HSP | heavy strand promoter |
LSP | light strand promoter |
MGME1 | mitochondrial genome maintenance exonuclease 1 |
mtSSB | mitochondrial single-stranded DNA-binding protein |
NCR | noncoding region |
TAS | termination-associated sequence |
What is Mitochondrial DNA (mtDNA)?
- Mitochondrial DNA (mtDNA) is the genetic material found in mitochondria, the energy-producing organelles within eukaryotic cells. Unlike nuclear DNA, which is linear and organized into chromosomes, mtDNA is typically a circular, covalently closed duplex. It is much smaller than nuclear DNA, consisting of about 16,569 base pairs in humans, and is present in multiple copies within each mitochondrion, allowing for a high copy number in somatic cells.
- Mitochondrial DNA encodes essential genes required for mitochondrial function, including those involved in the oxidative phosphorylation pathway, which is crucial for ATP production. Additionally, mtDNA is inherited maternally, meaning it is passed down from mothers to their offspring, while paternal mtDNA is usually degraded shortly after fertilization. This unique inheritance pattern and the distinct properties of mtDNA make it an important area of study in genetics, evolution, and various diseases.
Structure of Mitochondrial DNA (mtDNA)
- Shape:
- Circular: mtDNA is typically a circular molecule, which is distinct from the linear structure of nuclear DNA.
- Size:
- Length: In humans, mtDNA consists of approximately 16,569 base pairs, making it much smaller than the nuclear genome.
- Strands:
- Heavy (H) Strand:
- Rich in guanine (G) bases.
- Contains the majority of the genes.
- Light (L) Strand:
- Rich in cytosine (C) bases.
- Contains fewer genes compared to the H strand.
- Heavy (H) Strand:
- Nucleoprotein Complexes:
- Mitochondrial Nucleoids: mtDNA is organized into structures called nucleoids, which are complexes of mtDNA and proteins.
- Copy Number: Each nucleoid typically contains an average of 1.4 copies of mtDNA.
- Packaging:
- Non-histone Proteins: Unlike nuclear DNA, mtDNA is not packaged with histones. Instead, it is associated with mitochondrial transcription factor A (TFAM) and other proteins that help maintain its structure and function.
- D-loop Region:
- Displacement Loop (D-loop): A significant region of mtDNA that contains a three-stranded structure. This region is crucial for the regulation of mtDNA replication and transcription.
- Gene Content:
- Total Genes: mtDNA encodes 37 genes:
- 2 Ribosomal RNAs (rRNAs): Essential for mitochondrial protein synthesis.
- 22 Transfer RNAs (tRNAs): Required for the translation of mitochondrial mRNA.
- 13 Proteins: Involved in the oxidative phosphorylation pathway, which is critical for ATP production.
- Total Genes: mtDNA encodes 37 genes:
- Lack of Introns:
- Compact Structure: Mitochondrial genes are densely packed without introns, allowing for efficient transcription and translation.
- Replication:
- Independent Replication: mtDNA replicates independently of the cell cycle, allowing for rapid adaptation to the energy needs of the cell.
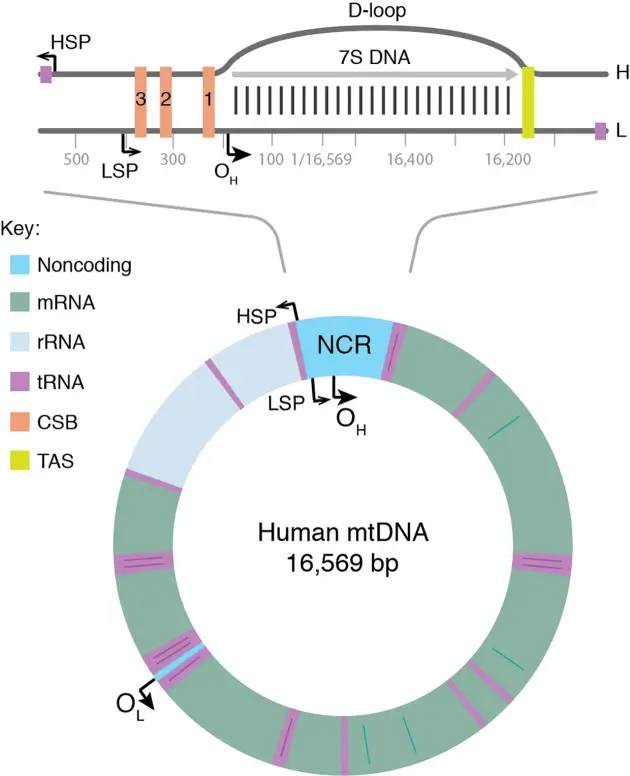
General Features of the Mitochondrial Genome
- Origin:
- Mitochondria are believed to have originated from a single endosymbiotic event between an ancestral eukaryotic cell and an aerobic α-proteobacterium approximately 2 billion years ago.
- Structure:
- Circular DNA: The mitochondrial genome is typically circular and covalently closed.
- Size: In humans, the mitochondrial genome is about 16,569 base pairs long, significantly smaller than the nuclear genome.
- Strands:
- Heavy (H) Strand: Contains a higher guanine content and encodes most of the mitochondrial genes.
- Light (L) Strand: Contains a higher cytosine content and encodes fewer genes.
- Gene Content:
- Total Genes: The human mitochondrial genome encodes 37 genes:
- 2 Ribosomal RNAs (rRNAs): Essential for mitochondrial protein synthesis.
- 22 Transfer RNAs (tRNAs): Required for the translation of mitochondrial mRNA.
- 13 Protein-Coding Genes: Involved in the oxidative phosphorylation pathway, crucial for ATP production.
- Total Genes: The human mitochondrial genome encodes 37 genes:
- Replication:
- Independent Replication: mtDNA replicates independently of the cell cycle, allowing for a high copy number in cells.
- Inheritance:
- Maternal Inheritance: Mitochondrial DNA is inherited exclusively from the mother, as paternal mtDNA is typically degraded after fertilization.
- Lack of Introns:
- Mitochondrial genes are densely packed without introns, facilitating efficient transcription and translation.
- Nucleoprotein Complexes:
- Mitochondrial Nucleoids: mtDNA is organized into structures called nucleoids, which consist of mtDNA and associated proteins, including mitochondrial transcription factor A (TFAM).
- D-loop Region:
- Control Region: The D-loop region serves as a major control site for mtDNA replication and transcription, containing regulatory elements.
