What is Oxidative Phosphorylation?
- Oxidative Phosphorylation, commonly abbreviated as OXPHOS, is a critical biochemical process in cellular respiration, particularly within the mitochondria of eukaryotic cells. This process is responsible for the generation of adenosine triphosphate (ATP), the primary energy currency of the cell. The intricacies of oxidative phosphorylation involve multiple steps and components, each playing a pivotal role in energy production.
- Central to this process are redox reactions, where electrons are transferred through a series of membrane-bound proteins within the inner mitochondrial membrane. This electron transfer is pivotal for the generation of ATP. The process begins with the oxidation of electron carriers, specifically nicotinamide adenine dinucleotide (NADH) and flavin adenine dinucleotide (FADH2). These molecules are key players in the process, having gained electrons during earlier stages of cellular respiration such as the citric acid cycle.
- Once oxidized, these carriers release electrons, which travel through the electron transport chain, a series of protein complexes located within the inner mitochondrial membrane. This electron transfer is coupled with the transport of protons (H+) across the mitochondrial membrane, creating a proton gradient. This gradient is a form of potential energy, critical for the next phase of the process.
- The final stage of oxidative phosphorylation involves the enzyme ATP synthase, which harnesses the energy from the proton gradient. As protons flow back across the membrane through ATP synthase, the enzyme catalyzes the synthesis of ATP from adenosine diphosphate (ADP) and an inorganic phosphate. This process, known as chemiosmosis, is fundamental to the conversion of the energy stored in nutrient bonds into a form usable by the cell.
- Besides ATP production, oxidative phosphorylation has a byproduct of water, formed when the electrons eventually combine with molecular oxygen. It is noteworthy that oxygen is the final electron acceptor in the chain, underscoring the aerobic nature of this process.
- In summary, oxidative phosphorylation is a complex, yet highly efficient process that converts the energy stored in reduced cofactors into ATP, a versatile and readily usable form of energy for cellular functions. This process is not only fundamental to energy metabolism in cells but also highlights the sophisticated mechanisms of energy transfer and utilization in biological systems.
Definition of Oxidative Phosphorylation
Oxidative phosphorylation is a metabolic process that occurs in the mitochondria, where energy derived from the electron transport chain is used to produce adenosine triphosphate (ATP) from adenosine diphosphate (ADP) and inorganic phosphate, with oxygen acting as the final electron acceptor.
Sites of oxidative phosphorylation in ETC
- Oxidation of FMNH2 by Coenzyme Q
- The first site of oxidative phosphorylation in the Electron Transport Chain (ETC) involves the oxidation of FMNH2 by coenzyme Q (CoQ). In this reaction, FMNH2 is oxidized, meaning it loses electrons, which are then transferred to CoQ. This electron transfer process is exergonic, releasing energy.
- Coenzyme Q, also known as ubiquinone, plays a pivotal role as a mobile electron carrier within the mitochondrial membrane. It effectively accepts electrons from FMNH2 and shuttles them to the next complex in the ETC.
- Oxidation of Cytochrome b by Cytochrome c1
- The second site is characterized by the oxidation of cytochrome b by cytochrome c1. In this phase, electrons are transferred from cytochrome b to cytochrome c1. This transfer is again an exergonic reaction, facilitating the release of energy.
- Cytochromes are proteins containing heme groups, and their role in the ETC is crucial. They function as electron carriers, with cytochrome b being part of a larger complex known as Complex III or the cytochrome bc1 complex, and cytochrome c1 acting as an intermediate in electron transfer.
- Cytochrome Oxidase Reaction
- The third and final site involves the cytochrome oxidase reaction. This reaction is a part of Complex IV of the ETC, where electrons are transferred to molecular oxygen, resulting in the formation of water.
- Cytochrome oxidase is a large enzyme complex that catalyzes the reduction of oxygen to water. It is the last electron acceptor in the chain and is crucial for maintaining the efficiency of the ETC.
Coupling Sites for ATP Production
- It is important to note that each of these reactions represents a coupling site for ATP production. However, the oxidation of FADH2, another electron donor in the ETC, only utilizes two of these coupling sites. This is because the first site, involving FMNH2 and CoQ, is bypassed when FADH2 is the electron donor. Therefore, the P:O ratio (the amount of ATP produced per oxygen atom reduced) for FADH2 is 2, which is lower than that for NADH.
Energetics of oxidative phosphorylation
- Redox Potential and Electron Transport
- The energetics of oxidative phosphorylation begin with the transport of electrons between redox pairs, primarily from NAD+/NADH to the redox pair O2/H2O. The redox potential difference between these two pairs is significant, measured at 1.14 volts (V). This potential difference is foundational for the movement of electrons through the electron transport chain (ETC).
- The simplified equation representing this electron transfer is: 1/2O2+NADH+H+→H2O+NAD+. This reaction underscores the transfer of electrons from NADH (reduced form) to oxygen, resulting in the formation of water.
- Energy Release and ATP Synthesis
- The energy release associated with this electron transfer is approximately 52 Cal/mole. This energy is utilized for synthesizing ATP, the energy currency of the cell. When NADH is oxidized in the ETC, it results in the formation of three molecules of ATP.
- Each ATP molecule is equivalent to 7.3 Calories, making the total energy conserved in the form of ATP approximately 21.9 Calories (3 ATP x 7.3 Cal/ATP).
- Efficiency of Energy Conservation
- The efficiency of energy conservation in oxidative phosphorylation is calculated by comparing the energy conserved in ATP with the total energy released during electron transfer. This is calculated as 21.9/52×100≈42%. Therefore, when NADH is oxidized, about 42% of the energy is trapped in the form of ATP.
- The remaining 58% of the energy is not conserved as ATP and is released as heat. However, this release of heat is not merely a wasteful byproduct. Instead, it plays a crucial role in maintaining the continuous operation of the ETC and in generating ATP. Moreover, this heat release is essential for maintaining the body’s temperature.
