What is Synapse?
- The term “synapse,” first introduced by Charles S. Sherrington in 1897, is derived from the Greek word “synapsis,” which translates to “to conjugate” or “to clasp.” Synapses serve as critical junctions that facilitate communication between neurons, enabling the transmission of nerve signals from one cell to another. This process is essential for all neural activities within the nervous system.
- To confirm the existence and structure of synapses, scientist Sanford Palay conducted studies on neural tissue ultrastructure. His observations highlighted that synapses are indispensable for the functioning of the nervous system; without them, signals cannot effectively reach the brain.
- Synapses can be classified into two main types: electrical and chemical. Electrical synapses allow for the direct transmission of nerve impulses between adjacent neurons, particularly during rapid defense responses. In contrast, chemical synapses involve the release of neurotransmitters triggered by the excitation of a nerve impulse. These neurotransmitters then bind to specific receptors on the postsynaptic neuron, propagating the signal further.
- A synapse consists of several key components. The presynaptic terminal houses synaptic vesicles filled with neurotransmitters, which are crucial for signal transmission. The synaptic cleft, a narrow gap measuring approximately 20 nanometers, separates the presynaptic and postsynaptic neurons. The postsynaptic terminal is equipped with receptor sites that can either inhibit or facilitate the transmission of the nerve signal, depending on the neurotransmitter involved.
- In summary, a synapse represents a junction between two neurons or between a neuron and an effector cell, such as a muscle cell. This junction is pivotal for the conduction of nerve impulses, which occurs from the axon terminal of one neuron to the dendrites of another. The synaptic cleft, the minuscule space between the presynaptic and postsynaptic membranes, is where neurotransmitter diffusion occurs. Following an influx of calcium ions, synaptic vesicles in the presynaptic terminal undergo rapid fusion, releasing neurotransmitters that traverse the synaptic cleft and bind to specialized receptors on the postsynaptic terminal.
Definition of Synapse
A synapse is a junction between two neurons or between a neuron and an effector cell, such as a muscle cell, that allows for the transmission of electrical or chemical signals. It consists of a presynaptic terminal, a synaptic cleft, and a postsynaptic terminal, facilitating communication within the nervous system.
Structure of Synapse
The typical synapse comprises three main elements: the presynaptic neuron, the synaptic cleft, and the postsynaptic neuron.
- Presynaptic Neuron:
- This component is responsible for storing and releasing neurotransmitters.
- It contains several structures:
- Synaptic Vesicles:
- Agranular vesicles, ranging from 35 to 50 nm in diameter, hold small molecule neurotransmitters such as glycine and glutamate.
- Granular vesicles, measuring 80 to 160 nm, typically contain neuropeptides.
- Active Zone:
- A specialized area of the presynaptic terminal where neurotransmitter release occurs.
- Rich in proteins that facilitate the fusion of vesicles with the presynaptic membrane.
- Cytoskeletal Elements:
- Actin filaments assist in the movement of synaptic vesicles toward the presynaptic membrane.
- Microtubules and proteins like fodrin and synapsin I play crucial roles in stabilizing the structure and regulating neurotransmitter release.
- Synaptic Vesicles:
- Synaptic Cleft:
- This is a narrow gap, typically 20 to 30 nanometers wide, filled with interstitial fluid.
- It acts as a medium through which neurotransmitters diffuse from the presynaptic neuron to the postsynaptic neuron.
- Postsynaptic Neuron:
- The postsynaptic membrane is rich in receptor proteins or ligand-gated ion channels.
- Its key functions include:
- Receptor Activation:
- Neurotransmitters released into the synaptic cleft bind to specific receptors, initiating a response in the postsynaptic neuron.
- Ion Flow Regulation:
- The binding of excitatory neurotransmitters (e.g., glutamate) leads to the influx of positive ions, resulting in depolarization (excitatory postsynaptic potential, EPSP).
- Inhibitory neurotransmitters cause the opening of ion channels that allow negatively charged ions to enter, leading to hyperpolarization (inhibitory postsynaptic potential, IPSP).
- Receptor Activation:
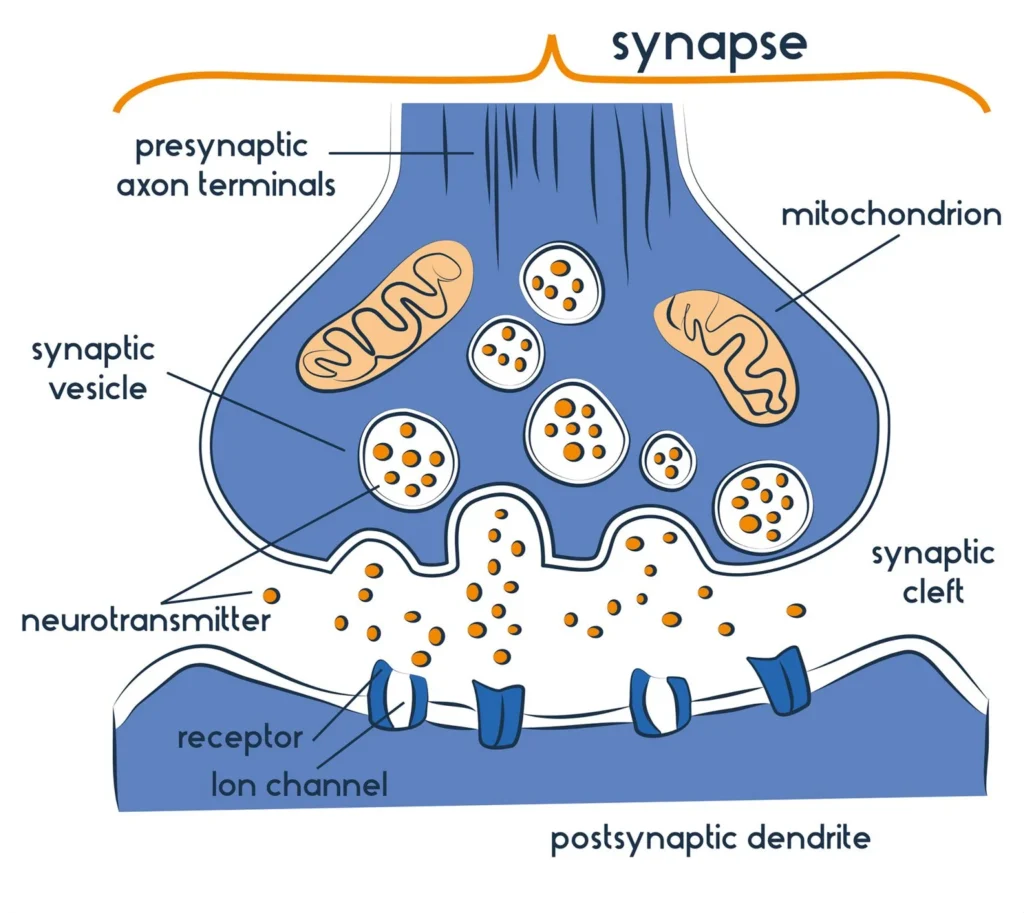
Properties of Synapse
The following are key properties of synapses, presented in a clear and detailed manner.