- Functional Role:
- The mitochondrial genome is essential for energy production, cellular metabolism, and various biochemical pathways, playing a critical role in the overall function of the cell.
Factors for mtDNA replication
Mitochondrial DNA (mtDNA) replication is a critical process distinct from nuclear DNA replication, relying on specific proteins that often have similarities with those identified in bacteriophages. Understanding these factors is essential for grasping how mtDNA is accurately replicated and maintained within mammalian cells.
Key Factors Involved in mtDNA Replication
- DNA Polymerase γ (POLγ):
- Composition: POLγ is the primary replicative polymerase in mitochondria. It is a heterotrimer composed of one catalytic subunit (POLγA) and two accessory subunits (POLγB).
- Function: POLγA, a member of the family A DNA polymerases, contains a 3′–5′ exonuclease domain, which provides proofreading capabilities, ensuring high fidelity with a misincorporation rate lower than 1 × 10−6.
- Role of POLγB: The accessory subunits POLγB enhance the interaction with the DNA template, increasing the catalytic activity and processivity of POLγA. These subunits are vital for efficient and accurate DNA synthesis.
- TWINKLE DNA Helicase:
- Mechanism: TWINKLE is a DNA helicase homologous to the T7 phage gene 4 protein. It unwinds the double-stranded DNA template ahead of POLγ, facilitating replication.
- Structure and Loading: TWINKLE forms a hexamer and requires a specific fork structure with a single-stranded 5′-DNA loading site and a short 3′-tail to initiate unwinding. This configuration allows TWINKLE to efficiently separate the DNA strands, providing a single-stranded template for replication.
- Mitochondrial Single-Stranded DNA-Binding Protein (mtSSB):
- Protection and Stabilization: mtSSB binds to the single-stranded DNA (ssDNA) formed during replication, protecting it from nucleases and preventing the formation of secondary structures that could hinder the replication process.
- Enhancement of Replication: By binding to ssDNA, mtSSB stimulates the helicase activity of TWINKLE and increases the processivity of POLγ, thereby enhancing the overall efficiency and accuracy of mtDNA replication.
- Additional Polymerases:
- Roles and Functions: Other polymerases such as PrimPol, DNA polymerase β, DNA polymerase θ, and DNA polymerase ζ have been implicated in mitochondrial functions. While these are not essential for mtDNA maintenance and cannot replace POLγ, they likely play roles in mtDNA repair. Their precise functions in mtDNA maintenance require further elucidation.
Detailed and Sequential Explanation
- Initiation:
- Role of POLγ: The replication process begins with POLγ, where POLγA performs the catalytic activity and POLγB enhances processivity and interaction with the DNA template.
- Proofreading: The 3′–5′ exonuclease activity of POLγA ensures that any misincorporated nucleotides are corrected, maintaining replication fidelity.
- Unwinding:
- Function of TWINKLE: TWINKLE helicase unwinds the double-stranded DNA, creating a single-stranded template necessary for replication.
- Hexamer Formation: TWINKLE forms a hexamer and binds to the replication fork, efficiently unwinding the DNA ahead of the polymerase.
- Single-Strand Stabilization:
- mtSSB Binding: mtSSB binds to the newly formed ssDNA, protecting it and preventing the formation of secondary structures that could impede replication.
- Processivity Enhancement: The binding of mtSSB also enhances the activity of both TWINKLE and POLγ, ensuring a smooth and efficient replication process.
- Elongation:
- Synthesis by POLγ: With the template strands stabilized and unwound, POLγ synthesizes the new DNA strand with high accuracy and efficiency, guided by the proofreading activity of its exonuclease domain.
Steps of Mitochondrial DNA Replication (mtDNA)
- Initiation:
- Origin of Replication: Replication begins at the D-loop region, specifically at the origin of heavy strand replication (O_H). This region is crucial for the binding of the replication machinery.
- Binding of Proteins: Mitochondrial transcription factor A (TFAM) and other proteins bind to the D-loop, facilitating the assembly of the replication complex.
- RNA Primer Synthesis:
- RNA Primers: The mitochondrial transcription apparatus synthesizes short RNA primers necessary for the initiation of DNA synthesis. This process involves mitochondrial RNA polymerase (POLRMT) and other transcription factors.
- Strand Displacement:
- Leading Strand Synthesis: The synthesis of the daughter heavy (H) strand begins, displacing the parental H strand. This results in the formation of a three-stranded structure in the D-loop region.
- Lagging Strand Initiation: The origin of light strand replication (O_L) is exposed as the replication fork progresses, allowing for the initiation of the lagging (L) strand synthesis.
- Synthesis of Daughter Strands:
- DNA Polymerase Activity: DNA polymerase gamma (POLG) synthesizes the new H strand continuously, while the L strand is synthesized in short segments (Okazaki fragments) due to its lagging nature.
- Helicase Activity: The helicase Twinkle unwinds the double-stranded DNA, allowing for the replication fork to advance.
- Processing of RNA Primers:
- Removal of RNA Primers: Once the daughter strands are synthesized, the RNA primers are removed by RNase H1, and the resulting gaps are filled in by mitochondrial genome maintenance exonuclease 1 (MGME1).
- Ligation:
- Sealing the Gaps: DNA ligase III (LIG3) seals the nicks in the newly synthesized strands, completing the formation of the daughter DNA molecules.
- Decatenation:
- Topological Separation: The newly formed daughter mtDNA molecules are initially linked as hemicatenanes. Mitochondrial topoisomerase 3α (TOP3A) catalyzes the decatenation process, allowing the daughter molecules to separate.
- Finalization:
- Tertiary Structure Formation: The daughter mtDNA molecules adopt their tertiary structure, which is essential for their stability and function within the mitochondria.
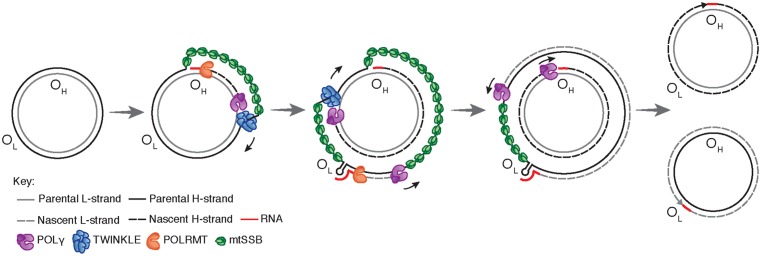
Replication of the human mitochondrial genome
- Mitochondrial DNA replication starts at OH and goes in one direction until the nascent H-strand is full length.
