Life supporting characteristics and biological importance of water to plants
The life-supporting characteristics of water stem from its unique physical and chemical properties, which play an essential role in biological systems, particularly in plants. The significance of water in plants can be attributed to its role in chemical reactions, germination, nutrient transport, temperature regulation, and maintaining cell structure. Below is a detailed outline of the vital functions of water in plants.
- Water as a Chemical Reactant:
- Water is essential for many biochemical reactions in plants, serving as both a reactant and a product.
- During photosynthesis, water undergoes photolysis in the light reaction, breaking into oxygen and hydrogen. The hydrogen is utilized in carbon fixation to produce glucose, forming the foundation of the autotrophic food chain.
- Many other vital biochemical reactions, such as condensation, hydrolysis, oxidation, and reduction, take place in an aqueous medium, highlighting the importance of water for plant life.
- Water for Seed Germination:
- Water is necessary for seeds to germinate, as it hydrates the protoplasm within seeds, initiating essential metabolic reactions.
- Hydration enables metabolites to move within an aqueous medium, triggering growth and development.
- Water as a Solvent:
- Water is highly polar and capable of extensive hydrogen bonding, making it an excellent solvent for numerous molecules, including sugars, amino acids, and carrier proteins such as hemoglobin and myoglobin.
- Polar molecules containing –OH or –NH2 groups dissolve easily in water, facilitating the uptake of mineral nutrients that are critical for plant growth.
- The property of water as a universal solvent also contributed to the chemical reactions in the “hot primeval soup” of Earth’s oceans, leading to the origin of life.
- Thermal Properties of Water:
- Water exhibits unique thermal properties, remaining liquid over a wide range of temperatures conducive to life.
- Its melting and boiling points are higher than expected due to extensive hydrogen bonding between water molecules, contributing to water’s high specific heat, thermal conductivity, latent heat of vaporization, and heat of fusion.
- These thermal characteristics enable water to buffer temperature changes in plant systems, ensuring that life-sustaining conditions are maintained.
- Adhesion and Cohesion:
- Water molecules exhibit cohesion (the attraction between water molecules) and adhesion (the attraction of water to other substances), both due to hydrogen bonding.
- Cohesion allows water to form droplets, while adhesion helps water adhere to the cell walls of the xylem in plants.
- The combination of adhesion and cohesion contributes to water’s tensile strength, allowing a continuous water column to move through plant structures without breaking.
- This tensile strength, combined with surface tension, gives water the property of capillarity, enabling it to rise through narrow spaces in plants, facilitating water transport.
- Providing Turgidity to the Cells:
- The uptake of water by plant cells leads to turgor pressure, inflating the semi-elastic cellulosic walls of the cells and enabling volume expansion.
- Turgidity is critical for maintaining the structure and rigidity of plant cells, with proteins, carbohydrates, and other metabolites being deposited later during growth.
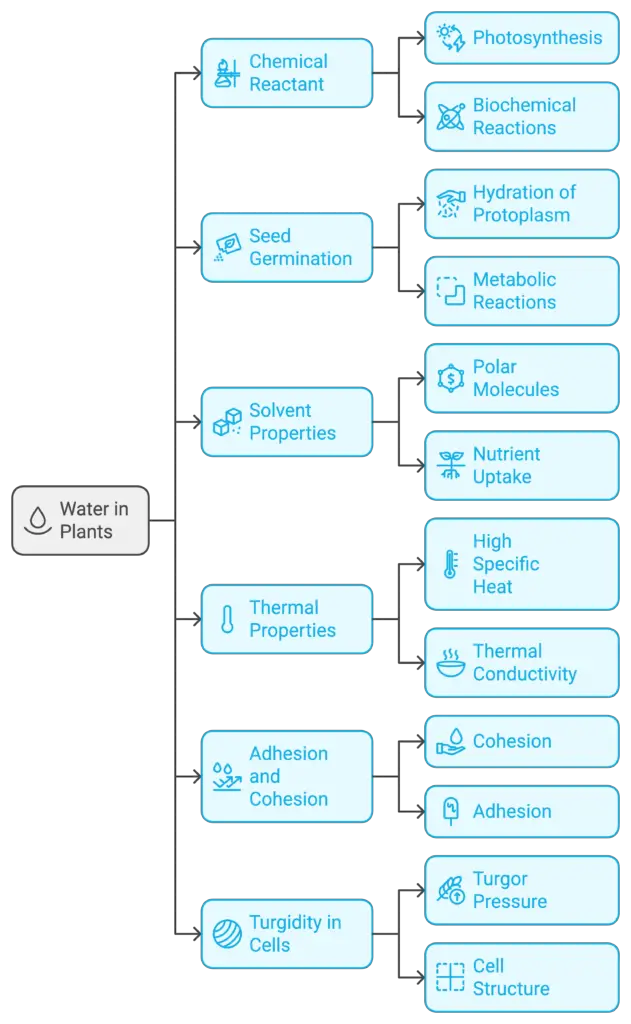
Water transport
Water transport in plants is a complex process that involves various mechanisms allowing water to flow from the soil, through the plant, and eventually into the atmosphere. This movement is essential for maintaining the plant’s hydration, nutrient distribution, and metabolic processes. To understand how water moves within plants, it is important to explore the principles of diffusion, imbibition, mass flow, and osmosis, as well as the different pathways through which water travels.