Fundamentals of Oxidative Phosphorylation
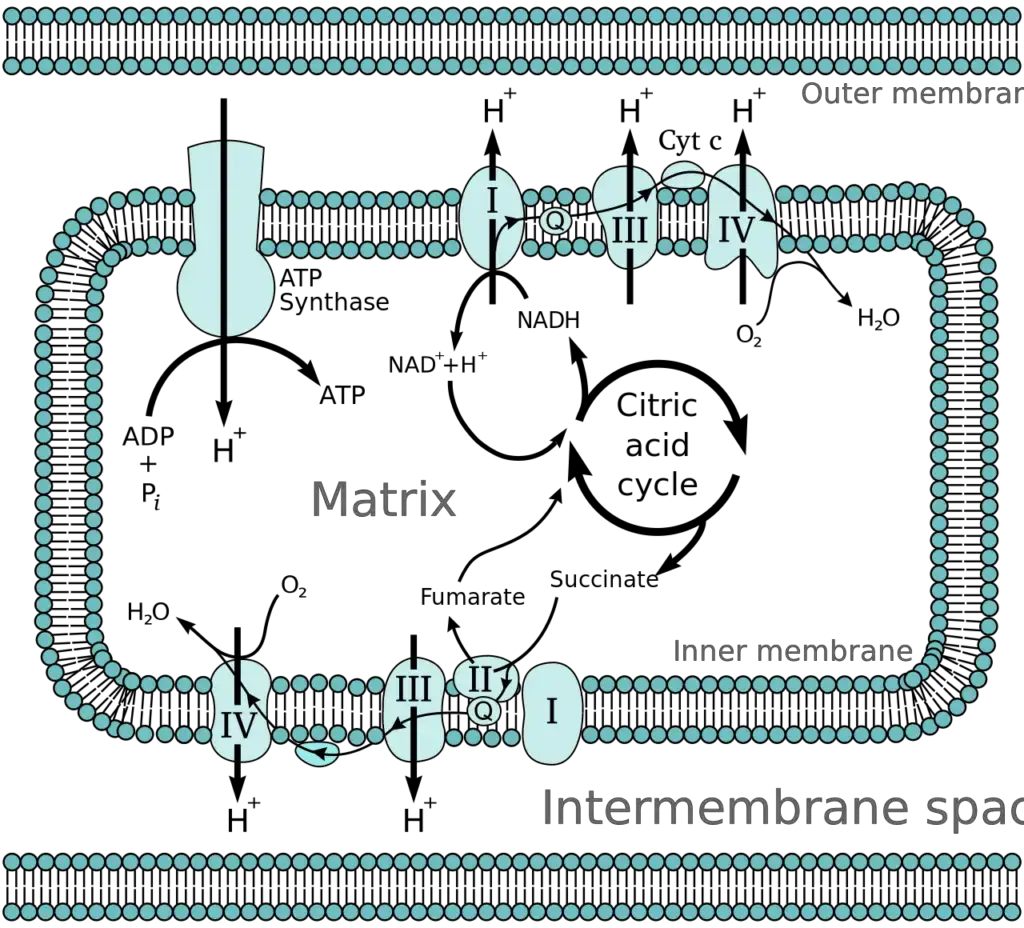
Oxidative phosphorylation, a crucial process in cellular metabolism, can be understood through its fundamental concepts, which include electronegativity, the sources of electron carriers, and the anatomy of the mitochondrion.
- Electronegativity
- Electronegativity refers to an element’s ability to attract a bonding pair of electrons. Elements with high electronegativity can attract electrons more readily.
- Oxygen, due to its high electronegativity and low molecular mass, is the primary final electron acceptor in oxidative phosphorylation. This is facilitated by its abundant availability in the atmosphere.
- Sources of Reduced NADH and FADH2
- Nicotinamide adenine dinucleotide (NAD+) and Flavin adenine dinucleotide (FAD+) are essential electron carrier coenzymes. They are present in both the cell cytoplasm and the mitochondrial matrix.
- These coenzymes are reduced to energy-rich forms, NADH and FADH2, during various metabolic reactions, including glycolysis, the tricarboxylic acid (citric acid) cycle, and beta-oxidation of fatty acids.
- The reduced forms, NADH and FADH2, are shuttled to the mitochondrion, where they donate high-energy electrons to the protein complexes in the electron transport chain.
- Anatomy of the Mitochondrion
- The mitochondrion consists of an outer and an inner membrane, both comprising phospholipid bilayers and integral proteins for enzymatic action and molecular transport.
- The inner membrane features fold-like projections called cristae, which increase the surface area for energy production.
- Protein complexes of the electron transport chain are located along the inner membrane.
- The mitochondrial matrix, enclosed by the inner membrane, contains mitochondrial DNA, ribosomes, enzymes, and metabolites.
- The intermembrane space, situated between the inner and outer membranes, is crucial for the accumulation of hydrogen ions (H+ ions) during the electron transport chain. This accumulation creates a membrane potential across the inner mitochondrial membrane, essential for ATP synthesis.
Cellular Level and Molecular Level of Oxidative Phosphorylation
Oxidative phosphorylation, a critical process in cellular metabolism, can be understood at both cellular and molecular levels:
Cellular Level
- Coupling of Electron Transfer and ATP Synthesis
- Oxidative phosphorylation couples the exergonic (energy-releasing) electron transfer from NADH to oxygen with the endergonic (energy-consuming) process of ATP synthesis.
- This coupling ensures that catabolic reactions (utilizing ATP) are synchronized with the anabolic reaction of ATP synthesis.
- The efficiency of the electron transport chain (ETC) in generating ATP (approximately 30 – 32 ATP molecules from one molecule of glucose) is significantly higher than that of glycolysis alone (2 ATP).
- Chemiosmosis and Proton Motive Force
- The ETC creates a proton gradient by pumping hydrogen ions (H+) into the intermembrane space of mitochondria, a process known as chemiosmosis.
- This creates an electrochemical gradient termed the ‘proton motive’ force, essential for ATP synthesis.
- Function of ATP Synthase (Complex V)
- ATP synthase harnesses the energy of the proton motive force to synthesize ATP.
- The energy used in pumping H+ ions is balanced by the energy generated by ATP synthase, adhering to the first law of thermodynamics.
Molecular Level
- Protein Complexes in the Electron Transport Chain
- The ETC involves four protein complexes (Complex I, II, III, IV) that function as enzymes facilitating electron transfer through oxidation-reduction reactions.
- Complexes I, III, and IV are responsible for pumping protons into the intermembrane space.
- The exact mechanism of proton pumping involves conformational changes in these protein complexes and is an ongoing area of research.