- mtSSB binds to the exposed parental H-strand and keeps it safe.
- When the replisome passes OL, a stem-loop structure is made that prevents mtSSB from binding. This leaves a single-stranded loop-region where POLRMT can start making primers.
- After about 25 nt, when POLγ replaces POLRMT at the 3′ end of the primer, the process changes to making L-strand DNA.
- The two strands are made in a continuous process until there are two full, double-stranded DNA molecules.
What is the D-loop?
The D-loop, or displacement loop, is a unique triple-stranded DNA structure found within mitochondrial DNA (mtDNA). It forms during a specific phase of mtDNA replication and is a crucial, albeit not fully understood, component of mitochondrial genetics.
Formation of the D-loop
- Initiation at OH:
- mtDNA replication typically initiates at the origin of heavy strand replication (OH). However, not all replication events proceed to completion. About 95% of these events terminate prematurely at termination-associated sequences (TAS) after synthesizing approximately 650 nucleotides.
- Creation of 7S DNA:
- This premature termination produces a short DNA fragment known as 7S DNA. The 7S DNA binds to the parental light strand (L-strand), while the parental heavy strand (H-strand) is displaced.
- Formation of the Triple-Stranded Structure:
- The binding of 7S DNA to the L-strand and the displacement of the H-strand result in the formation of a triple-stranded structure known as the D-loop.
Functional Significance and Regulation
- Unclear Functional Importance:
- The exact functional role of the D-loop remains unclear. Despite its presence in many mitochondrial genomes, its precise purpose in the replication process is not fully understood.
- Regulation of Replication:
- Termination at TAS appears to be a regulated event. This regulation may serve as a switch between abortive replication and full-length genome replication.
- Role of POLγ and TWINKLE:
- Under normal conditions, DNA polymerase γ (POLγ) stalls at the 3′-end of the D-loop, whereas the occupancy of the TWINKLE helicase is relatively low in this region. When mtDNA levels are depleted, TWINKLE occupancy increases, and 7S DNA levels decrease. This suggests that TWINKLE may reload in response to increased demands for mtDNA replication.
- Mouse Genetic Experiments:
- Studies in mice indicate that TWINKLE plays a significant role in controlling mtDNA copy number. Variations in TWINKLE levels correlate with changes in mtDNA levels, suggesting that mtDNA replication is regulated more at the level of pretermination than initiation.
Sequence Motifs and Binding Proteins
- Palindromic Sequence Motifs:
- The D-loop region contains two evolutionarily conserved palindromic sequence motifs (ATGN9CAT) located on each side of the D-loop. One is situated upstream of the 5′-end of 7S DNA, forming part of the conserved sequence box 1 (CSB1). The other, core-TAS, is located within the TAS region, downstream of the 3′-end of 7S DNA.
- Potential Binding Sites:
- These motifs might serve as binding sites for specific DNA-binding proteins. Despite extensive research, a TAS-binding protein has not yet been identified. It is hypothesized that these proteins may be membrane-bound or require specific conditions for binding, making them challenging to isolate.
- Secondary Structures:
- It is also possible that secondary structures within mtDNA, such as stem-loops or G-quadruplexes, play a role in the regulation and formation of the D-loop. These structures might contribute to the observed DNA footprints in vivo.
Initiation of Mitochondrial DNA Replication (mtDNA) at OH
The initiation of mitochondrial DNA (mtDNA) replication at the origin of heavy strand replication (OH) involves a series of coordinated steps facilitated by various factors and molecular processes. This process is crucial for the accurate replication and maintenance of the mitochondrial genome.
Key Components and Steps
- Formation of RNA Primers:
- POLRMT Function: DNA replication at OH begins with the formation of primers by mitochondrial RNA polymerase (POLRMT). POLRMT synthesizes RNA primers that provide the necessary 3′-ends for DNA polymerase γ (POLγ) to initiate DNA synthesis on the heavy strand.
- RNA-to-DNA Transition Points:
- Transcription Initiation: In human mitochondria, transcription from the light strand promoter (LSP) produces RNA transcripts with 3′-ends that are critical for POLγ to start DNA replication.
- Conserved Sequence Motifs: Downstream of LSP, several transition points where RNA converts to DNA are clustered around two conserved sequence motifs, CSB3 and CSB2. These motifs are guanine-rich and play a significant role in the replication initiation process.
- Formation of R-loop Structure:
- G-Quadruplex and R-loop: During transcription, the guanine-rich CSB2 region can form a G-quadruplex structure between the nascent RNA and the non-template DNA strand. This interaction anchors the nascent RNA to the mtDNA, forming an R-loop structure.
- Premature Transcription Termination: The G-quadruplex structure can induce premature transcription termination at sites corresponding to the RNA-to-DNA transition sites in the CSB2 region.
- Role of Transcription Elongation Factor TEFM:
- Influence on Transcription Termination: The transcription elongation factor TEFM reduces transcription termination and R-loop formation at CSB2. It is suggested that TEFM may influence the balance between primer formation and full-length transcription.
- Limited Effects of TEFM Knockdown: Experiments show that knocking down TEFM has minimal effects on mtDNA copy number and mitochondrial replication intermediates. This suggests that TEFM’s role in replication initiation may not be as pivotal as initially thought.
- R-loop Formation and Primer Use:
- Hypothesis on Primer Formation: It has been hypothesized that sequence-dependent transcription termination might contribute to primer formation at OH. However, direct experimental evidence showing that R-loops are used by POLγ to initiate DNA synthesis is lacking. Further research is required to clarify the precise role of R-loops and TEFM in replication initiation.
- Comparisons with Bacterial Plasmids:
- E. coli Plasmid ColE1: The initiation mechanisms at OH may be similar to those observed in the E. coli plasmid ColE1. In ColE1, a transcript known as RNAII forms an R-loop with the template strand, which is then used to prime DNA synthesis.
- RNase H Role: In ColE1, RNase H cleaves the R-loop before DNA synthesis can proceed. Whether mitochondrial RNase H1 performs a similar role in mammalian cells remains an area for further investigation.
Termination of Mitochondrial DNA Replication (mtDNA)
Termination of mitochondrial DNA (mtDNA) replication is a critical step in ensuring the accurate completion of the mitochondrial genome replication cycle. This process involves multiple coordinated activities to finalize DNA synthesis, remove residual RNA primers, and complete the circular mtDNA molecule.