- One-way Conduction: Synapses facilitate the unidirectional flow of electrical impulses. This means that signals propagate from the presynaptic neuron, which releases neurotransmitters, to the postsynaptic neuron, which receives these chemical signals. This directional transmission is fundamental to the organized functioning of neural circuits.
- Synaptic Delay: A characteristic feature of synaptic transmission is the synaptic delay, which refers to the brief interval between the arrival of an action potential at the presynaptic terminal and the generation of a postsynaptic potential in the postsynaptic neuron. This delay occurs due to the necessary time for neurotransmitter release, diffusion across the synaptic cleft, and receptor binding, typically lasting a few milliseconds.
- Fatigue: During sustained neural or muscular activity, synapses can exhibit signs of fatigue. This phenomenon is particularly evident in synapses that utilize acetylcholine, where prolonged stimulation can lead to a depletion of this neurotransmitter. Fatigue at synapses can impede effective signal transmission, affecting overall muscle performance and coordination.
- Electrical Properties: Synapses are characterized by their electrical properties, notably through the generation of inhibitory postsynaptic potentials (IPSPs) and excitatory postsynaptic potentials (EPSPs). IPSPs decrease the likelihood of the postsynaptic neuron firing, while EPSPs increase this probability. The balance between these potentials is crucial for the regulation of neuronal excitability and network dynamics.
- Summation: Summation is a process by which multiple excitatory inputs can lead to a cumulative effect on the postsynaptic neuron. This can occur through two mechanisms: spatial summation, where several presynaptic neurons fire simultaneously, and temporal summation, where a single presynaptic neuron fires repeatedly in quick succession. The resultant increase in EPSP can lead to the threshold being reached for action potential generation, thereby facilitating effective neural communication.
Types of Synapse
Synapses are critical junctions in the nervous system, facilitating communication between neurons. They can be classified based on their structure, neurotransmitter types, and signal transduction mechanisms. Understanding the various types of synapses is essential for comprehending how neural communication influences physiological processes and behaviors.
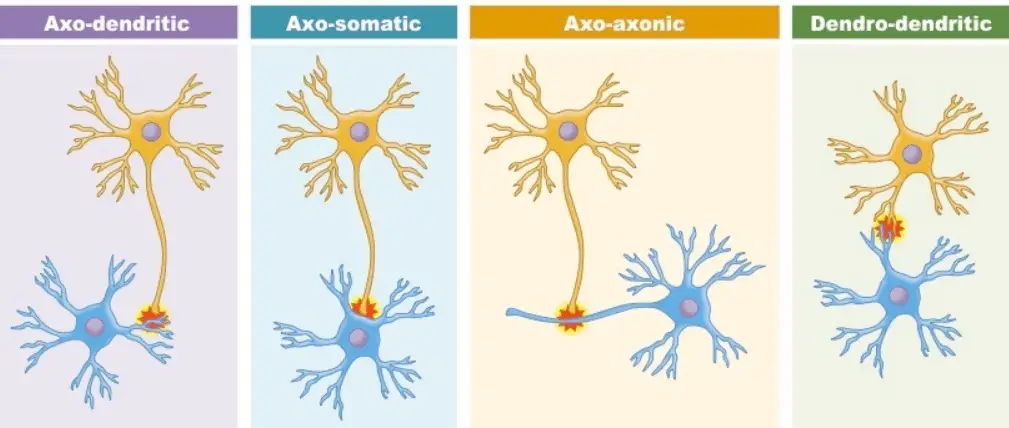
- Types of Structural Synapses:
- Axo-dendritic Synapses: Involve an axon connecting to a dendrite, enabling the transmission of signals to the receiving neuron.
- Axo-somatic Synapses: Occur when an axon synapses directly onto the cell body of another neuron, often influencing the overall excitability of the postsynaptic neuron.
- Axo-axonic Synapses: This configuration features an axon synapsing onto another axon, playing a pivotal role in modulating neurotransmitter release.
- Dendo-dendritic Synapses: These involve dendrites connecting to each other, allowing for complex integration of signals between multiple neurons.
- Classification Based on Neurotransmitter Types:
- Excitatory Ion Channel Synapses:
- Contain receptors that function as sodium ion channels.
- When activated, sodium ions flow into the cytosol, depolarizing the membrane potential and potentially generating an action potential.
- For action potential propagation, a stimulus must surpass a critical threshold.
- The arrival of an action potential at the presynaptic terminal stimulates the release of neurotransmitters (e.g., acetylcholine, glutamate) from synaptic vesicles, facilitating communication with adjacent neurons.
- Inhibitory Ion Channel Synapses:
- Utilize receptors that are chloride ion channels.
- The influx of chloride ions results in hyperpolarization, making it more challenging for the postsynaptic neuron to fire an action potential.
- Common inhibitory neurotransmitters include glycine and gamma-aminobutyric acid (GABA).
- Non-Channel Synapses:
- Feature receptors that act as membrane-bound enzymes rather than traditional ion channels.
- When activated by neurotransmitters, these enzymes release chemical messengers that induce longer-lasting effects, such as those related to learning and memory.
- Examples of involved neurotransmitters include acetylcholine, epinephrine, and dopamine.
- Excitatory Ion Channel Synapses:
- Classification Based on Signal Transduction Methods:
- Electrical Synapses:
- Allow direct transmission of nerve impulses through gap junctions, enabling rapid communication between adjacent cells.
- These synapses do not require neurotransmitters, and the signal can travel bidirectionally.
- The gap between the cells in electrical synapses is approximately 3.5 nm, facilitating synchronized ion movement.
- While electrical signals are typically excitatory, they can diminish in strength as they propagate between neurons, necessitating a larger presynaptic neuron to effectively stimulate a smaller postsynaptic neuron.
- Chemical Synapses:
- Characterized by synaptic knobs containing vesicles filled with neurotransmitters.