- Components of the Protein Complexes
- These complexes consist of multiple molecules, including iron-sulfur clusters (Fe-S), cytochromes, and prosthetic groups, all crucial in electron transfer.
- Role of Electron Carriers
- Electron carriers like cytochrome C (hydrophilic) and coenzyme Q10 (hydrophobic) shuttle electrons between the complexes.
- Cytochrome C, with its heme group structure, transfers individual electrons between complexes.
- Coenzyme Q10, or ubiquinone in its oxidized form, carries hydrogen ions and electrons, transforming into ubiquinol (QH2) when reduced.
- Genetic Information for the ETC
- The genetic information for the ETC complexes and electron carriers is housed in both mitochondrial DNA (mDNA) and nuclear DNA.
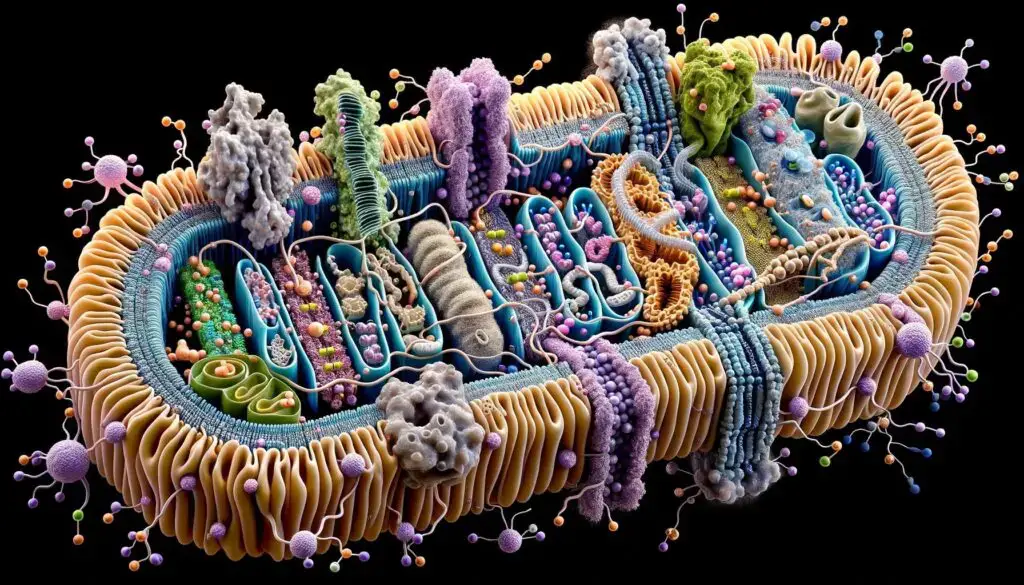
What is an Electron Transport Chain?
- The electron transport chain (ETC) is a sophisticated assembly of protein complexes strategically positioned within the inner mitochondrial membrane. Comprising four primary complexes, designated as I through IV, the ETC is instrumental in the process of oxidative phosphorylation.
- Electrons, primarily derived from NADH and FADH2 molecules produced during glycolysis and the citric acid cycle, are channeled through these complexes in the ETC. As electrons traverse this chain, they transition from a higher to a lower energy state, moving from molecules with a lower electron affinity to those with a higher affinity. This spontaneous transition, characterized as a downhill reaction, results in a decrease in free energy, with the products possessing less energy than the reactants.
- The energy liberated during these electron transitions is adeptly captured and utilized to pump protons (H+) across the inner mitochondrial membrane. This action culminates in the establishment of an electrochemical gradient, a differential concentration of protons between the mitochondrial matrix and the intermembrane space. This gradient is pivotal for the subsequent synthesis of ATP through a process termed chemiosmosis, which involves the movement of ions across a biological membrane.
- The ETC is not just a passive conduit for electrons but is an intricately coordinated series of redox reactions. These reactions involve the transfer of electrons between protein complexes and organic molecules embedded within the inner mitochondrial membrane. The chain incorporates four primary complexes, along with two mobile electron carriers, namely ubiquinone and cytochrome C. It’s noteworthy that while eukaryotic cells utilize ubiquinone as an electron carrier, plants employ a closely related compound called plastoquinone, and certain bacteria utilize menaquinone for this purpose.
- In essence, the electron transport chain serves as the cellular machinery that harnesses the energy from electron transitions, facilitating the creation of an electrochemical gradient. This gradient, in turn, powers the production of ATP, the cell’s primary energy currency, underscoring the ETC’s central role in cellular energy metabolism.
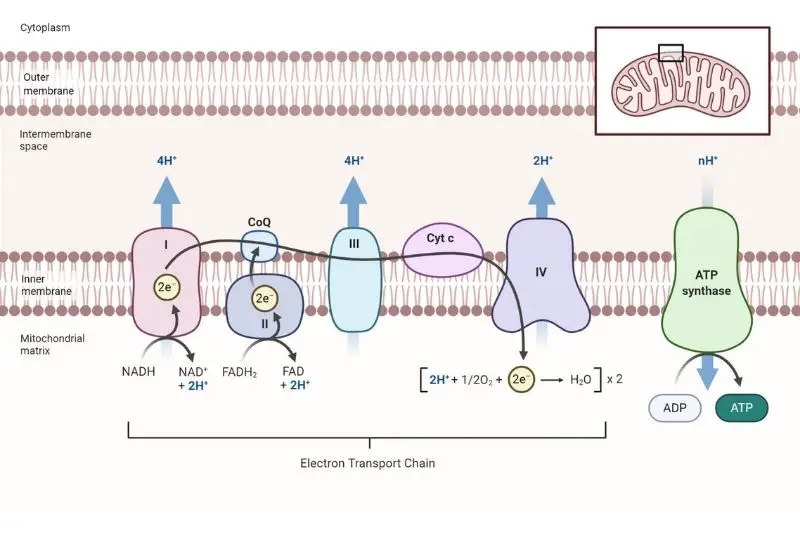
Steps of Electron Transport Chain
The Electron Transport Chain (ETC) is a meticulously organized series of protein complexes, embedded within the inner mitochondrial membrane, which orchestrates a crucial phase in oxidative phosphorylation. This chain, comprising complexes I through IV, functions as a conduit for electrons derived from NADH and FADH2, which are generated during preceding metabolic pathways, namely glycolysis and the citric acid cycle.