Key Processes and Mechanisms
- Ligation of Newly Synthesized DNA:
- Role of DNA Ligase III: Once POLγ has completed the synthesis of the nascent DNA strands, DNA ligase III facilitates the ligation of these newly formed strands. Effective ligation requires that the 5′- and 3′-ends of the nascent DNA are juxtaposed.
- Primer Removal: To enable efficient ligation, the RNA primers used during the initiation of mtDNA synthesis must be removed. This is essential to ensure that no RNA remains at the ligation junctions.
- RNA Primer Removal:
- Function of RNASEH1: RNASEH1 is a key enzyme responsible for the removal of RNA primers. In mouse embryonic fibroblasts lacking RNASEH1, RNA primers are retained in mitochondrial origin regions, leading to mtDNA loss in RNASEH1 knockout mice. Therefore, RNASEH1 plays a crucial role in the removal of RNA primers and the proper completion of mtDNA replication.
- Completion of Circular DNA Replication:
- Encounter with 5′-End: After synthesizing a full-length mtDNA strand, POLγ encounters the 5′-end of the nascent DNA. At this juncture, POLγ initiates successive cycles of polymerization and 3′–5′ exonuclease degradation at the nick to prepare the ends for ligation.
- Importance of Idling: The process of idling is necessary for proper ligation. POLγ with exonuclease activity can correct the nicks by degrading excess DNA, whereas POLγ lacking this activity continues synthesis into the double-stranded DNA region, creating a flap-structure that cannot be ligated.
- Formation of Flap-Structures:
- Impact of Exonuclease-Deficient POLγ: POLγ lacking exonuclease activity generates a flap-structure, which cannot be ligated. This failure to create ligatable DNA ends may explain why exonuclease-deficient POLγ mutants exhibit strand-specific nicks at the origin of heavy strand replication (OH).
- Generation of 5′-End of Nascent DNA:
- Position of 5′-End: A major 5′-end of the nascent DNA, located approximately 100 base pairs downstream of the RNA-to-DNA transition sites, has been identified. Historically, this site has been considered part of OH, but its exact generation mechanism is not well understood.
- Potential Processing: The 5′-end at position 191 might be generated through substantial processing during primer removal, potentially removing ∼100 nucleotides of downstream DNA. This processing could be facilitated by mitochondrial genome maintenance exonuclease 1 (MGME1).
- Role of MGME1:
- Function and Analysis: MGME1 is a mitochondrial RecB-type exonuclease from the PD-(D/E)XK nuclease superfamily. It has been shown to cut both single-stranded DNA and DNA flap substrates in vitro. Human cells lacking active MGME1 exhibit impaired ligation at OH and formation of linear mtDNA molecules with deletions spanning OH and OL.
- Impact on 7S DNA: Elevated levels of 7S DNA, with 5′-ends located closer to CSB2 than in normal cells, suggest that MGME1 is involved in processing the 5′-end of the nascent heavy strand. Further research on MGME1 and its interaction with RNASEH1 will enhance the understanding of mtDNA replication termination.
Mitochondrial DNA (mtDNA) Separation
Mitochondrial DNA (mtDNA) separation is a crucial aspect of mitochondrial DNA replication. This process ensures that newly synthesized mtDNA molecules are fully separated into distinct, functional entities, enabling proper mitochondrial function and maintenance. Here is a detailed exploration of the mechanisms involved in mtDNA separation:
Key Mechanisms and Processes
- Topological Challenges During Replication:
- Steric Hindrance: During mtDNA replication, the intact parental molecule presents a steric hindrance to the moving replication machinery. This challenge arises because the replication process generates torsional strain as the replication fork progresses through the circular DNA.
- Role of Topoisomerases: Topoisomerases are essential for alleviating this torsional strain. Topoisomerases of the type 1 family, such as TOP1MT, function to relieve this strain by allowing one strand of DNA to pass through another, effectively acting as a DNA “swivel” within the mitochondrial replisome.
- Topoisomerase 1MT (TOP1MT):
- Function and Importance: TOP1MT, a type IB enzyme, is specifically involved in mitochondrial DNA replication. It works in conjunction with the mitochondrial replisome to manage the topological issues that arise during DNA replication.
- Impact of Gene Knockout: Mice with a knockout of the Top1mt gene show altered mtDNA supercoiling, indicating the critical role of TOP1MT in maintaining proper mtDNA topology and function.
- Formation of Catenanes:
- Definition and Formation: During the replication of circular DNA, including mtDNA, daughter molecules can become mechanically interlocked, forming structures known as catenanes. These are linked but not yet fully separated DNA circles.
- Historical Context: The presence of catenanes in mitochondria was first reported by Vinograd and colleagues in 1967. They identified X-type branches linking mtDNA molecules, which were hypothesized to form during the completion of mtDNA replication.
- Resolution of Hemicatenanes:
- Identification and Function: Recent studies have shown that these X-type structures are hemicatenanes, which are double-stranded DNA molecules linked together via single-stranded linkages.
- Role of Topoisomerase 3α (Top3α): A mitochondrial isoform of Topoisomerase 3α (Top3α) is essential for resolving these hemicatenanes. Without Top3α, mtDNA accumulates in large, catenated networks, leading to decreased mtDNA levels and associated mitochondrial dysfunctions.
- Clinical Relevance: Mutations in Top3α that reduce its activity cause symptoms similar to those seen in other mitochondrial replication disorders, such as muscle-restricted mtDNA deletions and chronic progressive external ophthalmoplegia.
- Additional Proteins Involved in mtDNA Separation:
- Top3α and Associated Proteins: While Top3α is critical for resolving hemicatenanes, additional proteins likely participate in this process. In the nucleus, Top3α functions with the helicase BLM and OB-fold proteins RMI1 and RMI2 in the BTR complex, which resolves double Holliday junctions.
- Mitochondrial Partners: Since mitochondrial isoforms of BLM, RMI1, or RMI2 are not present, other mitochondrial-specific proteins may interact with Top3α to regulate and enhance its activity in mitochondria.