- Water Flow and Cellular Dynamics:
- Water moves in and out of plant cells through processes such as diffusion, osmosis, imbibition, and mass flow. These processes are driven by the differences in water potential between different areas of the plant.
- Diffusion refers to the passive movement of water molecules from an area of higher concentration to an area of lower concentration.
- Osmosis is the specific movement of water through a semipermeable membrane, driven by concentration gradients.
- Imbibition occurs when water is absorbed by hydrophilic substances, causing swelling.
- Mass flow involves the bulk movement of water in response to pressure differences, moving large amounts of water across regions of the plant.
- Together, these forces facilitate the translocation of water, either passively or actively, depending on the energy requirements of the plant at different stages of water transport.
- Uptake of Water from Soil:
- Water is absorbed by plant roots from the soil through root hairs and epidermal cells. The water enters the plant’s root system, where it is directed to the vascular tissues for transport to other parts of the plant.
- Root cells create a water potential gradient, driving water uptake through osmosis from the surrounding soil into the roots.
- Water Movement Pathways:
- Water moves through the plant via two primary pathways: the symplastic and apoplastic routes.
- In the symplastic pathway, water moves from cell to cell through plasmodesmata, which are tiny channels connecting the cytoplasm of adjacent plant cells. This pathway forms a continuous network of living cells within the plant, known as the symplasm.
- The apoplastic pathway is an alternative route in which water travels through the spaces between cell walls and the non-living cells of the xylem. This pathway moves water across the non-living portions of the plant, collectively referred to as the apoplasm.
- Water Movement to the Atmosphere:
- Once water reaches the leaves, it is lost to the atmosphere through the process of transpiration. This movement is driven by the evaporation of water from the leaf surfaces, primarily through stomata, small openings on the underside of leaves.
- Transpiration plays a crucial role in creating a negative pressure within the xylem, which pulls water upward from the roots to the leaves in a continuous flow.
1. Diffusion
Diffusion is a fundamental process essential to the movement of substances within plants, and it governs various biological activities across all forms of life. At its core, diffusion is the spontaneous movement of particles—whether ions, molecules, or atoms—driven by their inherent kinetic energy. These particles move randomly and tend to spread from areas of higher concentration (or free energy) to regions of lower concentration, resulting in an even distribution across the available space.
- Mechanism of Diffusion:
- Diffusion occurs because particles in any medium, whether gas, liquid, or solid, possess kinetic energy that causes them to collide with one another, dispersing in different directions.
- This dispersal follows a pattern in which particles move from an area of higher concentration (where particles are more densely packed) to an area of lower concentration (where particles are more spread out), continuing until equilibrium is achieved.
- Once equilibrium is reached, the movement of particles in all directions becomes equal, leading to a state where no net movement occurs.
- Fick’s First Law of Diffusion:
- The process of diffusion is quantitatively described by Fick’s First Law, which states that the rate of diffusion is directly proportional to the cross-sectional area of the diffusion path and the concentration or vapor pressure gradient.
- Conversely, the rate of diffusion is inversely proportional to the length of the diffusion path. Therefore, diffusion is most efficient over shorter distances, as the time required for diffusion increases with the square of the distance.
- For example, a glucose molecule can diffuse across a cell with a 50 µm diameter in just 2.5 seconds. However, the same molecule would take nearly 32 years to diffuse a distance of 1 meter in water, illustrating that diffusion is not effective for long-distance transport in plants.
- Factors Influencing the Rate of Diffusion:
- Temperature: The rate of diffusion increases with temperature, as higher temperatures enhance the kinetic energy of diffusate particles, causing them to move more rapidly. The temperature coefficient (Q₁₀) for diffusion is typically between 1.2 and 1.3, indicating the rate of change due to a 10°C increase in temperature.
- Density of Diffusate: Diffusion is inversely proportional to the square root of the diffusate’s density. Gases, being less dense, diffuse more readily than liquids, and solids diffuse the slowest.
- Diffusion Pressure: Diffusate particles exert pressure based on their tendency to diffuse. This pressure drives particles from regions of higher diffusion pressure to areas of lower diffusion pressure.
- Density and Concentration of Diffusant: The rate of diffusion is slower in denser and more concentrated diffusants, as these factors create greater resistance to the movement of particles.
- Phases of Diffusion:
- Diffusion can take place across various phases, including gas-gas, gas-liquid, liquid-liquid, solid-liquid, solid-gas, and even solid-solid interfaces. In each case, the principles of kinetic energy and concentration gradients govern the movement of particles.
- Importance of Diffusion in Plants:
- Diffusion plays a vital role in numerous plant processes, making it essential for the movement and exchange of substances. It contributes to:
- The uptake of water and minerals from the soil into plant roots.
- Osmosis, where water moves through semipermeable membranes.
- Transpiration, allowing water vapor to escape from the leaves.
- Gas exchange during photosynthesis and respiration, where carbon dioxide and oxygen diffuse in and out of plant tissues.
- Short-distance translocation of substances within the symplast (the interconnected cytoplasm of plant cells).
- The spread of ions and molecules throughout the protoplast.