- Transmission occurs via the release of these chemical messengers, facilitated by the influx of calcium ions through voltage-gated channels, which triggers vesicle fusion with the presynaptic membrane.
- Chemical synapses can be further categorized into:
- Excitatory Chemical Synapses:
- Promote the conduction of action potentials by allowing sodium ions to enter the cell, leading to depolarization.
- Inhibitory Chemical Synapses:
- Inhibit action potential propagation by opening potassium and chloride channels, resulting in hyperpolarization of the postsynaptic membrane.
- Excitatory Chemical Synapses:
- Electrical Synapses:
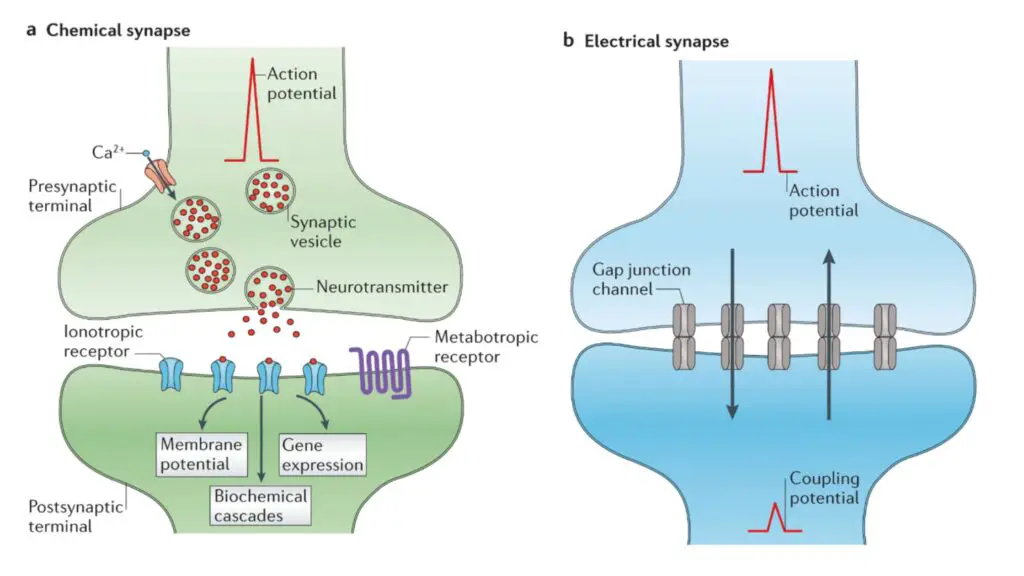
Differences Between between electrical and chemical synapses
These two types of synapses serve distinct roles in neural signaling, and their structural and functional characteristics vary significantly.
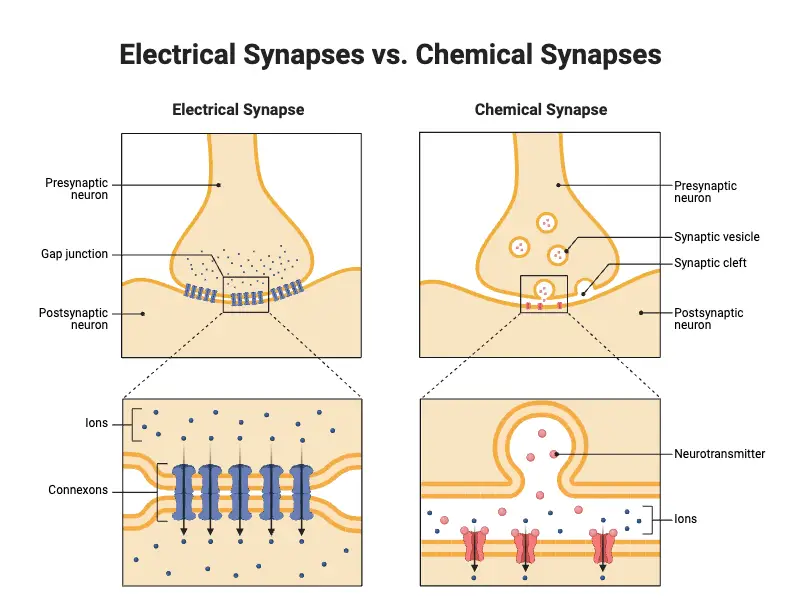
- Chemical Synapses:
- Location: Predominantly found in higher vertebrates.
- Transmission Mechanism: Utilizes neurotransmitters to convey impulses.
- Neurotransmitter Release: Presynaptic neurons release neurotransmitters into the synaptic cleft.
- Binding to Receptors: These neurotransmitters bind to receptors on the postsynaptic neuron, triggering a response.
- Directionality: Transmission is unidirectional, meaning signals travel from the presynaptic to the postsynaptic neuron.
- Gap Width: The synaptic cleft measures approximately 20 nm, allowing for the diffusion of neurotransmitters.
- Transmission Speed: Relatively slow, taking several milliseconds for signals to be transmitted.
- Functional Diversity: Can mediate both inhibitory and excitatory signals, depending on the neurotransmitter and receptor type.
- Signal Strength: Signals may attenuate over distance and time, requiring precise timing for effective communication.
- Sensitivity: Sensitive to changes in pH and hypoxia, which can affect neurotransmitter release and receptor function.
- Fatigue Vulnerability: More susceptible to fatigue during prolonged activity, leading to reduced neurotransmitter availability.
- Electrical Synapses:
- Location: Present in both lower and higher vertebrates, as well as invertebrates.
- Transmission Mechanism: Impulses are transmitted through the direct flow of ions.
- Gap Junctions: Formed by connexons that create channels allowing ions to pass between adjacent cells.
- Directionality: Transmission is bi-directional, permitting signals to flow in both directions between connected neurons.
- Gap Width: The synaptic cleft is much narrower, typically 3 to 5 nm, facilitating rapid ionic flow.
- Transmission Speed: Extremely fast, often occurring almost instantaneously.
- Functional Nature: Primarily excitatory, facilitating synchronized activity among neurons.
- Signal Strength: Signals remain robust but may diminish over distance, particularly in complex networks.
- Sensitivity: Generally insensitive to variations in pH and hypoxia, making them more stable under diverse physiological conditions.
- Fatigue Vulnerability: Less vulnerable to fatigue, allowing for sustained activity without significant signal loss.