- Complex I: NADH Dehydrogenase – The initial complex, denoted as Complex I or NADH dehydrogenase, facilitates the transfer of electrons from NADH to ubiquinone (Q). NADH, being a potent electron donor, relinquishes its electrons, which traverse through a series of redox reactions within the complex, thereby reverting NADH to its oxidized form, NAD+. The energy liberated during these electron transitions is harnessed to pump protons (H+) from the mitochondrial matrix to the intermembrane space, establishing an electrochemical gradient.
- Complex II: Succinate Dehydrogenase – Subsequently, Complex II or succinate dehydrogenase, engages in the oxidation of FADH2, a molecule that donates electrons at a lower energy level compared to NADH. FADH2 is oxidized to FAD, transferring its electrons to ubiquinone. Notably, Complex II does not possess the capability to pump protons across the membrane due to the lower energy release during the electron transfer.
- Ubiquinone and Complex III – Ubiquinone (Q), upon receiving electrons from Complexes I and II, is reduced to ubiquinol (QH2). This molecule then conveys the electrons to Complex III, also known as cytochrome c reductase. This complex facilitates the transfer of electrons to cytochrome c (Cyt c) while concurrently pumping protons across the membrane to further augment the electrochemical gradient.
- Complex IV: Cytochrome c Oxidase – In the subsequent step, cytochrome c transfers the electrons to Complex IV, or cytochrome c oxidase. This complex orchestrates the final electron transfer to oxygen (O2), the terminal electron acceptor. Oxygen, upon accepting electrons, is cleaved into two oxygen ions, which subsequently combine with protons to form water (H2O). This step necessitates four protons to reduce each molecule of O2, yielding two water molecules in the process.
- Complex V: ATP Synthase – Lastly, Complex V, or ATP synthase, utilizes the established proton gradient to synthesize ATP. As protons flow through the F0 subunit of ATP synthase, the F1 subunit is propelled to rotate, facilitating the conversion of ADP and inorganic phosphate into ATP. This mechanistic conversion of potential energy into chemical energy underscores the significance of the ETC in cellular energy production.
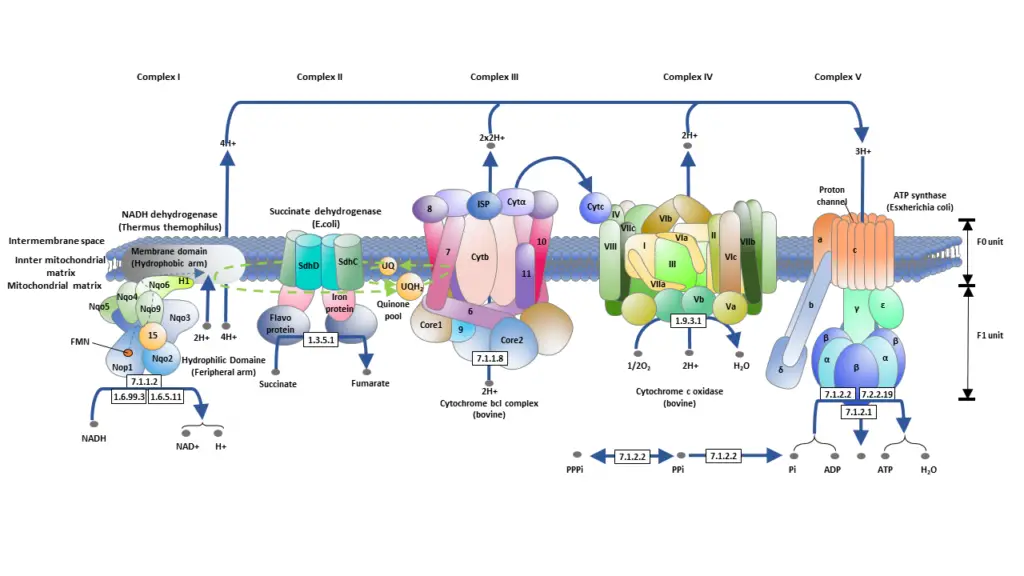
In essence, the ETC is a cascade of redox reactions that facilitate the sequential transfer of electrons through a series of protein complexes, culminating in the synthesis of ATP. This process is not only pivotal for cellular energy production but also exemplifies the intricate biochemical processes that sustain life at the cellular level. The stepwise progression of electrons, driven by the increasing electronegativity of each subsequent complex, underscores the precision and efficiency embedded within cellular respiration.
ATP Synthesis
- Adenosine triphosphate (ATP) synthesis within the mitochondria is a paramount cellular process, underpinning the generation of the cell’s primary energy currency. This synthesis is intricately linked to the establishment of an electrochemical gradient across the inner mitochondrial membrane, commonly referred to as the proton motive force (PMF).
- The genesis of the PMF is rooted in the active pumping of protons (H+) from the mitochondrial matrix to the intermembrane space. This action results in a differential concentration of protons on either side of the inner mitochondrial membrane. Given the hydrophobic nature of the phospholipid bilayer of the membrane, these protons are barred from freely diffusing back into the matrix. Instead, they necessitate specialized channel proteins equipped with hydrophilic tunnels to facilitate their movement.
- ATP synthase, a pivotal enzyme, serves as this exclusive channel. The flow of H+ ions down their electrochemical gradient activates ATP synthase, essentially turning it on. Once activated, ATP synthase harnesses the energy derived from the descending proton gradient to catalyze the phosphorylation of adenosine diphosphate (ADP), appending a phosphate group to yield ATP.
- The efficiency of ATP production is contingent upon the entry point of electrons into the respiratory chain. Electrons entering at complex I facilitate the synthesis of approximately 2.5 ATP molecules, whereas those entering at complex II yield around 1.5 ATP molecules. It’s noteworthy that the ATP generated is subsequently liberated, releasing energy in the form of heat.
- In essence, ATP synthesis epitomizes the cell’s adeptness at converting electrochemical gradients into chemical energy, underscoring the intricate bioenergetics that sustain cellular functions.
What is Chemiosmosis?
Chemiosmosis is a fundamental mechanism that underpins the synthesis of ATP in cells. This process intricately links two distinct sets of reactions: one that releases energy and another that consumes it, ensuring efficient energy transfer and utilization within the cell.