- Wetting of cell walls, facilitating cellular processes.
- Release of odors for pollination, aiding in plant reproduction.
- Diffusion plays a vital role in numerous plant processes, making it essential for the movement and exchange of substances. It contributes to:
2. Imbibition
Imbibition is a key process in plant biology, where water or another liquid is absorbed by solid particles of an insoluble material, referred to as the imbibant, without forming a solution. This mechanism is critical for several biological activities, particularly in seed germination and water movement within plant structures.
- Basic Mechanism:
- The liquid that is absorbed is called the imbibate, and the solid substance that absorbs the liquid is the imbibant.
- Imbibants are typically hydrophilic colloidal particles such as proteins, pectins, starches, and cellulose. These particles retain water on their surfaces and within the microscopic spaces between them.
- Although water is the primary liquid involved in plant imbibition, other substances can act as imbibants under different circumstances. For instance, rubber imbibes ether or kerosene instead of water.
- Imbibition Capacity:
- Different materials have varying capacities for imbibition. For example, agar-agar, a type of phycocolloid, has the highest imbibition capacity, able to absorb nearly 99 times its weight in water. Proteins are also effective, absorbing up to 15 times their own volume of water.
- On the other hand, cellulose and starch exhibit much lower imbibition capacities, and lignin is nearly incapable of imbibing water.
- This variance is observable in everyday life: seeds such as gram (which are protein-rich) swell much more when soaked compared to starchy seeds like rice or wheat.
- Physical Changes during Imbibition:
- As imbibition takes place, water is held within the solid particles, causing the imbibant to lose the kinetic energy associated with the water molecules. This lost kinetic energy is released as heat, referred to as the heat of wetting. For example, kneading wheat flour can result in a noticeable increase in temperature.
- Additionally, the imbibant swells as it absorbs water, though the increase in volume is less than the volume of water absorbed.
- Imbibition Pressure:
- The process of imbibition creates imbibition pressure, which is the pressure developed in an imbibant when it is brought into contact with water, without allowing the imbibant to expand. This pressure can be extremely high.
- Imbibition pressure is also referred to as matric potential, and dry seeds can have a matric potential of around -100 bars. When exposed to water, seeds such as peas can generate imbibition pressures as high as 1000 atm.
- This principle has practical applications, such as in ancient times when dry wood was used to split rocks. When the wood absorbed water, it swelled, exerting enough pressure to crack the rocks.
- Factors Influencing Imbibition:
- Temperature: A rise in temperature increases the rate of imbibition, as higher temperatures enhance the kinetic energy of the imbibant particles.
- Texture of the Imbibant: The molecular cohesion of the imbibant affects its imbibition capacity. Materials with loosely packed particles, such as gelatin, will absorb much more water than denser materials like hardwood.
- pH of the Medium: Since imbibants are usually colloidal in nature, the pH of the surrounding medium significantly impacts the process. Negatively charged colloids like cellulose imbibe water most effectively in alkaline conditions. Proteins, being amphoteric, exhibit maximum absorption in either acidic or alkaline conditions, but least absorption in neutral conditions.
- Presence of Electrolytes: Electrolytes slow down the rate of imbibition by neutralizing the charges of the imbibant, reducing its capacity to attract and hold water.
- Biological Importance of Imbibition:
- Seed Germination: Imbibition is critical for seed germination as water must be absorbed by dry seeds to trigger the necessary metabolic processes. This absorption often leads to the breaking of the seed coat.
- Dehiscence in Fruits: Imbibition plays a role in the opening of dehiscent fruits, such as legume pods and cotton bolls, through a process known as xerochasy, which occurs under dry conditions.
- Hygroscopic Movements: In some plants, such as bryophytes, elaters (specialized structures) undergo hygroscopic movements influenced by imbibition, allowing them to disperse spores effectively.
3. Osmosis
Osmosis is a fundamental biological process, first identified by Nollet in 1748, who observed the swelling and eventual bursting of a wine-filled bladder when placed in water. This phenomenon results from the movement of water across a semipermeable membrane, driven by differences in concentration. Osmosis involves the diffusion of water or another solvent from an area of lower solute concentration (dilute solution) to an area of higher solute concentration (stronger solution).
- Definition and Mechanism:
- Osmosis is the diffusion of water through a semipermeable membrane, which allows only certain molecules, like water, to pass through while blocking others. The movement of water during osmosis is influenced by concentration gradients and pressure differences on either side of the membrane.
- A semipermeable membrane plays a critical role in osmosis, regulating the movement of water between two compartments, ensuring water flows from the area with lower solute concentration to the area with higher solute concentration until equilibrium is reached.
- Types of Osmosis:
- Endosmosis refers to the inward movement of water into a cell or system, leading to swelling. For example, water-soaked raisins swell due to endosmosis.
- Exosmosis involves the outward movement of water from a cell in response to a hypertonic solution, resulting in shrinkage. Fresh grapes placed in a concentrated salt or sugar solution undergo exosmosis, causing them to shrink and wrinkle.
- Thistle Funnel Experiment:
- The classic thistle funnel experiment demonstrates osmosis. A thistle funnel filled with a concentrated solution is placed in a container of water. Over time, water enters the funnel through the semipermeable membrane, causing the liquid level inside the funnel to rise, visibly illustrating the osmotic movement of water.
