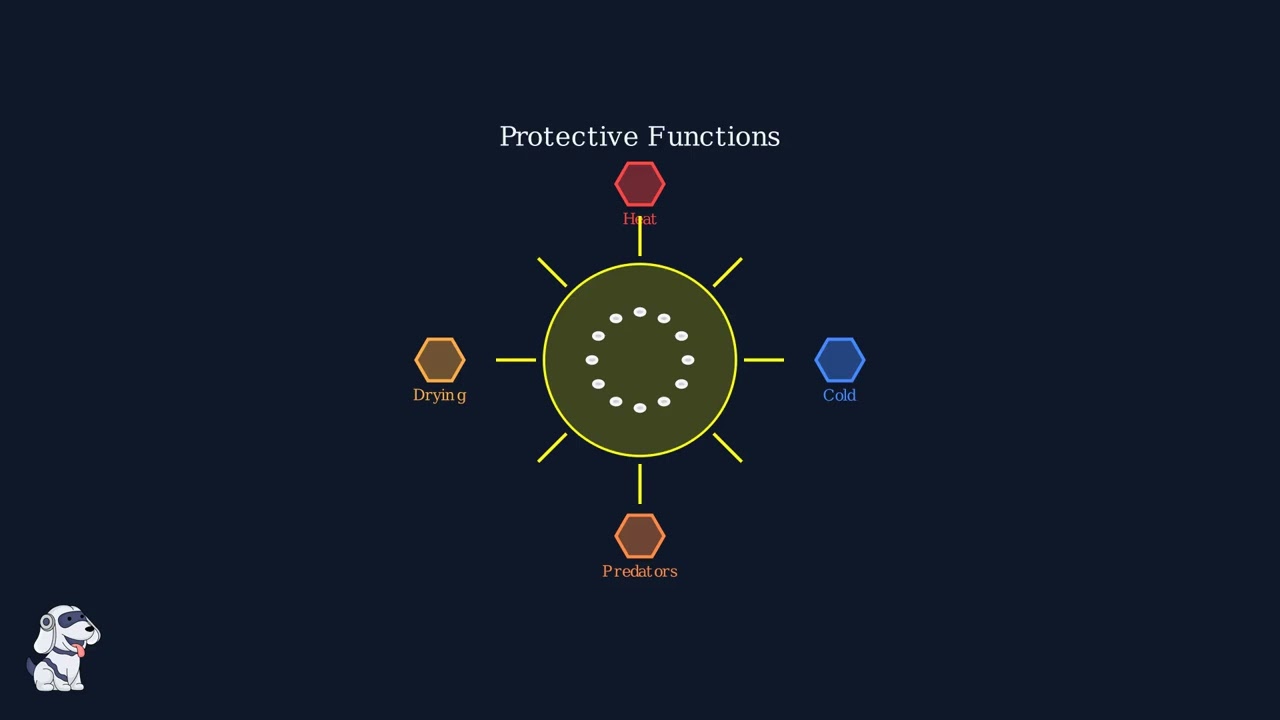
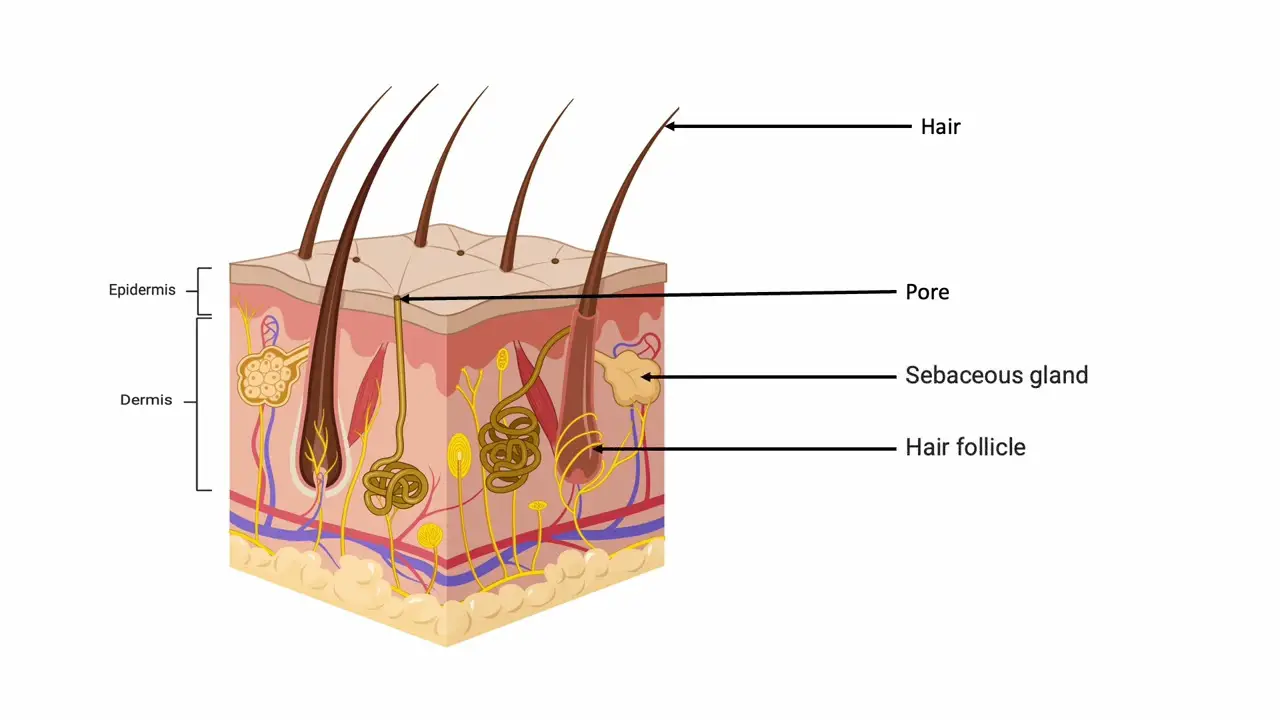