- Coupling of Exergonic and Endergonic Processes: Oxidative phosphorylation exemplifies the harmonious coupling of two contrasting reactions. The flow of electrons through the electron transport chain represents an exergonic process, meaning it releases energy. Conversely, the synthesis of ATP is an endergonic process, which requires an input of energy. The term “exergonic” describes reactions that are spontaneous and release energy to the surroundings, resulting in a negative change in free energy. On the other hand, “endergonic” reactions absorb energy, leading to a positive change in free energy.
- Role of Membranes: The intricate dance of these two processes occurs within a membrane, which serves as a platform for energy transfer. This membrane setting ensures that the energy released by the exergonic process is directly channeled to power the endergonic reaction.
- The Essence of Chemiosmosis: At the heart of this energy transfer is the movement of protons (H+ ions). As electrons traverse the electron transport chain, protons are pumped across the membrane, creating a gradient. This gradient, or proton motive force, is the driving force behind ATP synthesis. As protons flow back across the membrane through ATP synthase, their movement provides the energy required to convert ADP and inorganic phosphate into ATP. This mechanism of harnessing the energy from proton movement to drive ATP synthesis is termed chemiosmosis.
In summary, chemiosmosis is a sophisticated cellular strategy that ensures efficient energy utilization. By coupling energy-releasing and energy-consuming reactions within a membrane, cells can effectively harness and direct energy to where it is most needed, exemplifying the precision and elegance of cellular bioenergetics.
The mechanism of oxidative phosphorylation
The mechanism of oxidative phosphorylation, a key process in cellular respiration, has been elucidated through various hypotheses and models.
- Chemical Coupling Hypothesis
- Proposed by Edward Slater in 1953, this hypothesis suggests that during electron transfer in the respiratory chain, a series of phosphorylated high-energy intermediates are produced, which are then utilized for ATP synthesis. These reactions are considered analogous to substrate-level phosphorylation in glycolysis or the citric acid cycle. However, this hypothesis lacks robust experimental evidence as high-energy intermediates have not been successfully isolated.
- Chemiosmotic Hypothesis
- Introduced by Peter Mitchell in 1961 and now widely accepted, this hypothesis explains the production of ATP from ADP and inorganic phosphate (Pi) through electron transport. It likens the energy stored in the mitochondrial membrane to that in a battery, with separated positive and negative charges.
- Proton Gradient Formation: The inner mitochondrial membrane, impermeable to protons (H+) and hydroxyl ions (OH–), allows electron transport coupled with proton translocation from the matrix to the intermembrane space, creating an electrochemical or proton gradient.
- Enzyme System for ATP Synthesis: ATP synthase, located in Complex V, utilizes this proton gradient to synthesize ATP. ATP synthase, also an ATPase, comprises F1 and F0 subunits and resembles ‘lollipops’ in structure. The proton accumulation in the intermembrane space facilitates ATP synthesis upon their re-entry into the mitochondrial matrix.
- Rotary Motor Model for ATP Generation
- Proposed by Paul Boyer in 1964 (Nobel Prize, 1997), this model posits that conformational changes in mitochondrial membrane proteins drive ATP synthesis. It is also known as the rotary motor/engine driving model or the binding change model.
- ATP synthase, the F0F1 complex of Complex V, consists of channel protein ‘C’ subunits and F1-ATP synthase, which includes a central γ subunit surrounded by alternating α and β subunits.
- Proton flux induces physical rotation of the γ subunit, leading to conformational changes in the β subunits, ultimately resulting in ATP release. The three β subunits of F1-ATP synthase adopt open (O), loose (L), and tight (T) conformations, facilitating the binding of ADP and Pi, ATP synthesis, and ATP release, respectively. This cycle repeats, generating three ATP molecules per revolution.
- Inherited Disorders of Oxidative Phosphorylation
- Oxidative phosphorylation relies on about 100 polypeptides, with 13 coded by mitochondrial DNA (mtDNA) and the rest by nuclear DNA. mtDNA, more prone to mutations than nuclear DNA, is maternally inherited, and mutations are common in tissues with high oxidative phosphorylation rates. Disorders like Leber’s hereditary optic neuropathy, characterized by bilateral vision loss, are examples of mtDNA mutations.
- Inhibitors of the Electron Transport Chain
- Understanding of the ETC has been enhanced by studying the effects of specific inhibitors. These inhibitors block electron transport, causing accumulation of reduced components before the blockade and oxidized components after it. Inhibitors also obstruct ATP formation, with three primary sites of action: between NADH and coenzyme Q; between cytochrome b and c1; and at cytochrome oxidase. Examples include rotenone, antimycin A, and cyanide, with cyanide being a potent inhibitor that binds to cytochrome oxidase, leading to cell death.
Regulation of Oxidative Phosphorylation
Regulation of oxidative phosphorylation is crucial for maintaining cellular energy homeostasis, ensuring that ATP production aligns with the energy demands of the cell. This process is influenced and modulated by various factors and regulatory mechanisms:
- Availability of Oxygen
- Oxygen is the final electron acceptor in the electron transport chain (ETC) of oxidative phosphorylation. The availability of oxygen is, therefore, a critical factor in the regulation of this process. When oxygen levels are high, oxidative phosphorylation proceeds efficiently, facilitating ATP production. Conversely, low oxygen levels, a condition known as hypoxia, can impede the ETC, reducing ATP synthesis.
- Concentrations of ADP and ATP
- The concentrations of ADP (adenosine diphosphate) and ATP (adenosine triphosphate) serve as indicators of the cellular energy state and regulate oxidative phosphorylation accordingly. When ADP levels are high, indicating a need for more ATP, oxidative phosphorylation is upregulated to increase ATP production. In contrast, high levels of ATP signal sufficient energy availability, leading to a downregulation of the process. This regulation is a form of feedback control, maintaining energy balance within the cell.
- Proton Gradient Across the Inner Mitochondrial Membrane
- The proton gradient across the inner mitochondrial membrane is a driving force for ATP synthesis. The gradient, established by the ETC, is utilized by ATP synthase to produce ATP. Any changes in this proton gradient can significantly affect the rate of ATP synthesis. For instance, a steep gradient (high proton concentration difference across the membrane) typically enhances ATP production, while a diminished gradient slows it down.