- Osmotically Active Solutions:
- A solution that causes water to move into it is referred to as osmotically active. The movement of water into such solutions is driven by concentration differences and occurs through the semipermeable membrane.
- Types of Solutions Based on Osmosis:
- Hypertonic Solution: This is a solution with a higher concentration of solutes compared to the cell’s internal environment (cell sap). When a cell is placed in a hypertonic solution, water exits the cell through exosmosis, causing the protoplasm to shrink, a process known as plasmolysis.
- Hypotonic Solution: A hypotonic solution has a lower concentration of solutes compared to the cell sap. When a cell is immersed in such a solution, water enters the cell through endosmosis, leading to an increase in cell volume.
- Isotonic Solution: In an isotonic solution, the concentration of solutes outside the cell matches that inside the cell sap. As a result, there is no net movement of water, and the cell volume remains unchanged.
4. Membrane Permeability
Membrane Permeability refers to the ability of a membrane to control or restrict the movement of substances through it, a fundamental characteristic of all biological membranes. This property determines how solutes and solvents pass through different membranes, impacting cellular functions and the overall physiology of an organism. Various types of membranes exhibit distinct permeability characteristics, depending on their structure and composition.
- Types of Membranes Based on Permeability:
- Permeable membranes allow both solute and solvent particles to pass freely. An example of a permeable membrane is the cellulosic cell wall, which enables the unrestricted movement of substances.
- Impermeable membranes do not permit any substance, whether solute or solvent, to pass through. Cork cells with suberized walls or cutinized walls serve as examples of impermeable membranes, effectively blocking the passage of all materials.
- Semipermeable membranes are selective, allowing only the solvent, typically water, to move through while restricting the passage of solute particles. Common examples of semipermeable membranes include parchment, copper ferrocyanide, and collodion membranes.
- Plasma Membrane and Selective Permeability:
- Biological membranes such as the plasma membrane, tonoplast, and membranes surrounding organelles exhibit differential or selective permeability. These membranes allow the movement of both water (solvent) and specific solutes, but only through specialized mechanisms. This selective transport is vital for regulating the internal environment of cells and organelles.
- The plasma membrane’s selective permeability arises from its complex lipoprotein structure, which includes specialized proteins responsible for controlling substance movement. This ensures that essential substances like water and ions enter the cell while harmful or unnecessary compounds are kept out.
- Mechanisms of Selective Transport:
- Aquaporins are specialized protein-lined channels responsible for the efficient absorption and movement of water through membranes. These channels regulate water balance in cells, making them essential for osmoregulation and hydration processes.
- Ion channels, approximately 30 kDa in size, are responsible for the transport of ions across membranes. These channels selectively permit the movement of ions such as sodium (Na+), potassium (K+), and calcium (Ca2+), maintaining electrical gradients and facilitating various cellular processes like signal transduction and muscle contraction.
5. Osmotic Pressure
Osmotic Pressure is the hydrostatic pressure that develops in a solution, preventing the flow of water or another solvent from a dilute solution into a more concentrated solution through a semipermeable membrane. It can be described as the maximum pressure required to stop osmosis. Osmotic pressure is crucial in regulating the movement of water and solutes across biological membranes, which is essential for maintaining cellular homeostasis.
- Definition and Concept:
- Osmotic pressure (OP) represents the force necessary to prevent water or solvent movement from a pure solvent to a solution when separated by a semipermeable membrane. It can also be seen as the pressure that an osmotically active solution develops when interacting with its pure solvent across a semipermeable barrier.
- OP is often measured using instruments like Pfeffer’s and Hartley’s osmometers. Pfeffer’s osmometer utilizes very dilute solutions, while Hartley’s osmometer uses external pressure to counter the osmotic pressure, measuring the equilibrium between the two forces.
- Factors Affecting Osmotic Pressure:
- OP is directly proportional to the concentration of solutes in a solution. This relationship is expressed by van’t Hoff’s equation: OP=mRT or OP=cRTOP = mRT \text{ or } OP = cRTOP=mRT or OP=cRT Where:
- m/c = molar concentration of the solute,
- R = universal gas constant (0.082 atm/mol),
- T = absolute temperature in Kelvin (K).
- As an example, a 1 mole glucose solution at 0°C generates an osmotic pressure of approximately 22.4 atm. At 20°C, the pressure increases to about 24 atm due to the rise in temperature.
- OP is directly proportional to the concentration of solutes in a solution. This relationship is expressed by van’t Hoff’s equation: OP=mRT or OP=cRTOP = mRT \text{ or } OP = cRTOP=mRT or OP=cRT Where:
- Application to Electrolytes and Non-Electrolytes:
- For non-electrolyte solutions, such as glucose or sucrose, osmotic pressure can be directly calculated using the van’t Hoff equation. However, for electrolyte solutions like sodium chloride (NaCl) or potassium chloride (KCl), the degree of dissociation must be considered. This adjustment is made using the ionization constant (i) in the modified formula: OP=ciRTOP = ciRTOP=ciRT
- Ionization increases the number of particles in a solution, meaning electrolytes exert greater osmotic pressure than non-electrolytes at the same molar concentration. For example, a 0.01 M glucose solution has an osmotic pressure of 0.24 atm, while an equimolar NaCl solution has a higher osmotic pressure of around 0.47 atm.