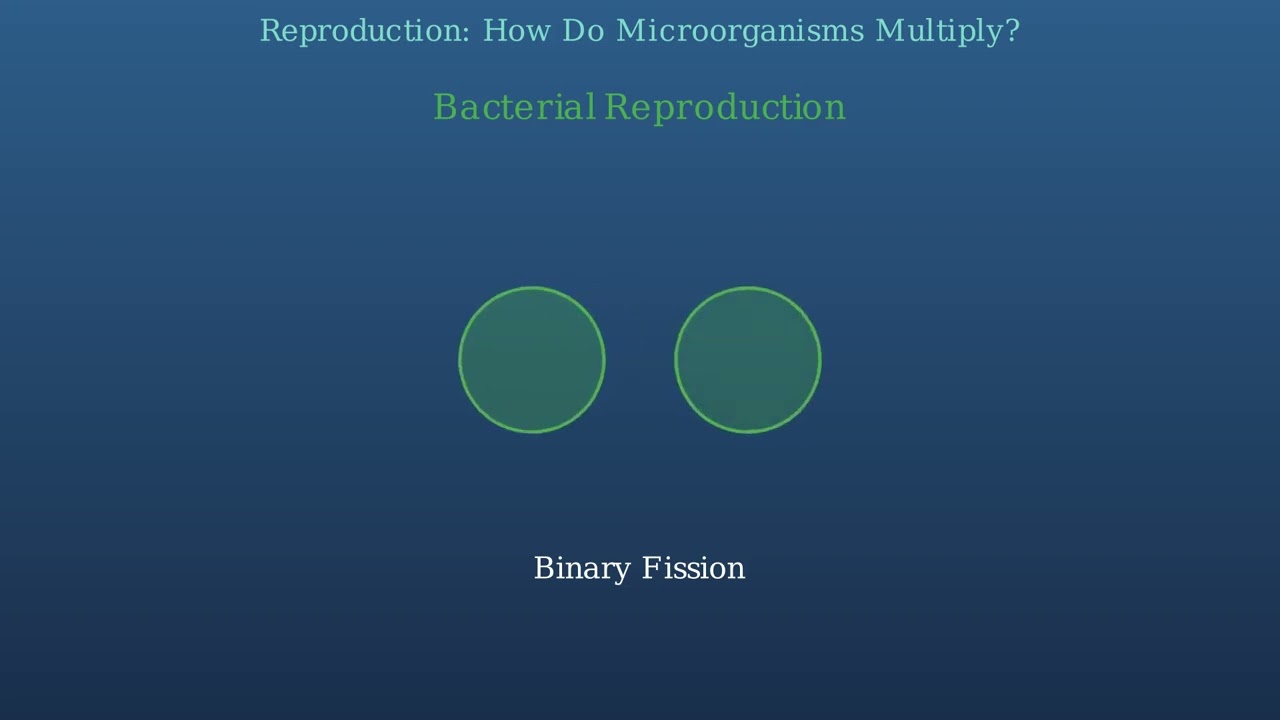