- Feedback Inhibition
- Feedback inhibition is a regulatory mechanism where the end product of a process inhibits the process itself. In oxidative phosphorylation, high levels of ATP can inhibit certain enzymes in the ETC, thereby slowing down the electron transport and subsequent ATP production. This mechanism prevents the overaccumulation of ATP when it is not needed, conserving resources and energy.
- Control of Electron Carrier Availability
- The availability of electron carriers, such as NADH and FADH2, also plays a role in regulating oxidative phosphorylation. These carriers, which are reduced during earlier stages of cellular respiration, donate electrons to the ETC. Their availability is influenced by the cell’s metabolic state and can vary with different physiological conditions. An abundance of these carriers can stimulate oxidative phosphorylation, while a scarcity can limit it.
Transport Of Reducing Equivalents—shuttle Pathways
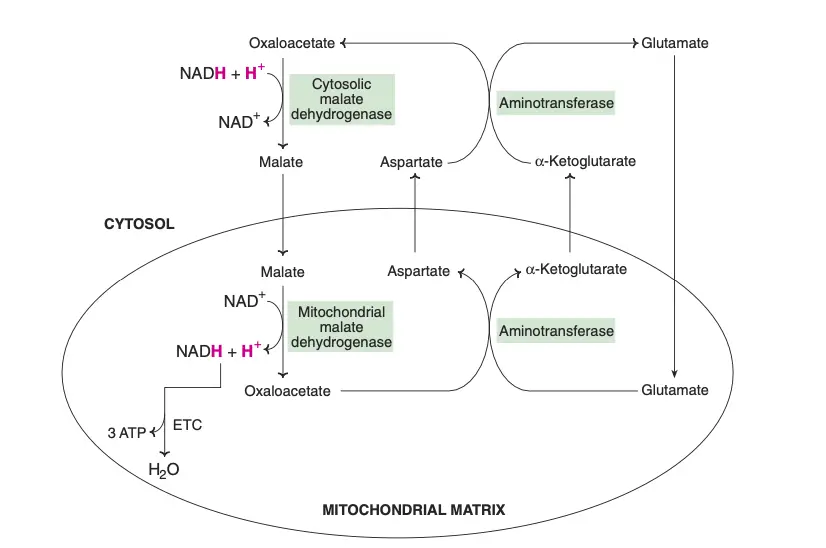
The transport of reducing equivalents from the cytosol to the mitochondria is a vital process in cellular metabolism. Due to the impermeability of the inner mitochondrial membrane to NADH, shuttle pathways are employed to transfer these reducing equivalents. Two primary shuttle systems facilitate this transfer: the glycerol-phosphate shuttle and the malate-aspartate shuttle.
- Glycerol-Phosphate Shuttle
- This shuttle involves the enzyme cytosolic glycerol 3-phosphate dehydrogenase, which oxidizes NADH to NAD+, transferring the reducing equivalents to glycerol 3-phosphate.
- Glycerol 3-phosphate dehydrogenase, located on the outer surface of the inner mitochondrial membrane, then reduces flavin adenine dinucleotide (FAD) to FADH2.
- Dihydroxyacetone phosphate, a byproduct of this reaction, moves back into the cytosol, allowing the shuttle to continue. In the electron transport chain (ETC), FADH2 is oxidized to generate 2 ATP molecules per cycle.
- Malate-Aspartate Shuttle
- In the cytosol, oxaloacetate accepts reducing equivalents from NADH and is converted into malate. Malate then enters the mitochondria, where it is oxidized by mitochondrial malate dehydrogenase, regenerating NADH and oxaloacetate.
- The mitochondrial NADH is then oxidized via the ETC, producing 3 ATP molecules per cycle, in contrast to the 2 ATP generated by the glycerol-phosphate shuttle.
- In the mitochondria, oxaloacetate undergoes a transamination reaction with glutamate to produce aspartate and α-ketoglutarate. Aspartate then returns to the cytosol and participates in a reverse transamination reaction to regenerate oxaloacetate and glutamate.
- Shuttle Pathways and Tissues
- Different tissues preferentially utilize these shuttles based on their specific metabolic requirements.
- The liver and heart predominantly use the malate-aspartate shuttle, yielding 3 ATP per mole of NADH oxidized.
- Most other tissues, however, rely on the glycerol-phosphate shuttle, resulting in the production of 2 ATP from each NADH.
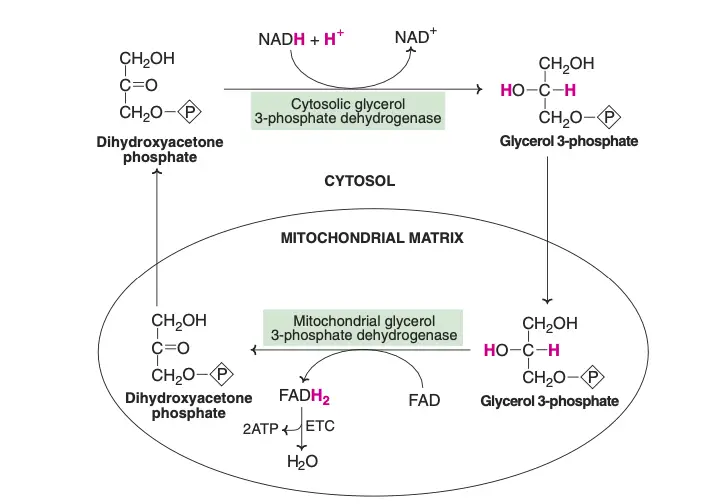
In summary, these shuttle pathways are essential for the transport of reducing equivalents into the mitochondria, a necessary step for efficient energy production in the cell. They illustrate the intricate regulatory mechanisms that cells employ to maximize energy production while adapting to varying metabolic demands.
Enzymes Involved In Biological Oxidation
Enzymes involved in biological oxidation play a crucial role in various metabolic processes. These enzymes, classified as oxidoreductases, are categorized into four main types: Oxidases, Dehydrogenases, Hydroperoxidases, and Oxygenases. Each category has distinct functions and characteristics:
- Oxidases
- Oxidases catalyze the removal of hydrogen from substrates, with oxygen typically acting as the hydrogen acceptor to form water. However, in some cases, hydrogen peroxide (H2O2) is formed instead.
- Examples include cytochrome oxidase, tyrosinase, and monoamine oxidase. Cytochrome oxidase, notably, is the terminal component of the electron transport chain, transferring electrons from oxidized substrates to oxygen.