- As a result, water naturally moves from areas of lower osmotic pressure to regions with higher osmotic pressure.
- For non-electrolyte solutions, such as glucose or sucrose, osmotic pressure can be directly calculated using the van’t Hoff equation. However, for electrolyte solutions like sodium chloride (NaCl) or potassium chloride (KCl), the degree of dissociation must be considered. This adjustment is made using the ionization constant (i) in the modified formula: OP=ciRTOP = ciRTOP=ciRT
- Turgor Pressure:
- Turgor pressure (TP), also known as hydrostatic or pressure potential, refers to the pressure exerted by a cell’s internal water content against its cell wall. This only applies to living cells, where water movement creates swelling in the cell due to the osmotic pressure of the cell sap.
- As water enters the cell, the hydrated cytoplasm expands and presses against the cell wall, creating outward force known as turgor pressure. The cell wall, in turn, exerts an equal and opposite force called wall pressure (WP), preventing the cell from bursting. In plant cells, turgor pressure plays a key role in maintaining cell structure and rigidity.
- In a fully turgid cell, turgor pressure equals the osmotic pressure, providing maximum hydrostatic pressure. Conversely, in a flaccid cell, where water has left the cell, turgor pressure becomes zero. Under plasmolytic conditions, turgor pressure may even become negative.
- Functions of Turgor Pressure:
- Turgor pressure is essential for maintaining the hydration and structural integrity of the protoplast and its organelles, ensuring proper cellular functioning. It also plays a vital role in various physiological processes such as cell elongation, seed germination, and several types of plant movements, including seismonastic movements and the regulation of stomatal opening and closing.
6. Diffusion Pressure Deficit (DPD)
Diffusion Pressure Deficit (DPD) refers to the reduction in diffusion pressure of water in a solution compared to pure water, due to the presence of solutes. It plays a significant role in water movement and absorption in cells, determining the direction and intensity of osmotic processes. The concept of DPD helps in understanding the water relations within plant cells, particularly their ability to absorb water.
- Definition and Concept:
- DPD is the difference between the diffusion pressure of pure water and that of a solution at the same temperature and pressure. Pure water has the highest diffusion pressure, and the addition of solutes lowers this pressure. DPD represents the potential of a cell or solution to absorb water, with higher DPD indicating a greater need for water.
- The term “Diffusion Pressure Deficit” was introduced by Meyer in 1938, replacing the earlier term “Suction Pressure” (SP) proposed by Renner in 1915. The concept of DPD is considered more appropriate because it reflects the reduced diffusion pressure caused by solutes.
- Relation to Osmotic Pressure and Turgor Pressure:
- DPD is calculated as the difference between osmotic pressure (OP) and wall pressure (WP), which is equal to turgor pressure (TP). Therefore, the equation for DPD is: DPD=OP−TPDPD = OP – TPDPD=OP−TP
- Osmotic Pressure (OP): The pressure exerted by solutes in a solution, driving the movement of water into the cell.
- Turgor Pressure (TP): The pressure exerted by the cell’s contents against the cell wall, which counteracts osmotic pressure.
- DPD is calculated as the difference between osmotic pressure (OP) and wall pressure (WP), which is equal to turgor pressure (TP). Therefore, the equation for DPD is: DPD=OP−TPDPD = OP – TPDPD=OP−TP
- Water Movement and Turgidity:
- When a cell is placed in pure water or a hypotonic solution (less concentrated than the cell sap), water enters the cell due to the higher DPD of the cell. As water enters, turgor pressure increases, and osmotic pressure decreases as the concentration of solutes in the cell decreases. Eventually, when TP equals OP, the cell becomes fully turgid, and DPD becomes zero: DPD=OP−TP=0DPD = OP – TP = 0DPD=OP−TP=0
- In this fully turgid state, the cell can no longer absorb water, as the forces driving water movement have balanced out.
- When a cell is placed in pure water or a hypotonic solution (less concentrated than the cell sap), water enters the cell due to the higher DPD of the cell. As water enters, turgor pressure increases, and osmotic pressure decreases as the concentration of solutes in the cell decreases. Eventually, when TP equals OP, the cell becomes fully turgid, and DPD becomes zero: DPD=OP−TP=0DPD = OP – TP = 0DPD=OP−TP=0
- Plasmolysis and DPD in Hypertonic Solutions:
- When a cell is placed in a hypertonic solution (more concentrated than the cell sap), water exits the cell by exosmosis, causing the cytoplasm to shrink and the protoplast to detach from the cell wall, leading to plasmolysis. As water leaves, osmotic pressure increases and turgor pressure decreases, eventually reaching zero when the cell becomes completely flaccid. At this stage, DPD equals OP: DPD=OPDPD = OPDPD=OP
- In the fully flaccid state, the cell has its maximum absorptive capacity because it has the highest potential to take in water.