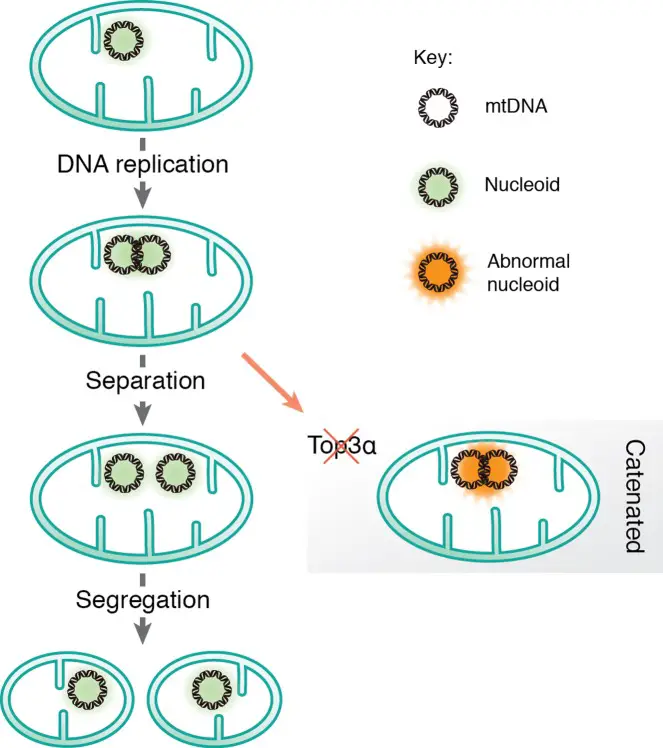
Replication of Nucleoid
The replication of mitochondrial DNA (mtDNA) involves the dynamic organization and function of nucleoid complexes within mitochondria. These nucleoids are integral to the proper maintenance and replication of mtDNA. The following outlines the key components and processes involved in nucleoid replication:
1. Nucleoid Structure and Composition
- Definition and Visualization:
- Nucleoids are not simple DNA molecules but are instead large nucleoprotein complexes. These structures, visualized using fluorescent microscopy, are approximately 100 nm in diameter.
- Each nucleoid typically contains a single mtDNA molecule.
- Major Structural Protein:
- TFAM (Mitochondrial Transcription Factor A): TFAM is the primary structural protein of the nucleoid. It binds mtDNA with a ratio of one TFAM subunit per 16–17 base pairs of mtDNA.
- Characteristics: TFAM is a member of the high mobility group (HMG) box domain family and binds DNA non-specifically, allowing for versatile interactions with mtDNA.
2. Role of TFAM in mtDNA Packaging
- DNA Compaction:
- TFAM plays a crucial role in compacting mtDNA by facilitating cross-strand binding and loop formation. This protein can reconstitute nucleoid-like particles by simply mixing TFAM with mtDNA, demonstrating its ability to fully compact mtDNA independently.
- TFAM binds cooperatively, forming protein patches along mtDNA, contributing to its structural organization.
- Transcription Initiation:
- During mitochondrial transcription initiation, TFAM binds upstream of the transcription start site, inducing a sharp bend in the DNA. This bending is essential for the proper assembly of the transcription machinery.
3. Epigenetic Regulation by TFAM
- Impact on mtDNA Replication and Transcription:
- TFAM is suggested to act as an epigenetic regulator of mtDNA replication. Its varying forms and concentrations can significantly influence mtDNA compaction and availability for replication and transcription.
- Super-resolution microscopy indicates different forms of nucleoids, with more compact structures potentially representing a storage form and larger forms involved in active replication and transcription.
- Coordination with Cellular Structures:
- Nucleoids involved in active replication are often localized at contact points between the endoplasmic reticulum (ER) and mitochondria. These contact sites are crucial for mitochondrial division, suggesting that ER-mitochondria interactions coordinate mtDNA synthesis with mitochondrial division to ensure proper distribution.
4. In Vitro Studies and Effects of TFAM Concentrations
- TFAM-DNA Ratio:
- In vitro studies reveal that variations in the TFAM-to-DNA ratio can lead to significant changes in mtDNA compaction. At physiological TFAM levels, both fully compacted nucleoids and naked DNA can be observed.
- A slight increase in TFAM concentration can markedly increase the number of fully compacted mtDNA molecules, indicating a high sensitivity of mtDNA compaction to TFAM levels.
- Regulation of mtDNA Availability:
- The proportion of TFAM to mtDNA in vivo is relatively stable, which may explain the proportional relationship between TFAM levels and mtDNA levels. TFAM levels, therefore, likely regulate the number of mtDNA molecules available for active processes.
- Increased TFAM patches can prevent DNA unwinding, impeding the progress of mtDNA replication and transcription. This suggests that TFAM functions as an epigenetic regulator, modulating mtDNA replication and gene expression.
Methods of Transmission of the Mitochondrial Genome
- Maternal Inheritance:
- The primary method of mitochondrial genome transmission is maternal inheritance. In mammals, the entire mitochondrial DNA (mtDNA) is passed from mother to offspring through the oocyte. The sperm’s mitochondria are typically eliminated after fertilization, ensuring that only maternal mtDNA is transmitted.
- Oocyte Contribution:
- Oocytes contain a significantly higher number of mtDNA copies compared to sperm. This high copy number ensures that maternal mtDNA predominates in the zygote, effectively diluting any paternal mtDNA that may enter during fertilization.
- Mechanisms Preventing Paternal mtDNA Transmission:
- Several mechanisms actively prevent the transmission of paternal mtDNA:
- Degradation of Paternal mtDNA: After fertilization, paternal mtDNA is often degraded by cellular mechanisms, including the action of mitochondrial endonuclease G, which plays a key role in this degradation process.
- Dilution Effect: The large number of maternal mtDNA molecules in the oocyte further dilutes any paternal mtDNA, making it unlikely to be detected in the offspring.
- Several mechanisms actively prevent the transmission of paternal mtDNA:
- Mitochondrial Bottleneck:
- During oogenesis, a bottleneck occurs where only a small number of mtDNA molecules are selected for amplification and transmission to the next generation. This can lead to variability in mtDNA composition among siblings, even though they inherit mtDNA exclusively from their mother.
- Clonal Transmission:
- Once mtDNA is transmitted to the offspring, it is clonally inherited. This means that all cells in the offspring will carry the same mtDNA, which is passed down through subsequent generations via maternal lines.
- Potential for Paternal Contribution:
- Although the strict maternal inheritance of mtDNA is the norm, there have been rare reports of paternal mtDNA contributions in some families. However, these cases are considered exceptions and do not affect the general understanding of mtDNA transmission.
- Implications for Genetic Counseling:
- Understanding the methods of mitochondrial genome transmission is crucial for genetic counseling, especially in cases of mitochondrial diseases, which are inherited in a maternal manner. This knowledge helps in assessing recurrence risks and informing family planning decisions.
What are the primary methods of transmission for the mitochondrial genome?