- Some flavoproteins, containing flavin adenine dinucleotide (FAD) or flavin mononucleotide (FMN), also function as oxidases. Examples are L-amino acid oxidase (FMN) and xanthine oxidase (FAD).
- Dehydrogenases
- Dehydrogenases do not use oxygen as a hydrogen acceptor. They facilitate the reversible transfer of hydrogen from one substrate to another, thereby mediating oxidation-reduction reactions.
- This group includes a wide variety of enzymes:
- NAD+ dependent dehydrogenases, such as alcohol dehydrogenase and glycerol 3-phosphate dehydrogenase.
- NADP+ dependent dehydrogenases, like HMG CoA reductase and enoyl reductase.
- FMN and FAD dependent dehydrogenases, including NADH dehydrogenase and succinate dehydrogenase.
- Cytochromes in the electron transport chain, except for the terminal cytochrome oxidase, also fall into this category.
- Hydroperoxidases
- Hydroperoxidases use hydrogen peroxide as their substrate. They are crucial for mitigating the harmful effects of H2O2, which is constantly produced in reactions catalyzed by aerobic dehydrogenases.
- Key enzymes in this category are peroxidase and catalase, which convert hydrogen peroxide into water and oxygen (2H2O2 → 2H2O + O2).
- Oxygenases
- Oxygenases directly incorporate oxygen into substrate molecules and are divided into two subcategories:
- Dioxygenases (true oxygenases) incorporate both atoms of molecular oxygen (O2) into the substrate. Examples include homogentisate oxidase and L-tryptophan pyrrolase.
- Monooxygenases (mixed-function oxidases) incorporate one atom of oxygen into the substrate, while the other is reduced to water. These enzymes often utilize NADPH for reducing equivalents. The cytochrome P450 monooxygenase system is a notable example, involved in drug metabolism and steroid hormone biosynthesis.
- Oxygenases directly incorporate oxygen into substrate molecules and are divided into two subcategories:
Category | Enzyme Type | Examples/Functions |
---|---|---|
1. Oxidases | – Cytochrome oxidase | Terminal component of the electron transport chain, transfers electrons to oxygen. |
– Tyrosinase | Involved in the melanin synthesis. | |
– Monoamine oxidase | Catalyzes the oxidation of monoamines. Often forms H2O2 instead of H2O. | |
– Flavoprotein oxidases | Contain FAD or FMN, e.g., L-amino acid oxidase (FMN), xanthine oxidase (FAD). | |
2. Dehydrogenases | – NAD+ dependent dehydrogenases | E.g., Alcohol dehydrogenase, Glycerol 3-phosphate dehydrogenase. |
– NADP+ dependent dehydrogenases | E.g., HMG CoA reductase, Enoyl reductase. | |
– FMN dependent dehydrogenases | E.g., NADH dehydrogenase. | |
– FAD dependent dehydrogenases | E.g., Succinate dehydrogenase, Acyl CoA dehydrogenase. | |
– Cytochromes (except oxidase) | Cytochromes b, c1, and c in the electron transport chain. | |
3. Hydroperoxidases | – Peroxidase | Catalyzes reactions involving H2O2, converting it into water and oxygen. |
– Catalase | Breaks down hydrogen peroxide into water and oxygen. | |
4. Oxygenases | – Dioxygenases (True Oxygenases) | Incorporate both atoms of O2 into substrates, e.g., Homogentisate oxidase, L-tryptophan pyrrolase. |
– Monooxygenases (Mixed Function Oxidases) | Incorporate one atom of O2 into the substrate, e.g., Cytochrome P450 system for drug metabolism. |
Factors Affecting Oxidative Phosphorylation
Oxidative phosphorylation, a key biochemical process in cells, can be affected by various factors. These include inhibitors, uncoupling agents, physiological conditions, and environmental influences, each impacting the process in distinct ways:
- Inhibitors
- Respiratory chain inhibitors impede electron transport at specific points in the respiratory chain, affecting the oxidation process. Examples include rotenone, phenoxymycin A, and barbital, which inhibit electron transfer from NADH to CoQ.
- Other inhibitors, like antimycin A and dimercaptopropanol, prevent electron transport between cytochrome b (Cytb) and cytochrome c1 (Cytc1).
- Cytochrome oxidase, crucial for the final step of electron transport, can be inhibited by substances like cyanide, azide, hydrogen sulfide (H2S), and carbon monoxide (CO), disrupting the transfer of electrons to oxygen.
- Oxidative Phosphorylation Inhibitors
- These chemicals directly interfere with ATP synthesis by obstructing electron transport. Oligomycin and dicyclohexylcarbonyldiimide, for example, bind to the F0 unit of ATP synthase, impeding the return of hydrogen ions through the proton channel and leading to incomplete phosphorylation.
- Uncoupling Agents
- Uncoupling agents, such as 2,4-dinitrophenol (DNP), separate the processes of electron transfer and ATP synthesis. These agents do not inhibit electron transfer but prevent ATP formation, leading to the conversion of the free energy from electron transfer into heat, causing excessive oxygen and fuel substrate usage.
- ADP Availability
- In normal organisms, the rate of oxidative phosphorylation is largely governed by the availability of ADP. When more ATP is utilized, and ADP is transported into the mitochondria, oxidative phosphorylation rate increases. Conversely, a scarcity of ADP slows down the process, allowing for regulation in response to physiological demands.
- Thyroid Hormone
- Thyroid hormone can activate Na+-K+ATPase on cell membranes, accelerating the breakdown of ATP into ADP and inorganic phosphate (Pi), and thereby increasing ADP levels in mitochondria. This enhances oxidative phosphorylation, raising the basal metabolic rate, a significant clinical indicator in hyperthyroidism.
- Mitochondrial DNA Mutation
- Mitochondrial DNA (mtDNA) mutations, due to its lack of protein protection and damage repair mechanism, can impact oxidative phosphorylation. Since mtDNA encodes 13 proteins related to this pathway, mutations can lead to reduced ATP production and various diseases.
- Environmental Factors
- Environmental factors, such as exposure to toxins, pollutants, or specific drugs, can disrupt the electron transport chain, affecting ATP synthesis. Nutritional changes, like fasting or a high-fat diet, can also influence the efficiency of oxidative phosphorylation.