Feature | Chemical Synapses | Electrical Synapses |
---|---|---|
Location | Predominantly in higher vertebrates | In both lower and higher vertebrates, invertebrates |
Transmission Mechanism | Uses neurotransmitters | Direct ion flow |
Neurotransmitter Release | Released by presynaptic neurons into synaptic cleft | Formed by connexons allowing ion passage |
Binding to Receptors | Binds to receptors on postsynaptic neurons | Direct ion exchange between cells |
Directionality | Unidirectional (presynaptic to postsynaptic) | Bi-directional |
Gap Width | ~20 nm (facilitates neurotransmitter diffusion) | 3 to 5 nm (facilitates rapid ionic flow) |
Transmission Speed | Relatively slow (milliseconds) | Extremely fast (almost instantaneous) |
Functional Diversity | Can be excitatory or inhibitory | Primarily excitatory |
Signal Strength | May attenuate over distance and time | Robust signals, may diminish over distance |
Sensitivity | Sensitive to pH and hypoxia | Generally insensitive to pH and hypoxia |
Fatigue Vulnerability | More susceptible to fatigue | Less vulnerable to fatigue |
Chemical Synapse and Its Functions
Understanding the processes involved in chemical synapses is crucial for comprehending how nerve impulses are propagated.
- Structure of Chemical Synapses: Chemical synapses are the most prevalent type of synapse in the nervous system. The presynaptic terminal, characterized by its knob-like structure, houses synaptic vesicles that contain neurotransmitters. These vesicles are critical for the transmission of signals across the synaptic cleft.
- Release of Neurotransmitters: Upon the arrival of an action potential at the presynaptic terminal, synaptic vesicles fuse with the membrane and release their neurotransmitter contents into the synaptic cleft. This release is a calcium-dependent process, initiated by the influx of calcium ions through voltage-gated calcium channels.
- Binding to Receptors: The released neurotransmitters diffuse across the synaptic cleft and bind to specific receptors on the postsynaptic membrane. This interaction is crucial, as it determines the postsynaptic neuron’s response to the signal.
- Ion Flow and Membrane Polarization: Binding of neurotransmitters to receptors results in the opening of ion channels, allowing the flow of ions such as sodium (Na⁺) or potassium (K⁺). This ion movement alters the polarization of the postsynaptic membrane. If positively charged ions enter the cell, the membrane depolarizes, leading to excitatory postsynaptic potentials (EPSPs). Conversely, if the movement of ions makes the membrane more negative, the postsynaptic neuron experiences hyperpolarization, resulting in inhibitory postsynaptic potentials (IPSPs).
- Types of Neurotransmitters: Neurotransmitters can exert either excitatory or inhibitory effects, depending on the type of receptors they bind to and the specific cellular context. For instance, while glutamate typically acts as an excitatory neurotransmitter, gamma-aminobutyric acid (GABA) primarily functions as an inhibitory neurotransmitter. Therefore, one neurotransmitter may initiate varying responses in different target cells.
- Signal Termination: The action of neurotransmitters is transient. After binding to receptors, neurotransmitters are either enzymatically degraded or reabsorbed by the presynaptic neuron through reuptake mechanisms. This recycling process is essential for terminating the signal and preparing the synapse for subsequent impulses.
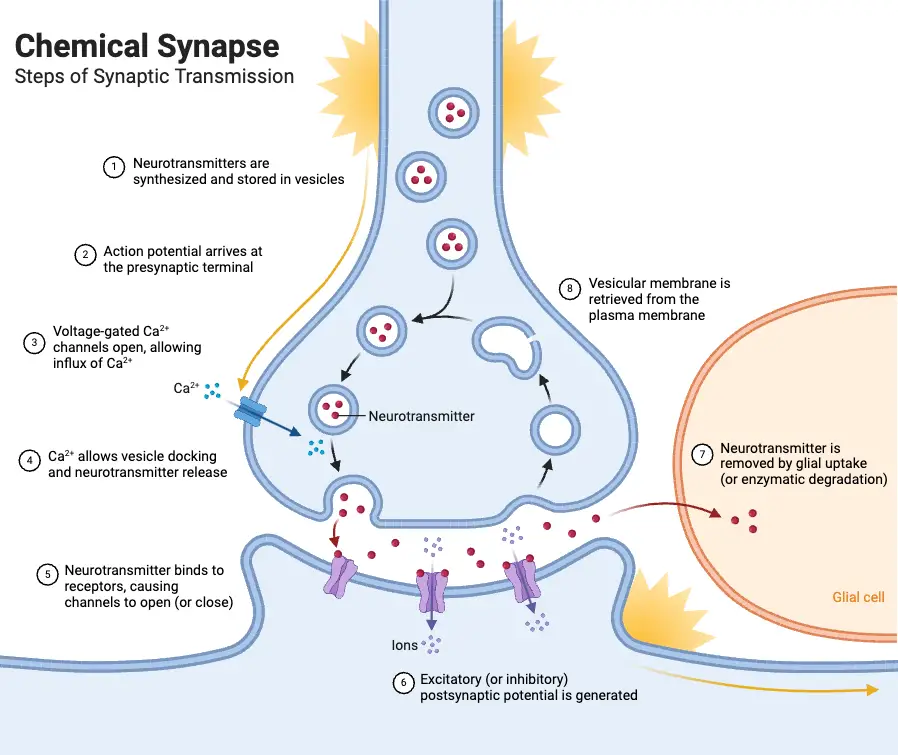
Electrical Synapse and Its Functions
These synapses enable direct electrical communication, facilitating swift responses in neural circuits.
- Rapid Transmission: Electrical synapses are notable for their speed, allowing impulses to travel more quickly than through chemical synapses. This rapid communication is essential in situations requiring immediate responses, such as reflexes.
- Gap Junctions: The defining feature of electrical synapses is the presence of gap junctions. These specialized structures consist of clusters of protein channels known as connexons, which connect the presynaptic and postsynaptic membranes. This close proximity allows for direct ion flow between neurons.
- Ion Flow and Electrical Signals: At electrical synapses, the conduction of an electric signal occurs similarly to how impulses are transmitted along an axon. The direct passage of ions through gap junctions enables the instantaneous transfer of depolarization, allowing for synchronized neuronal firing.
- Lack of Flexibility: Unlike chemical synapses, electrical synapses lack the capacity for modulation. They do not convert excitatory signals to inhibitory ones, limiting their functional versatility. This inherent rigidity is a drawback compared to the dynamic nature of chemical signaling.
- Distribution in Organisms: Electrical synapses are primarily found in certain lower invertebrates, where rapid coordination is crucial for survival. In humans, these synapses are more localized, notably occurring between glial cells, which support and modulate neuronal activity.