The primary method of transmission for the mitochondrial genome is maternal inheritance. In nearly all mammals, the entire spermatozoon, including its mitochondria, is present at fertilization; however, only the maternal mitochondrial DNA (mtDNA) is passed on to the offspring. This is due to several mechanisms that actively prevent the transmission of paternal mtDNA, including:
- Dilution: The mtDNA copy number in an oocyte is significantly higher than that in a spermatozoon, leading to a dilution effect where paternal mtDNA is not detected in the offspring.
- Degradation of Paternal mtDNA: Shortly before or after fertilization, paternal mtDNA is actively degraded, a process in which mitochondrial endonuclease G plays a key role.
- Genetic Bottleneck: During female germline development, a small number of mtDNA molecules are sampled from a larger population for amplification and transmission, leading to variability in mtDNA mutation load among offspring.
These mechanisms ensure that mtDNA is inherited exclusively from the mother, which has significant implications for the study of mitochondrial diseases and genetic counseling
How does maternal inheritance influence the transmission of mitochondrial DNA?
Maternal inheritance significantly influences the transmission of mitochondrial DNA (mtDNA) in several key ways:
- Exclusivity of Maternal mtDNA: In mammals, mtDNA is inherited solely from the mother. During fertilization, while the sperm contributes its nuclear DNA, the mitochondria from the sperm are typically eliminated shortly after fertilization. This means that all offspring receive their mtDNA exclusively from the maternal lineage.
- High mtDNA Copy Number in Oocytes: Oocytes (egg cells) contain a much higher copy number of mtDNA compared to sperm cells. This high concentration of maternal mtDNA ensures that it is overwhelmingly represented in the zygote, further diluting any potential contribution from paternal mtDNA.
- Active Degradation of Paternal mtDNA: Mechanisms exist that actively degrade paternal mtDNA shortly after fertilization. This degradation is mediated by specific cellular processes, such as the action of mitochondrial endonuclease G, which helps ensure that only maternal mtDNA is retained in the developing embryo.
- Genetic Bottleneck: During the development of the female germline, a phenomenon known as the mitochondrial genetic bottleneck occurs. This process involves a significant reduction in the number of mtDNA molecules that are passed on to the next generation. As a result, the mtDNA that is eventually transmitted to the offspring can exhibit considerable variability, leading to differences in heteroplasmy levels among siblings.
- Implications for Genetic Counseling: Because mtDNA is maternally inherited, any mutations present in the maternal mtDNA can be passed on to all offspring. This has important implications for genetic counseling, as it allows for the prediction of the inheritance of mitochondrial diseases and the assessment of recurrence risks in families.
- Phenotypic Variation: The maternal inheritance of mtDNA can lead to significant phenotypic variation among siblings due to the random segregation of mtDNA variants during oogenesis and the effects of the genetic bottleneck. This variability can complicate the clinical presentation of mitochondrial disorders within families.
What types of mutations can occur in the mitochondrial genome, and how do they affect mitochondrial function?
Mutations in the mitochondrial genome can be classified into several types, each of which can have varying effects on mitochondrial function. The main types of mutations include:
- Point Mutations: These are single nucleotide changes in the mtDNA sequence. Point mutations can lead to:
- Missense Mutations: These result in the substitution of one amino acid for another in a protein, potentially altering its function. For example, mutations in genes encoding components of the oxidative phosphorylation pathway can impair ATP production.
- Nonsense Mutations: These create a premature stop codon, leading to truncated proteins that are often nonfunctional.
- Insertions and Deletions (Indels): These mutations involve the addition or loss of nucleotides in the mtDNA sequence. Indels can disrupt the reading frame of genes, leading to frameshift mutations that produce nonfunctional proteins. They can also affect the regulation of gene expression and the stability of the mitochondrial genome.
- Large Deletions: These involve the loss of significant segments of the mtDNA. Large deletions can result in the loss of essential genes required for mitochondrial function, leading to severe mitochondrial diseases. For example, Kearns-Sayre syndrome is associated with large deletions in mtDNA.
- Heteroplasmic Mutations: Mitochondrial DNA can exist in a heteroplasmic state, where a mixture of normal and mutated mtDNA coexists within the same cell. The proportion of mutated mtDNA can vary between tissues and individuals, leading to variable expressivity of mitochondrial diseases. This can result in a range of clinical symptoms, depending on the threshold of mutated mtDNA required to impair function.
- Depletion Mutations: These mutations lead to a significant reduction in mtDNA copy number, which can severely impair mitochondrial function. Mitochondrial DNA depletion syndromes are characterized by low levels of mtDNA in affected tissues, resulting in energy deficits and organ dysfunction.
Effects on Mitochondrial Function:
- Impaired ATP Production: Many mutations affect the components of the oxidative phosphorylation pathway, leading to reduced ATP synthesis, which is critical for cellular energy.
- Increased Reactive Oxygen Species (ROS) Production: Mutations can disrupt the electron transport chain, leading to increased production of ROS, which can cause further damage to mitochondrial and cellular components.
- Altered Metabolism: Mutations can affect metabolic pathways that rely on mitochondrial function, leading to metabolic disorders.
- Cellular Dysfunction and Death: Severe mitochondrial dysfunction can result in cell death, contributing to the pathology of various mitochondrial diseases, particularly in high-energy-demand tissues such as the brain, heart, and muscles.
What is heteroplasmy, and why is it significant in the context of mitochondrial diseases?
Heteroplasmy refers to the presence of a mixture of different mitochondrial DNA (mtDNA) variants within a single cell or tissue. This condition occurs when both normal (wild-type) and mutated mtDNA coexist in the mitochondria of an individual. The significance of heteroplasmy in the context of mitochondrial diseases includes several key aspects:
- Variable Expression of Mitochondrial Diseases: The proportion of mutated mtDNA can vary widely among different tissues and even among cells within the same tissue. This variability can lead to differences in the severity and type of symptoms experienced by individuals with mitochondrial diseases. For example, some tissues may have a higher load of mutated mtDNA, leading to more pronounced dysfunction and clinical manifestations.
- Threshold Effect: Many mitochondrial diseases are associated with a “threshold effect,” where a certain percentage of mutated mtDNA must be present before clinical symptoms manifest. This means that individuals with low levels of mutated mtDNA may be asymptomatic, while those with higher levels may exhibit severe symptoms. The threshold can vary depending on the specific mutation and the tissue affected.
- Genetic Counseling Challenges: Heteroplasmy complicates genetic counseling for families with mitochondrial diseases. Because the proportion of mutated mtDNA can change during cell division and development (due to processes like the mitochondrial genetic bottleneck), predicting the recurrence risk for offspring can be difficult. Siblings may inherit different levels of mutated mtDNA, leading to varying clinical outcomes.