- When a cell is placed in a hypertonic solution (more concentrated than the cell sap), water exits the cell by exosmosis, causing the cytoplasm to shrink and the protoplast to detach from the cell wall, leading to plasmolysis. As water leaves, osmotic pressure increases and turgor pressure decreases, eventually reaching zero when the cell becomes completely flaccid. At this stage, DPD equals OP: DPD=OPDPD = OPDPD=OP
- DPD in Hypotonic Solutions:
- If a cell is placed in a hypotonic solution other than pure water, the DPD is calculated differently. The equation becomes: DPD=(OP−OP1)−TPDPD = (OP – OP_1) – TPDPD=(OP−OP1)−TP
- Where OP₁ is the osmotic pressure of the hypotonic solution. This equation accounts for the difference between the osmotic pressure of the cell and the surrounding solution.
- If a cell is placed in a hypotonic solution other than pure water, the DPD is calculated differently. The equation becomes: DPD=(OP−OP1)−TPDPD = (OP – OP_1) – TPDPD=(OP−OP1)−TP
- Negative Turgor Pressure:
- In certain situations, turgor pressure may become negative, particularly in plasmolyzed cells where the cell wall exerts a pulling force in the opposite direction. In these cases, the equation for DPD becomes: DPD=OP+TPDPD = OP + TPDPD=OP+TP
- This means that the DPD of a plasmolyzed cell can exceed the osmotic pressure, as the negative turgor pressure contributes to the overall water deficit within the cell.
- In certain situations, turgor pressure may become negative, particularly in plasmolyzed cells where the cell wall exerts a pulling force in the opposite direction. In these cases, the equation for DPD becomes: DPD=OP+TPDPD = OP + TPDPD=OP+TP
- Role of DPD in Water Movement:
- DPD determines the water-absorbing or water-losing capacity of a cell or osmotic system. Unlike osmotic pressure (OP) and turgor pressure (TP) considered separately, DPD integrates both pressures, acting as the driving force for water movement. Water always moves from areas of lower DPD to areas of higher DPD, guiding osmotic processes within plant cells.
7. Chemical Potential
Chemical Potential is a measure of a substance’s free energy within a system, indicating its capacity to perform work. In thermodynamics, a system refers to the set of interacting components being studied, such as a solution in a container, where individual molecules interact to influence overall properties. Chemical potential represents the energy available for work within a system and is crucial for understanding processes like diffusion, osmosis, and chemical reactions.
- Definition of Chemical Potential:
- The chemical potential of a substance in a system reflects the free energy associated with that substance. Free energy is defined as the energy available to perform work, calculated as force multiplied by distance. In simpler terms, chemical potential measures the energy per mole of a substance and is expressed in joules per mole (J mol⁻¹).
- For instance, in pure water, the molecules possess maximum free energy since they are uninfluenced by any other substances. However, when solutes like sugar are added to water, the free energy of water decreases because some water molecules become associated with the sugar molecules, lowering their ability to perform work.
- Relative Nature of Chemical Potential:
- Chemical potential is a relative measure, indicating the difference in free energy between a substance in its current state and in a standard state. The standard state often refers to pure conditions, such as pure water under standard temperature and pressure (STP). The addition of solutes to water reduces its chemical potential, meaning that pure water has the highest chemical potential, while solutions have progressively lower potentials.
- Chemical Potential and Vapor Pressure:
- The chemical potential of water in a solution is directly related to its vapor pressure. Vapor pressure is the pressure exerted by a liquid in equilibrium with its vapor. The relationship between chemical potential (µ) and vapor pressure is expressed mathematically as: μw−μw°=RTln(ee°)μ_w – μ_w° = RT \ln \left( \frac{e}{e°} \right)μw−μw°=RTln(e°e)
- Where:
- µw = chemical potential of water in the solution.
- µw° = chemical potential of pure water at standard temperature and pressure.
- R = gas constant.
- T = absolute temperature (in Kelvin).
- e = vapor pressure of water in the solution.
- e° = vapor pressure of pure water.
- Where:
- The chemical potential of water in a solution is directly related to its vapor pressure. Vapor pressure is the pressure exerted by a liquid in equilibrium with its vapor. The relationship between chemical potential (µ) and vapor pressure is expressed mathematically as: μw−μw°=RTln(ee°)μ_w – μ_w° = RT \ln \left( \frac{e}{e°} \right)μw−μw°=RTln(e°e)
- Chemical Potential of Pure Water:
- When dealing with pure water, the vapor pressure ratio ee°\frac{e}{e°}e°e becomes 1, leading to a logarithmic value of 0. Thus, the change in chemical potential (∆µ) is zero for pure water, and its chemical potential is set to zero by convention. In any other case, where solutes are present, the vapor pressure of water decreases, making ee°\frac{e}{e°}e°e a fraction, and the change in chemical potential (∆µ) becomes negative.
- Flow of Water Based on Chemical Potential:
- Water will naturally move from regions of higher chemical potential to regions with lower chemical potential. In practice, this means water will flow from areas of pure water (or solutions with fewer solutes) to areas with more concentrated solutions, where the chemical potential of water is lower. This flow occurs spontaneously as the system seeks equilibrium, distributing energy evenly across the solution.
- Effects of Solutes on Chemical Potential:
- The addition of solutes to water reduces its free energy or chemical potential. Since chemical potential is a measure of the energy available for work, adding solutes makes the water less able to perform work, effectively lowering its energy. This reduction in energy is why the chemical potential in a solution is always negative compared to pure water, where it is at its maximum (zero).