- Role in Neural Networks: Electrical synapses play a vital role in coordinating activity within neural networks, particularly in regions where synchrony is paramount. Their ability to facilitate quick, bidirectional communication among neurons contributes to the overall efficiency of neural processing.
Synaptic transmission
Synaptic transmission is a fundamental process that enables communication between neurons and other target cells. It can occur through either electrical synapses or chemical synapses, each with its own mechanisms.
In electrical synapses, direct and rapid communication occurs through specialized ion channels known as gap junctions. These channels create a low-resistance pathway between the pre-synaptic and post-synaptic cells, allowing the flow of ions and electrical currents. This direct electrical coupling allows for the synchronized activity and rapid transmission of signals between neurons. Electrical synapses are particularly important in situations that require fast and synchronized responses, such as in reflex arcs.
In contrast, chemical synapses involve a more complex process. When an action potential reaches the presynaptic neuron, it triggers the release of neurotransmitters from specialized structures called synaptic vesicles. These neurotransmitters are released into the synaptic cleft, a small gap between the pre-synaptic and post-synaptic cells. The neurotransmitters then diffuse across the cleft and bind to specific receptors on the post-synaptic cell’s membrane.
The binding of neurotransmitters to their receptors initiates a series of events in the post-synaptic cell. Depending on the specific neurotransmitter and receptor combination, this can lead to the opening or closing of ion channels, resulting in changes in the electrical potential of the post-synaptic cell. These changes can either depolarize the cell, increasing the likelihood of generating an action potential (excitatory synapse), or hyperpolarize the cell, decreasing the likelihood of an action potential (inhibitory synapse).
The transmission of signals across chemical synapses is a finely regulated process. After neurotransmitter binding, the neurotransmitters are either cleared from the synaptic cleft through reuptake mechanisms or enzymatically broken down. This termination of the signal allows for precise control and modulation of synaptic transmission.
Overall, synaptic transmission plays a crucial role in information processing, integration, and communication within the nervous system. It allows for the transfer of signals from one neuron to another or to target cells, enabling the coordination of various physiological processes, sensory perception, motor control, and cognitive functions.
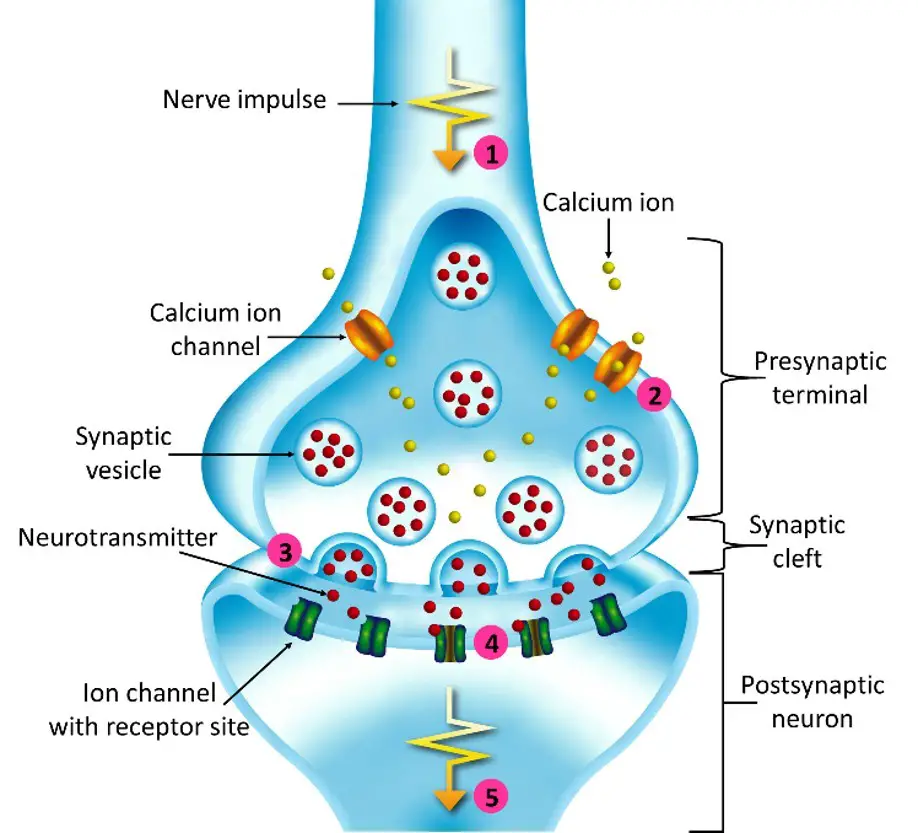
Principles of chemical synaptic transmission
Chemical synaptic transmission is a complex yet finely tuned process essential for communication between neurons. It involves multiple steps, each critical for the effective relay of signals throughout the nervous system. Understanding these principles can illuminate the intricate workings of neural communication.
- Neurotransmitter Synthesis: Chemical synaptic transmission begins with the synthesis of neurotransmitters. Different neurotransmitters, such as amino acids, amines, and peptides, are produced through various biochemical pathways. Amino acids like glutamate and glycine are readily available, while neurotransmitters like GABA and amines require specific enzymes that are synthesized in the neurons that release them.
- Storage in Synaptic Vesicles: Once synthesized, neurotransmitters are packed into synaptic vesicles, small membrane-bound compartments. Transporter proteins embedded in the vesicle membrane facilitate this process, concentrating neurotransmitters for efficient release.
- Action Potential and Neurotransmitter Release: The arrival of an action potential at the axon terminal triggers the opening of voltage-gated calcium channels. This influx of calcium ions ([Ca²⁺]i) is crucial; it prompts synaptic vesicles to undergo exocytosis, a process where vesicle membranes fuse with the presynaptic membrane, releasing neurotransmitters into the synaptic cleft. The rapidity of this process is significant, occurring within milliseconds.
- Postsynaptic Responses: Upon entering the synaptic cleft, neurotransmitters bind to specific receptors on the postsynaptic membrane. These receptors can be classified into two main types: transmitter-gated ion channels and G-protein-coupled receptors. The binding induces conformational changes that lead to various postsynaptic effects. For example, neurotransmitter-gated ion channels typically mediate fast synaptic responses by allowing ions like sodium or chloride to flow through, generating excitatory or inhibitory postsynaptic potentials (EPSPs or IPSPs).