- Impact on Treatment and Management: The presence of heteroplasmy can influence the approach to treatment and management of mitochondrial diseases. Understanding the levels of heteroplasmy in affected tissues can help guide therapeutic strategies and inform prognosis.
- Research Implications: Heteroplasmy is a critical factor in the study of mitochondrial genetics and the development of potential therapies. Research into methods for selectively targeting and reducing mutated mtDNA (such as mitoTALENs and ZFNs) is ongoing, with the goal of improving outcomes for individuals with high levels of deleterious mtDNA mutations.
Can you explain the concept of the mitochondrial bottleneck and its implications for mtDNA inheritance?
The mitochondrial bottleneck is a concept that describes a critical phase during the development of female germ cells (oocytes) in which a limited number of mitochondrial DNA (mtDNA) molecules are selected from a larger pool for replication and transmission to the next generation. This phenomenon has significant implications for mtDNA inheritance and the expression of mitochondrial diseases.
Key Aspects of the Mitochondrial Bottleneck:
- Reduction in mtDNA Copy Number: During oogenesis, there is a marked reduction in the number of mtDNA copies. This means that only a small subset of the total mtDNA present in the primordial germ cells is passed on to the developing oocyte. This sampling process can lead to a significant shift in the proportion of normal versus mutated mtDNA.
- Random Genetic Drift: The selection of mtDNA during the bottleneck occurs randomly, which can result in different levels of heteroplasmy in the offspring. Some oocytes may inherit a higher proportion of mutated mtDNA, while others may inherit a lower proportion, leading to variability in the expression of mitochondrial diseases among siblings.
- Implications for Inheritance:
- Phenotypic Variation: Because of the bottleneck, siblings from the same mother can exhibit a wide range of clinical symptoms and severity of mitochondrial diseases, even if they share the same maternal mtDNA mutation. This variability is due to the different levels of heteroplasmy inherited from the mother.
- Recurrence Risk: The bottleneck complicates the prediction of recurrence risk for mitochondrial diseases. Since the proportion of mutated mtDNA can vary significantly between offspring, genetic counseling becomes challenging. It is difficult to estimate the likelihood that a child will inherit a particular level of mutated mtDNA that could lead to disease.
- Selection Against Deleterious Mutations: The bottleneck may also serve as a filtering mechanism, where severely deleterious mutations are less likely to be passed on. This is thought to occur because the metabolic environment during oocyte development may favor the survival of mtDNA with less harmful mutations, thereby reducing the recurrence risk of certain mitochondrial disorders.
- Research and Therapeutic Implications: Understanding the mitochondrial bottleneck is crucial for developing potential therapies aimed at reducing the transmission of mutated mtDNA. Strategies that target the bottleneck phase could help in selectively amplifying healthy mtDNA while minimizing the transmission of deleterious mutations.
What are some common mitochondrial diseases associated with defects in the mitochondrial genome?
Common mitochondrial diseases associated with defects in the mitochondrial genome include a variety of syndromes, each characterized by specific clinical features and underlying genetic mutations. Some of the notable mitochondrial diseases are:
- Leber’s Hereditary Optic Neuropathy (LHON): This condition is primarily characterized by sudden vision loss due to the degeneration of retinal ganglion cells. It is often associated with mutations in mtDNA, particularly in genes encoding subunits of complex I of the mitochondrial respiratory chain.
- Mitochondrial Encephalomyopathy, Lactic Acidosis, and Stroke-like episodes (MELAS): MELAS is a multisystem disorder that can affect the brain and muscles, leading to symptoms such as seizures, headaches, and muscle weakness. It is commonly associated with the m.3243A>G mutation in the mitochondrial tRNA gene for leucine.
- Myoclonic Epilepsy with Ragged Red Fibers (MERRF): This syndrome is characterized by myoclonus, epilepsy, ataxia, and the presence of ragged red fibers in muscle biopsies. It is often linked to mutations in the mitochondrial tRNA gene for lysine (e.g., m.8344A>G).
- Kearns-Sayre Syndrome (KSS): KSS is a rare mitochondrial disorder that typically presents with progressive external ophthalmoplegia, pigmentary retinopathy, and cardiac conduction defects. It is often caused by large-scale deletions in mtDNA.
- Pearson Syndrome: This is a rare disorder that affects multiple systems, including the bone marrow and pancreas. It is associated with large deletions in mtDNA and can lead to sideroblastic anemia and pancreatic dysfunction.
- NARP Syndrome (Neuropathy, Ataxia, and Retinitis Pigmentosa): NARP is characterized by neurological symptoms, including neuropathy and ataxia, along with retinitis pigmentosa. It is often associated with the m.8993T>G mutation in the ATP6 gene, which affects ATP synthesis.
- Mitochondrial Diabetes and Deafness (MIDD): This condition is characterized by the combination of diabetes mellitus and sensorineural hearing loss. It is commonly associated with the m.3243A>G mutation, which can also cause MELAS.
- Leigh Syndrome: This is a severe neurological disorder that typically presents in infancy or early childhood. It is associated with various genetic defects, including those in mitochondrial genes, leading to energy production failure in the brain.
How do mitochondrial DNA deletions differ from point mutations in terms of their impact on cellular function?
Mitochondrial DNA (mtDNA) deletions and point mutations are two distinct types of genetic alterations that can occur in the mitochondrial genome, and they have different implications for cellular function. Here’s a comparison of their impacts:
Mitochondrial DNA Deletions
- Nature of the Mutation:
- Mitochondrial DNA deletions involve the loss of a segment of the mtDNA sequence. This can range from small deletions to large-scale deletions that remove multiple genes or regulatory regions.
- Impact on Gene Function:
- Deletions can result in the complete loss of function of one or more genes, particularly those encoding essential components of the oxidative phosphorylation (OXPHOS) pathway, which is crucial for ATP production.
- The loss of entire genes can disrupt the assembly of respiratory chain complexes, leading to deficiencies in ATP synthesis and increased production of reactive oxygen species (ROS).
- Clinical Manifestations:
- Mitochondrial deletions are often associated with specific syndromes such as Kearns-Sayre Syndrome (KSS) and Pearson Syndrome, which can lead to severe clinical symptoms, including muscle weakness, cardiac issues, and neurological deficits 12.
- Heteroplasmy:
- Deletions can lead to a high degree of heteroplasmy, where cells may contain a mixture of normal and deleted mtDNA. The proportion of deleted mtDNA can influence the severity of the phenotype, as a higher load of deleted mtDNA is often correlated with more severe disease manifestations.