Inhibitors Of Oxidative Phosphorylation
The process of oxidative phosphorylation can be inhibited or altered by various compounds, which are categorized as uncouplers, ionophores, and other specific inhibitors. Each of these plays a distinct role in affecting the efficiency and mechanism of oxidative phosphorylation.
- Uncouplers
- Uncouplers separate or uncouple the electron transport from oxidative phosphorylation. This results in the oxidation of substrates like NADH or FADH2 without the concomitant formation of ATP. The energy generated is dissipated as heat instead.
- 2,4-Dinitrophenol (DNP) is a well-studied uncoupler. It is a small lipophilic molecule that acts as a proton carrier and diffuses through the inner mitochondrial membrane. Although previously used for weight loss, its use has been discontinued due to severe side effects, including hyperthermia.
- Other uncouplers include dinitrocresol, pentachlorophenol, and trifluorocarbonylcyanide phenylhydrazone (FCCP), with FCCP being notably more potent than dinitrophenol. High doses of aspirin can also act as an uncoupler.
- Physiological Uncouplers
- Certain physiological substances, such as thermogenin, thyroxine, and long-chain free fatty acids, can act as uncouplers at higher concentrations. Unconjugated bilirubin is also thought to be an uncoupler, although this is not conclusively proven.
- Brown adipose tissue, particularly in hairless and hibernating animals and those adapted to cold environments, exhibits a form of non-shivering thermogenesis. It contains mitochondria rich in electron carriers that oxidize fat to generate heat rather than ATP.
- Ionophores
- Ionophores are lipophilic substances that facilitate the transport of ions across biological membranes.
- While all uncouplers are proton ionophores, certain antibiotics like valinomycin, gramicidin A, and nigercin act as ionophores for potassium ions (K+). These compounds can also dissipate the proton gradient across the inner mitochondrial membrane, inhibiting oxidative phosphorylation.
- Other Inhibitors of Oxidative Phosphorylation
- Oligomycin is an antibiotic that inhibits both mitochondrial oxidation and phosphorylation. It binds to ATP synthase and blocks proton channels, preventing proton re-entry into the mitochondrial matrix and ultimately halting electron transport.
- Atractyloside, a plant toxin, inhibits oxidative phosphorylation indirectly by blocking the adenine nucleotide carrier system, which is responsible for transporting ATP and ADP. This blockade prevents the adequate supply of ADP necessary for phosphorylation.
Importance of Oxidative Phosphorylation
Oxidative phosphorylation (OxPhos) is a fundamental biological process that plays a pivotal role in cellular energy metabolism. Its significance can be understood through the following points:
- Primary ATP Production: Oxidative phosphorylation is the primary source of ATP in aerobic organisms. ATP, often termed the “energy currency” of the cell, powers a vast array of cellular processes, from muscle contraction to signal transduction.
- Efficiency in Energy Extraction: Compared to other metabolic pathways like glycolysis, OxPhos is highly efficient. A single glucose molecule can yield up to 32 ATP molecules through oxidative phosphorylation, whereas glycolysis alone produces only 2 ATP molecules.
- Regulation of Metabolism: The process provides a feedback mechanism for cellular metabolism. High levels of ATP inhibit the process, ensuring that energy is produced only when needed, preventing wasteful overproduction.
- Maintenance of Electrochemical Gradients: The pumping of protons across the inner mitochondrial membrane during OxPhos establishes an electrochemical gradient. This gradient, known as the proton motive force, is essential not only for ATP synthesis but also for other cellular processes like the transport of certain substrates into the mitochondria.
- Redox Balance: Oxidative phosphorylation helps maintain the redox balance in the cell. Electron carriers like NADH and FADH2 are reoxidized to NAD+ and FAD, respectively, ensuring the continuation of other metabolic pathways like the citric acid cycle.
- Heat Production: In certain organisms, especially in mammals, the inefficiencies in the process are utilized for thermogenesis. The “leakage” of protons across the mitochondrial membrane without ATP production generates heat, which is crucial for maintaining body temperature.
- Role in Apoptosis: Components of the electron transport chain, especially cytochrome c, play roles in the intrinsic pathway of apoptosis, a regulated form of cell death. This highlights the importance of OxPhos in cell fate decisions.
- Interplay with Other Pathways: The intermediates and products of oxidative phosphorylation, such as ATP, NADH, and reactive oxygen species (ROS), interact with various other cellular pathways, influencing cell signaling, gene expression, and metabolic regulation.
- Clinical Relevance: Defects in oxidative phosphorylation are linked to a range of human diseases, including mitochondrial disorders, neurodegenerative diseases, and certain types of cancer. Understanding OxPhos is crucial for diagnosing, managing, and potentially treating these conditions.
Quiz
References
- Bender, D.A. (2003). Encyclopedia of Food Sciences and Nutrition || OXIDATIVE PHOSPHORYLATION. , (), 4295–4301. doi:10.1016/b0-12-227055-x/01407-3
- Cardol, Pierre (2009). The Chlamydomonas Sourcebook || Oxidative Phosphorylation. , (), 469–502. doi:10.1016/b978-0-12-370873-1.00021-6
- Engelking, Larry R. (2015). Textbook of Veterinary Physiological Chemistry || Oxidative Phosphorylation. , (), 219–224. doi:10.1016/b978-0-12-391909-0.50036-0
- Oxidative Phosphorylation – Definition, Oxidative Phosphorylation Steps. BYJUS. (2022). Retrieved 30 May 2022, from https://byjus.com/biology/oxidative-phosphorylation/.
- Nelson, D., & Cox, M. (2005). Lecture notebook for Lehninger Principles of biochemistry, fourth edition (4th ed., pp. 690-719). Freeman.
- Cooper GM. The Cell: A Molecular Approach. 2nd edition. Sunderland (MA): Sinauer Associates; 2000. The Mechanism of Oxidative Phosphorylation. Available from: https://www.ncbi.nlm.nih.gov/books/NBK9885/
- Deshpande OA, Mohiuddin SS. Biochemistry, Oxidative Phosphorylation. [Updated 2023 Jul 31]. In: StatPearls [Internet]. Treasure Island (FL): StatPearls Publishing; 2023 Jan-. Available from: https://www.ncbi.nlm.nih.gov/books/NBK553192/