- Neurotransmitter Recovery and Degradation: After neurotransmitter release, it is imperative to clear the synaptic cleft to enable subsequent signaling. This can occur through diffusion, reuptake by presynaptic neurons, or enzymatic degradation. Transporter proteins on the presynaptic membrane facilitate reuptake, allowing neurotransmitters to be repackaged into vesicles or metabolized. Enzymes, such as acetylcholinesterase (AChE), play a vital role in breaking down neurotransmitters like acetylcholine to prevent prolonged activation of postsynaptic receptors.
- Autoreceptors: Presynaptic neurons also contain autoreceptors, which monitor neurotransmitter levels in the synaptic cleft. These receptors, typically G-protein-coupled, provide feedback to inhibit further release when neurotransmitter concentration is sufficiently high, thus functioning as a regulatory mechanism.
- Pharmacological Implications: The intricate nature of chemical synaptic transmission makes it a target for various drugs and toxins, which can modify synaptic function. Understanding these interactions, such as the roles of receptor agonists and antagonists, has profound implications for neuropharmacology, particularly in treating neurological and psychiatric disorders.
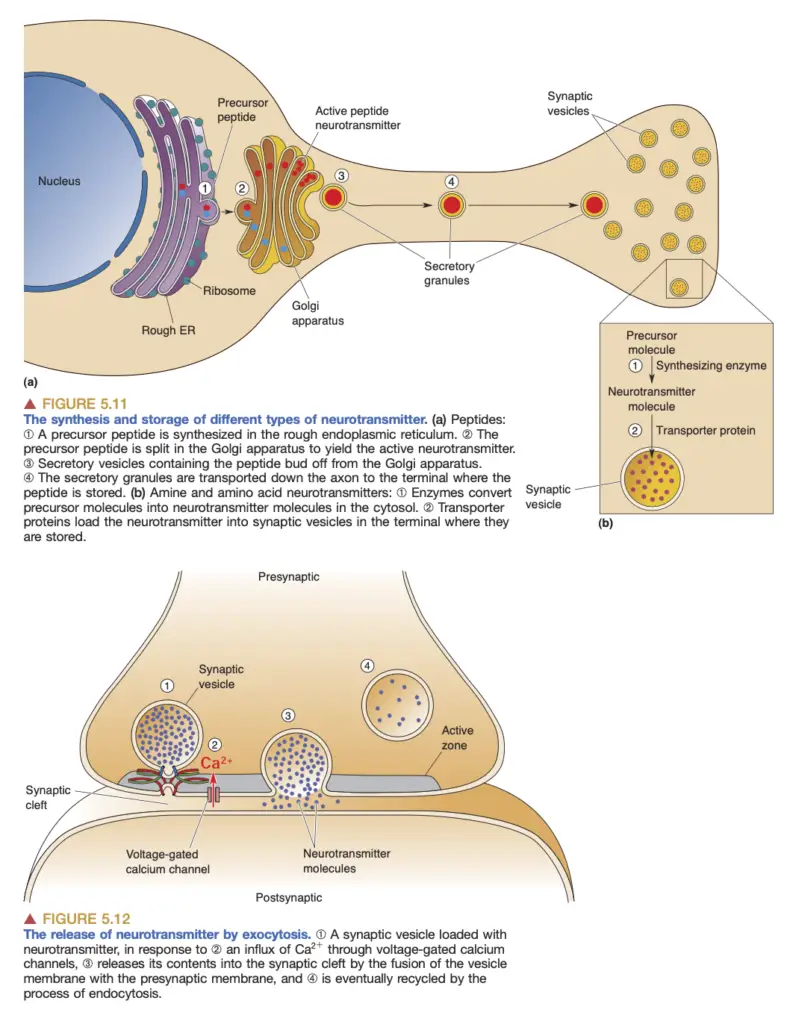
Principles of synaptic integration
Synaptic integration is a fundamental process through which postsynaptic neurons combine multiple synaptic inputs, transforming these diverse signals into a coherent output, primarily action potentials. This transformation allows the nervous system to perform complex computations essential for behavior and cognitive function.
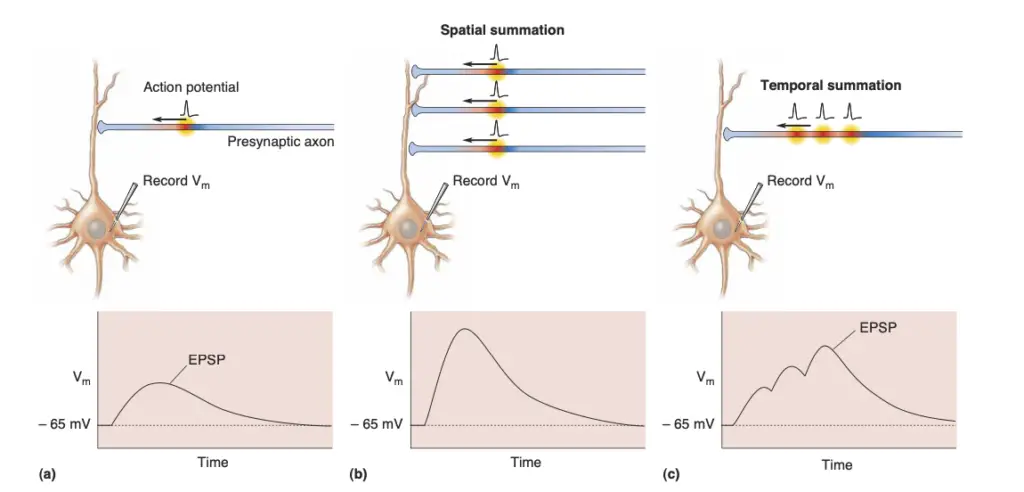
- Synaptic Inputs: Central nervous system (CNS) neurons can receive thousands of synaptic inputs, activating various transmitter-gated ion channels and G-protein-coupled receptors. This intricate interplay of signals results in a unified postsynaptic response.
- Excitatory Postsynaptic Potentials (EPSPs): The simplest response in the postsynaptic neuron arises from the opening of transmitter-gated channels, resulting in depolarization and generating EPSPs. The strength of the EPSP is contingent upon the quantity of neurotransmitter released, which is determined by the activation of synaptic vesicles.
- Quantal Analysis: The quantal nature of neurotransmitter release can be understood through the concept of quantal analysis. Each synaptic vesicle contains a consistent number of neurotransmitter molecules, and the resultant postsynaptic response is a multiple of the response to a single vesicle. This analysis reveals that the amplitude of EPSPs is quantized and reflects the number of vesicles released during synaptic transmission.