Point Mutations
- Nature of the Mutation:
- Point mutations involve a change in a single nucleotide in the mtDNA sequence. This can result in missense mutations (which change an amino acid in a protein), nonsense mutations (which create a premature stop codon), or silent mutations (which do not change the amino acid sequence).
- Impact on Gene Function:
- Point mutations can affect the function of specific proteins, particularly those involved in the electron transport chain (ETC). For example, mutations in genes encoding subunits of the respiratory complexes can impair their enzymatic activity, leading to reduced ATP production and increased oxidative stress.
- The impact of point mutations can vary widely depending on the specific mutation and its location within the gene. Some mutations may have minimal effects, while others can lead to significant dysfunction.
- Clinical Manifestations:
- Point mutations are associated with a range of mitochondrial diseases, including Leber’s Hereditary Optic Neuropathy (LHON) and Myoclonic Epilepsy with Ragged Red Fibers (MERRF). The clinical presentation can vary based on the specific mutation and its effects on protein function .
- Heteroplasmy:
- Like deletions, point mutations can also exhibit heteroplasmy. The proportion of mutated versus normal mtDNA can influence the severity of the disease, with higher levels of mutated mtDNA often correlating with more severe symptoms.
What role do reactive oxygen species (ROS) play in mitochondrial dysfunction?
Reactive oxygen species (ROS) play a significant role in mitochondrial dysfunction, impacting cellular health and contributing to various diseases. Here are the key aspects of how ROS are involved in mitochondrial dysfunction:
1. Production of ROS in Mitochondria
- Mitochondria are the primary site of ATP production through oxidative phosphorylation, a process that involves the electron transport chain (ETC). During this process, electrons are transferred through a series of protein complexes, and some of these electrons can leak and react with oxygen to form ROS, such as superoxide anions (O2•−) and hydrogen peroxide (H2O2) 2.
2. Oxidative Stress
- When ROS production exceeds the capacity of the cellular antioxidant defense systems, it leads to oxidative stress. This condition can damage cellular components, including lipids, proteins, and DNA, ultimately impairing mitochondrial function and contributing to cell death 12.
3. Mitochondrial Damage
- ROS can directly damage mitochondrial DNA (mtDNA), leading to mutations and deletions that impair mitochondrial function. This damage can disrupt the synthesis of essential proteins involved in the ETC, further exacerbating energy production deficits and increasing ROS generation 9.
4. Disruption of Mitochondrial Dynamics
- Mitochondria are dynamic organelles that undergo fission and fusion processes to maintain their function and integrity. Elevated ROS levels can disrupt these processes, leading to mitochondrial fragmentation, which is associated with impaired energy metabolism and increased susceptibility to apoptosis 1.
5. Activation of Cell Death Pathways
- High levels of ROS can trigger apoptotic pathways, leading to programmed cell death. This is particularly relevant in conditions where mitochondrial dysfunction is present, as the release of cytochrome c from damaged mitochondria can activate caspases and promote apoptosis 2.
6. Role in Disease Pathogenesis
- Mitochondrial dysfunction and increased ROS production are implicated in various diseases, including neurodegenerative disorders (e.g., Alzheimer’s and Parkinson’s diseases), cardiovascular diseases, and metabolic syndromes. In these conditions, the interplay between ROS and mitochondrial dysfunction contributes to disease progression and severity 15.
7. Feedback Loop
- The relationship between ROS and mitochondrial dysfunction can create a feedback loop: increased ROS can lead to mitochondrial damage, which in turn can result in further ROS production. This cycle can perpetuate cellular damage and contribute to the pathophysiology of various diseases 12.
Keynote
- mtDNA in mammals is replicated by proteins separate from those employed for replication of nuclear DNA.
- In accordance with the strand displacement concept, replication begins from two separate origins, OH and OL.
- The primer used by POL to trigger DNA synthesis at OH is derived from LSP-initiated transcripts.
- OL produces a stem–loop structure, and POLRMT begins primer production in the loop region of single-stranded DNA.
- The function of the mitochondrial D-loop is unknown.
- RNASEH1 and MGME1 play crucial roles in primer elimination, but the specifics of this mechanism remain unclear.
- Top3α is necessary for the resolution of hemicatenane structures between newly replicated mtDNA molecules.
- mtDNA is not a free-floating molecule, but is instead packed into nucleoprotein complexes called nucleoids.
- mtDNA synthesis is linked to mitochondrial division.
References
- Apostolova, N., & Esplugues, J. V. (2011). Mitochondrial DNA Replication in Health and Disease. In (Ed.), DNA Replication – Current Advances. IntechOpen. https://doi.org/10.5772/19162
- Holt IJ, Reyes A. Human mitochondrial DNA replication. Cold Spring Harb Perspect Biol. 2012 Dec 1;4(12):a012971. doi: 10.1101/cshperspect.a012971. PMID: 23143808; PMCID: PMC3504440.
- Falkenberg M. Mitochondrial DNA replication in mammalian cells: overview of the pathway. Essays Biochem. 2018 Jul 20;62(3):287-296. doi: 10.1042/EBC20170100. PMID: 29880722; PMCID: PMC6056714.
- Gustafson, Margaret & McCormick, Elizabeth & Perera, Lalith & Longley, Matthew & Bai, Renkui & Kong, Jianping & Dulik, Matthew & Shen, Lishuang & Goldstein, Amy & Mccormack, Shana & Laskin, Benjamin & Leroy, Bart & Ortiz-Gonzalez, Xilma & Ellington, Meredith & Copeland, William & Falk, Marni. (2019). Mitochondrial single-stranded DNA binding protein novel de novo SSBP1 mutation in a child with single large-scale mtDNA deletion (SLSMD) clinically manifesting as Pearson, Kearns-Sayre, and Leigh syndromes. PLOS ONE. 14. e0221829. 10.1371/journal.pone.0221829.
- http://genesdev.cshlp.org/content/19/20/2466/F1.expansion.html
- https://biocyclopedia.com/index/genetics/chemistry_of_the_gene_synthesis_modification_and_repair_of_dna/mitochondrial_dna_replication_and_dloops.php
- https://exama2z.in/mitochondrial-dna-replication/
- https://www.news-medical.net/life-sciences/Mitochondrial-DNA-Organization-and-Replication-Steps.aspx
- https://www.sciencedirect.com/topics/biochemistry-genetics-and-molecular-biology/mitochondrial-dna-replication