8. Components of Water Potential
Components of Water Potential are integral to understanding the movement of water within a system, especially in plant physiology. Water potential (Ψw) measures the difference between the chemical potential of water at any point in a system and that of pure water under standard temperature and pressure (STP). This concept is critical because water moves spontaneously from regions of higher water potential to lower water potential. Water potential is influenced by several factors and is expressed in pressure units, commonly joules per cubic centimeter (J cm⁻³). The major components affecting water potential include solute concentration, pressure, matric forces, and gravity.
- Solute Potential (Ψs):
- The effect of solutes on water potential is referred to as solute potential or osmotic potential (Ψπ). When solutes are dissolved in water, they reduce the water’s free energy, thereby lowering its water potential. For example, if pure water in two chambers is separated by a semi-permeable membrane, adding solutes to one chamber reduces the water potential in that chamber. Water will move from the chamber with higher water potential (pure water) to the one with lower water potential (the chamber containing solutes).
- The osmotic potential is expressed as a negative value since the presence of solutes reduces the water potential compared to pure water. Osmotic potential is calculated using the relationship: π=−Ψπ\pi = -\Psi_{\pi}π=−Ψπ
- For example, a solution with an osmotic pressure of 5 bars will have an osmotic potential of –5 bars. Thus, solute potential represents the reduction in water potential due to the dissolved particles in the solution, whether ionic or non-ionic.
- Pressure Potential (Ψp):
- Pressure potential describes how pressure affects water potential. Applying pressure to a system increases the free energy of the water, raising its potential. When pressure is applied to one side of a semi-permeable membrane, water will move from the high-pressure side to the low-pressure side.
- In plant cells, pressure potential is usually positive and is associated with turgor pressure, which occurs when water presses against the cell walls, helping maintain the plant’s structural integrity. In contrast, in structures like xylem, a negative pressure or tension may develop, lowering the pressure potential. The combined effect of solute potential and pressure potential determines the overall water potential.
- Matric Potential (Ψm):
- Matric potential refers to the effect of surface interactions on water potential. Water can become adsorbed onto solid surfaces, such as soil particles or plant tissues, reducing its free energy. The force between water and these surfaces is called matric pressure, and its effect is always negative.
- Matric potential is significant in certain contexts, such as in dry soils, seeds, or plants adapted to arid environments (xerophytes). In well-watered conditions, matric potential may be negligible, but in dry conditions, it plays a vital role in determining water potential.
- Gravitational Potential (Ψg):
- Gravitational potential takes into account the effect of gravity on water movement. Water naturally moves downward due to gravitational force. While this component is typically small and often negligible in cellular-level processes, it becomes more relevant in larger-scale movements, such as water transport in tall trees.
- Overall Water Potential:
- Water potential (Ψw) is the algebraic sum of its components: Ψw=Ψs+Ψp+Ψm+Ψg\Psi_w = \Psi_s + \Psi_p + \Psi_m + \Psi_gΨw=Ψs+Ψp+Ψm+Ψg
- The overall water potential is influenced by the collective effects of solute potential, pressure potential, matric potential, and gravitational potential. In most biological systems, solute and pressure potentials are the dominant factors, with matric and gravitational potentials being significant in specific conditions like soil water movement.
- Water potential (Ψw) is the algebraic sum of its components: Ψw=Ψs+Ψp+Ψm+Ψg\Psi_w = \Psi_s + \Psi_p + \Psi_m + \Psi_gΨw=Ψs+Ψp+Ψm+Ψg
- Water Movement and Flux:
- Water moves from regions of higher (less negative) water potential to regions of lower (more negative) water potential. This movement occurs spontaneously, driven by the differences in water potential between sources (higher water potential) and sinks (lower water potential). The rate of water movement, or flux, is determined by the permeability of membranes and the water potential gradient.
- Water flux (Jw) can be described by the equation: Jw=LpΔΨwJ_w = L_p \Delta \Psi_wJw=LpΔΨw
- Where:
- LpL_pLp = permeability coefficient of the membrane.
- ΔΨw\Delta \Psi_wΔΨw = difference in water potential between two points.
- Where:
- In plant tissues, water primarily moves through the xylem because it offers the least resistance to water flow, making it the most efficient pathway for long-distance transport. The resistance in xylem is inversely proportional to the diameter of the xylem vessels, with smaller vessels providing greater resistance to water flow.
9. Gradients of Water Potential
Understanding gradients of water potential is crucial for grasping how water moves within plants, from individual cells to the entire organism. Water potential is a measure that combines osmotic potential and pressure potential, dictating the movement of water across different biological levels.
- Movement of Water in a Single Cell:
- Isolated cells, single-celled organisms, and root hairs absorb water directly from their surrounding media. For instance, in a typical parenchymatous cell, the vacuole can occupy up to 90% of the cell’s volume, containing cell sap, which is a dilute solution of salts and small molecules.
- Due to osmotic potential, the water potential of the cell sap is lower than that of pure water. When such a cell is placed in pure water, a gradient forms because of the disparity in water potential, prompting water to move into the cell.