- Miniature Postsynaptic Potentials (mPSPs): In the absence of presynaptic stimulation, some vesicles release their contents spontaneously, generating miniature EPSPs, or “minis.” The amplitude of an evoked EPSP correlates with these minis, providing insight into the number of vesicles involved during synaptic transmission.
- EPSP Summation: For a neuron to fire an action potential, it must integrate multiple EPSPs, which occurs through two primary mechanisms: spatial summation and temporal summation. Spatial summation combines EPSPs from different synapses, while temporal summation aggregates EPSPs from the same synapse in quick succession.
- Dendritic Properties: The effectiveness of EPSPs in triggering action potentials also depends on dendritic properties. Dendrites function as passive electrical cables, where the spread of depolarization diminishes with distance due to ionic leakage. The dendritic length constant, which indicates how far depolarization can propagate, is influenced by internal resistance (related to dendritic diameter) and membrane resistance (dependent on ion channel activity).
- Excitable Dendrites: Notably, many dendrites are not purely passive; they contain voltage-gated channels that can amplify EPSPs. These channels allow for the generation of local depolarizations, enhancing signal propagation toward the soma.
- Inhibition: Inhibitory synapses play a crucial role in modulating neuronal output. When activated, these synapses create inhibitory postsynaptic potentials (IPSPs) that move the membrane potential away from action potential threshold. The presence of inhibitory synapses, particularly those using neurotransmitters like GABA or glycine, can significantly dampen the effect of excitatory inputs.
- Shunting Inhibition: This specific form of inhibition occurs when the opening of inhibitory channels allows negatively charged ions (such as chloride) to flow in, effectively reducing the excitatory current flowing toward the soma. This prevents the postsynaptic neuron from reaching the threshold for action potential generation.
- Modulation: Besides direct excitatory and inhibitory signals, synaptic integration can be influenced by modulatory synapses. These synapses utilize G-protein-coupled receptors that do not directly produce EPSPs or IPSPs but instead alter the efficacy of other synaptic inputs. For instance, the binding of norepinephrine to its receptors can initiate a cascade that enhances the postsynaptic response to subsequent excitatory inputs.
- Biochemical Cascades: Modulation often involves second messengers, such as cyclic AMP (cAMP), which can influence various cellular processes, including the phosphorylation of ion channels. Such changes can enhance the resistance of dendrites, increasing their ability to integrate signals over longer distances.

What are EPSPs and IPSPs?
EPSPs (excitatory postsynaptic potentials) and IPSPs (inhibitory postsynaptic potentials) are critical components of synaptic transmission, playing significant roles in neural communication. They reflect how neurons integrate signals and determine whether an action potential will be generated.
- EPSPs:
- Definition: EPSPs result from the influx of positive ions through neurotransmitter-gated ion channels when an excitatory neurotransmitter binds to its receptor on the postsynaptic membrane.
- Mechanism: The process begins with the release of neurotransmitters from presynaptic neurons, leading to the opening of ion channels that allow sodium ions (Na⁺) to enter the postsynaptic neuron, causing depolarization.
- This depolarization can be quantified and is directly proportional to the number of synaptic vesicles released, each containing a specific number of neurotransmitter molecules.
- The amplitude of an EPSP is thus a multiple of the response generated by the contents of a single synaptic vesicle, often referred to as a “quantum.”
- Summation: The effectiveness of EPSPs is influenced by their summation.
- Spatial Summation: Occurs when multiple synapses activate simultaneously, combining their effects on the postsynaptic membrane.
- Temporal Summation: Takes place when successive EPSPs occur at a single synapse in quick succession, enhancing the likelihood of reaching the action potential threshold.
- IPSPs:
- Definition: IPSPs are caused by the activation of inhibitory synapses, resulting in hyperpolarization of the postsynaptic membrane.
- Mechanism: This hyperpolarization occurs primarily through the influx of negatively charged chloride ions (Cl⁻) or the efflux of positive potassium ions (K⁺), effectively making the inside of the neuron more negative.
- Like EPSPs, the receptors for inhibitory neurotransmitters (such as GABA or glycine) are also transmitter-gated ion channels, but they allow for different ionic flows.
- Shunting Inhibition: This phenomenon occurs when the inhibitory synapse is activated close to an excitatory synapse. It diverts positive current away from the axon hillock, thus diminishing the likelihood of action potential generation.
- Shunting inhibition acts as an electrical short circuit, making it harder for the excitatory current to reach the threshold necessary for firing.
- Integration of EPSPs and IPSPs:
- The interplay between EPSPs and IPSPs is essential for neuronal computation.
- The presence of multiple excitatory and inhibitory inputs allows neurons to refine their responses based on the collective signals received.
- A neuron must integrate these postsynaptic potentials to determine whether to fire an action potential, which requires careful consideration of both the number and timing of incoming signals.
- Dendritic properties, such as membrane resistance and length constants, influence the extent to which these potentials can affect the spike-initiation zone.
- The effectiveness of a given EPSP or IPSP diminishes with distance from the synapse due to the passive electrical properties of dendrites.
- The interplay between EPSPs and IPSPs is essential for neuronal computation.