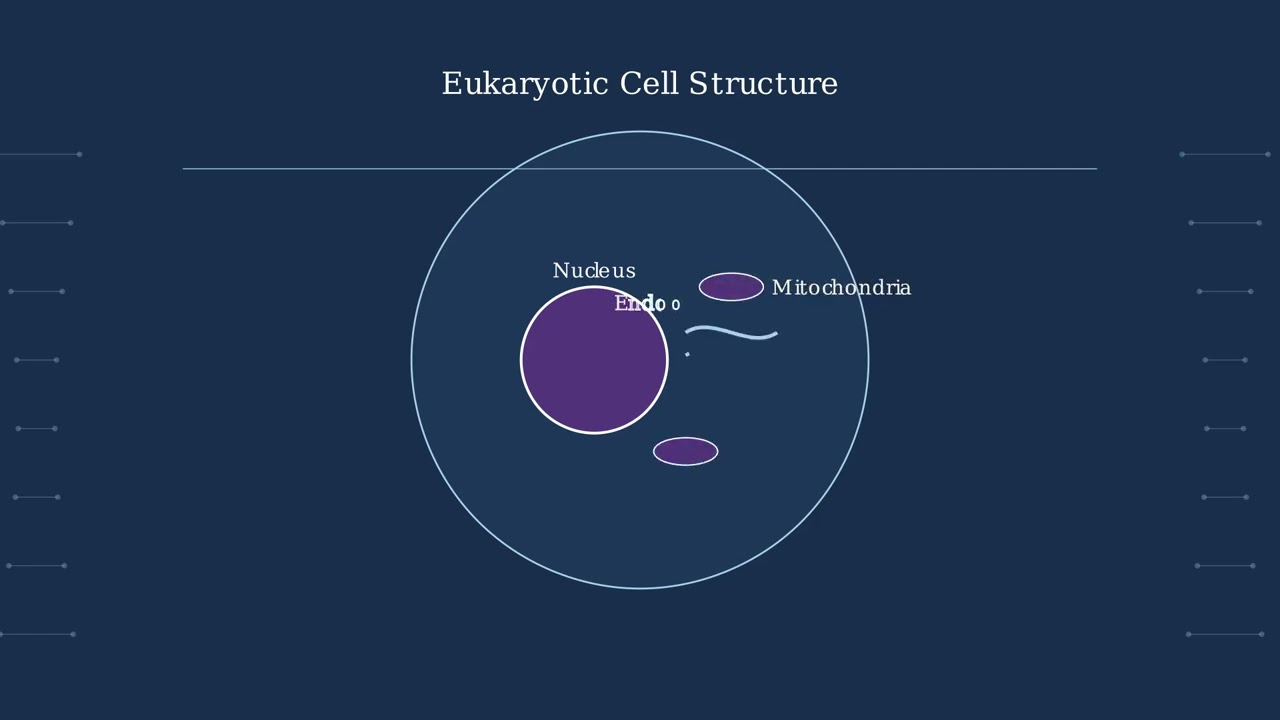
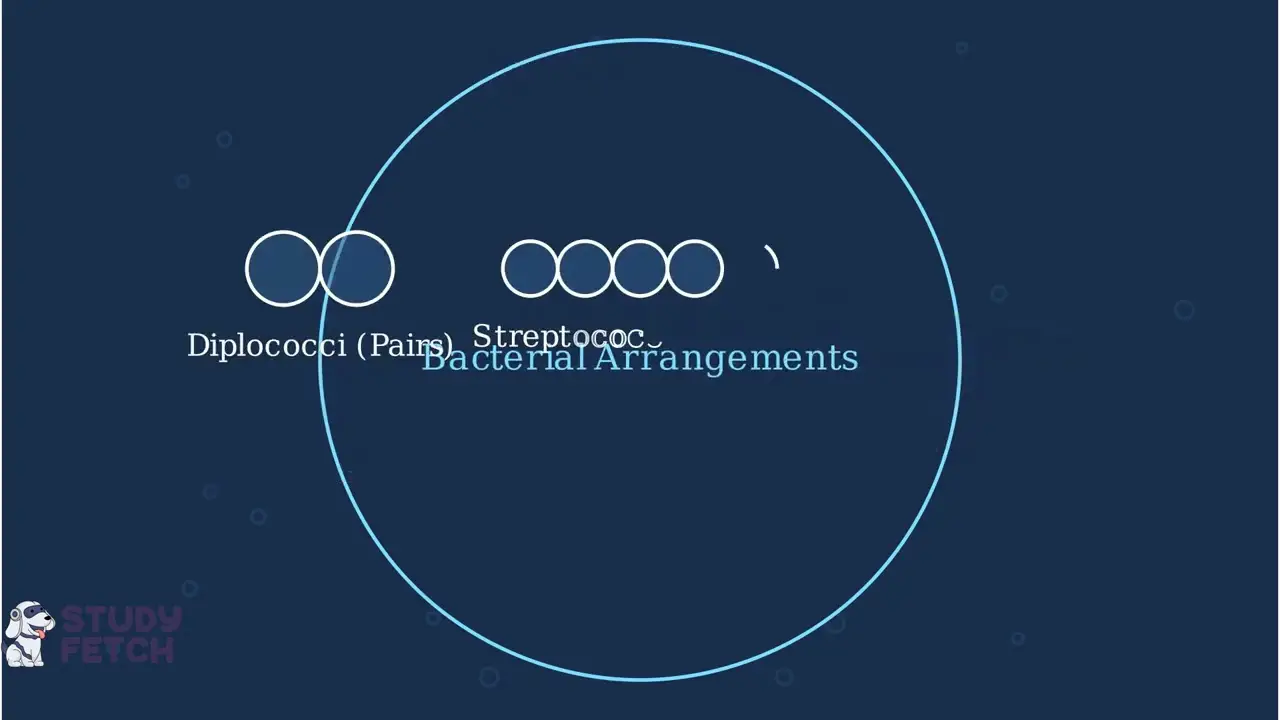