- As water enters, the concentration of the sap decreases, which in turn lowers its osmotic potential. Consequently, the difference in potential between pure water and cell sap diminishes, reducing the force driving water entry into the cell.
- This relationship can be expressed by the equation:
Ψw (outside the cell)=Ψπ+Ψp (inside the cell)\Psi_w \text{ (outside the cell)} = \Psi_\pi + \Psi_p \text{ (inside the cell)}Ψw (outside the cell)=Ψπ+Ψp (inside the cell)
where Ψw\Psi_wΨw is the total water potential, Ψπ\Psi_\piΨπ is the osmotic potential, and Ψp\Psi_pΨp is the pressure potential. When turgor pressure reaches its maximum, the sum of Ψp\Psi_pΨp and Ψπ\Psi_\piΨπ equals zero, leading to Ψw\Psi_wΨw also being zero.
- Driving Force of Water Movement:
- The driving force (F) causing water movement can be represented as:
F=gradient(Ψc−Ψe)F = \text{gradient} (\Psi_c – \Psi_e)F=gradient(Ψc−Ψe)
where Ψc\Psi_cΨc is the total water potential of the cell (including the cell sap) and Ψe\Psi_eΨe is the total water potential of the external medium. If the external medium is pure water, Ψe\Psi_eΨe is zero, making the driving force equal to Ψc\Psi_cΨc. - As water enters the cell, Ψc\Psi_cΨc adjusts based on Ψπ\Psi_\piΨπ and Ψp\Psi_pΨp. The cell wall’s elasticity also significantly influences this dynamic, as the cell’s volume can increase up to a limit with sap dilution, subsequently affecting the total osmotic potential.
- The driving force (F) causing water movement can be represented as:
- Water Relations in Root and Leaf Cells:
- Within root and leaf cells, the external medium comprises water in the cell walls and intercellular spaces (the apoplast), which is generally under atmospheric pressure and has low osmotic potential. The matric forces exerted by the cell walls play a significant role here, influencing the water potential in the apoplast.
- It is vital to recognize that the osmotic status of a cell adjusts in response to water gain or loss, illustrated in the Hofler diagram, which relates Ψw\Psi_wΨw, Ψs\Psi_sΨs, and Ψp\Psi_pΨp to water movement dynamics. When a cell is placed in a hypotonic solution, endosmosis causes the central vacuole to expand, increasing turgor pressure (TP). At full turgidity, TP equals osmotic pressure (OP), leading to zero diffusion pressure deficit (DPD) and halting further water entry into the cell. The relevant relationships can be summarized as follows:
- At full turgidity:
- DPD=OP−TPDPD = OP – TPDPD=OP−TP
- Ψw=(−)Ψs−(+)Ψp\Psi_w = (-)\Psi_s – (+)\Psi_pΨw=(−)Ψs−(+)Ψp
- Ψw=Ψs+Ψp\Psi_w = \Psi_s + \Psi_pΨw=Ψs+Ψp
- TP=OPTP = OPTP=OP [Ψs=Ψp\Psi_s = \Psi_pΨs=Ψp]
- DPD=0DPD = 0DPD=0
- Ψw=0\Psi_w = 0Ψw=0
- Conversely, when immersed in a hypertonic solution, a turgid cell loses water, causing the solute potential of the cell sap to become more negative and the pressure potential to decrease, leading to maximal decline in water potential.
- At full turgidity:
- Water Relations of Tissues:
- In higher plants, cells are interconnected and not isolated. For example, a root hair is surrounded by other cells, and its water relations are influenced by both the surrounding medium and adjacent cells.
- Considering two adjacent cells, A and B, if their total water potentials (Ψw\Psi_wΨw) are identical, no net water exchange occurs. The total water potential can be expressed as:
ΨwA=ΨπA+ΨpA\Psi_wA = \Psi_\pi A + \Psi_p AΨwA=ΨπA+ΨpA
ΨwB=ΨπB+ΨpB\Psi_wB = \Psi_\pi B + \Psi_p BΨwB=ΨπB+ΨpB - The driving force (F) for water movement between these cells is given by:
F=(ΨwA−ΨwB)F = (\Psi_wA – \Psi_wB)F=(ΨwA−ΨwB) - If ΨwA\Psi_wAΨwA equals ΨwB\Psi_wBΨwB, the gradient and thus the driving force (F) is zero, resulting in no water movement. However, if ΨwB\Psi_wBΨwB is lower than ΨwA\Psi_wAΨwA, water will flow into cell B until equilibrium is reached. This principle can be expanded to larger groups of interconnected cells within a tissue.
- Water Relations of a Whole Plant:
- Examining the water relations of an entire plant, including leaves, stems, and roots, reveals a continuum facilitating water movement within the soil-plant-atmosphere system. The total water potential in the atmosphere can be significantly lower, affected by temperature and humidity.
- For instance, approximate water potential values are:
- Soil: -0.1 to -20.0 bars
- Leaf: -5.0 to -50.0 bars
- Atmosphere: -100 to -2000 bars
- This data indicates that the difference in total water potential across the soil-plant-atmosphere continuum creates a driving force for water movement from soil through the plant to the atmosphere. If this continuum is disrupted, the driving force for water movement ceases, demonstrating the essential interconnectedness of these components in plant water relations.