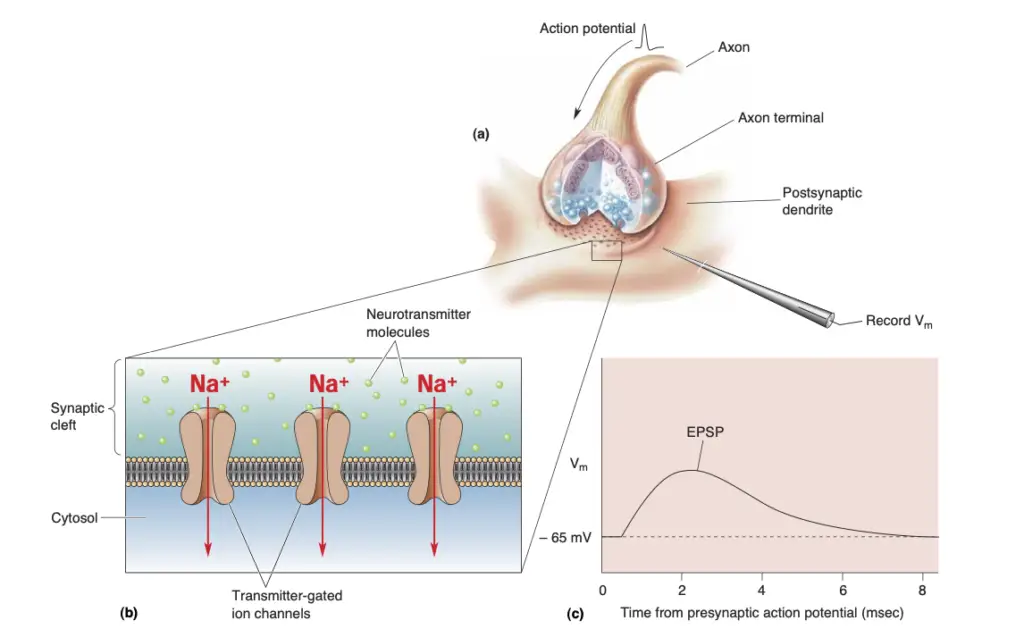
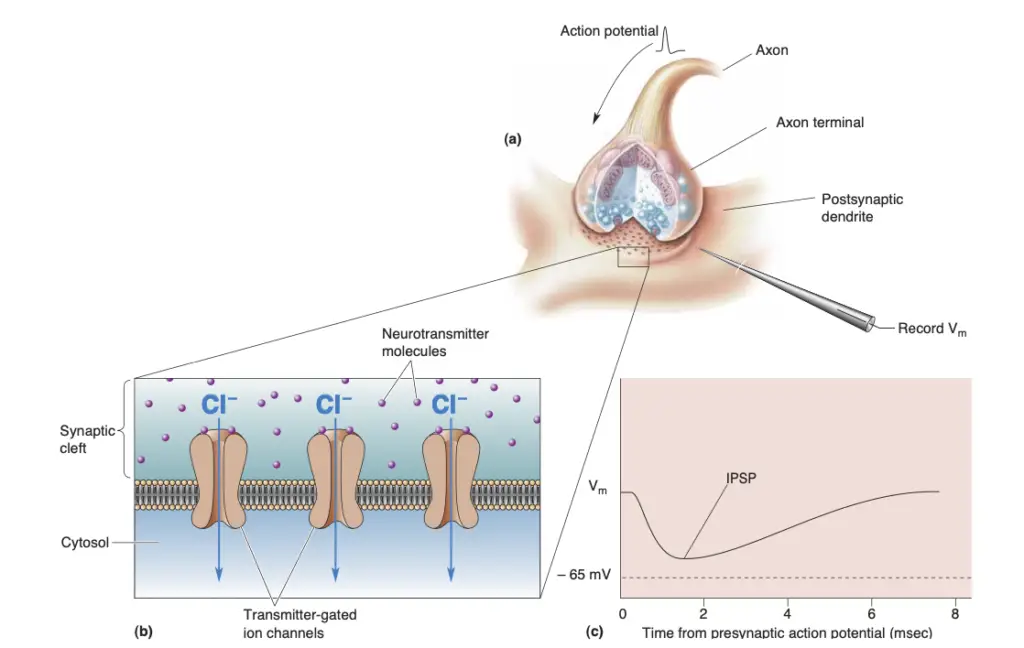
FAQ
What is a synapse?
A synapse is a specialized junction between two neurons, where information is transmitted from one neuron to another.
What is synaptic transmission?
Synaptic transmission is the process by which information is transferred from the presynaptic neuron (sending neuron) to the postsynaptic neuron (receiving neuron) across the synapse.
What are neurotransmitters?
Neurotransmitters are chemical messengers that transmit signals between neurons. They are released from the presynaptic neuron and bind to receptors on the postsynaptic neuron.
How does information transfer occur at the synapse?
Information transfer at the synapse occurs through the release of neurotransmitters from the presynaptic neuron, which bind to receptors on the postsynaptic neuron, leading to changes in its electrical activity.
What are excitatory and inhibitory synapses?
Excitatory synapses increase the likelihood of the postsynaptic neuron firing an action potential, while inhibitory synapses decrease the likelihood of firing an action potential.
What is the role of vesicles in synaptic transmission?
Vesicles are small sacs within the presynaptic neuron that store neurotransmitters. They release neurotransmitters into the synaptic cleft upon the arrival of an action potential.
How are neurotransmitters cleared from the synapse?
Neurotransmitters are cleared from the synapse through various mechanisms, including reuptake by presynaptic transporters, enzymatic degradation, and diffusion away from the synaptic cleft.
What is long-term potentiation (LTP)?
Long-term potentiation is a process that strengthens the connection between two neurons, leading to enhanced synaptic transmission and improved neural communication. It is believed to underlie learning and memory formation.
What are the types of synapses?
There are two main types of synapses: chemical synapses, where neurotransmitters are used to transmit signals, and electrical synapses, where electrical currents directly pass between neurons through gap junctions.
How do drugs and medications affect synaptic transmission?
Drugs and medications can affect synaptic transmission by altering the release, uptake, or receptor binding of neurotransmitters. For example, antidepressants can increase the availability of certain neurotransmitters in the synapse, helping to alleviate symptoms of depression.
- https://www.brainkart.com/article/Classification-of-Synapses_18876/
- https://web.williams.edu/imput/introduction_main.html
- https://www.oist.jp/image/diagram-synaptic-transmission
- https://www.simplypsychology.org/synapse.htmlhttps://www.brainkart.com/article/The-Synapse—Human-Neuroanatomy_18874/
- https://biologyreader.com/synapse.html
- https://www.brainfacts.org/brain-anatomy-and-function/cells-and-circuits/2022/synapses-and-neurotransmission-113022
- https://www.biotopics.co.uk/A17/Synaptic_transmission.html
- https://teachmephysiology.com/nervous-system/synapses/synaptic-transmission/
- https://en.wikipedia.org/wiki/Synapse
- https://www.khanacademy.org/science/biology/human-biology/neuron-nervous-system/a/overview-of-neuron-structure-and-function
- https://eduinput.com/what-are-types-of-synapse/
- https://www.geeksforgeeks.org/synaptic-cleft-anatomy-structure-disease-function/
- https://www.studysmarter.co.uk/explanations/biology/responding-to-change/types-of-synapse/
- https://www.ncbi.nlm.nih.gov/books/NBK11001/
- https://www.medicinenet.com/what_is_synaptic_function/article.htm
- https://openbooks.lib.msu.edu/neuroscience/chapter/synapse-structure/
- https://www.ncbi.nlm.nih.gov/books/NBK526047/
- https://www.sciencefacts.net/synapse.html
- https://www.learnwithabe.com/science-resources/neurons-and-synapses