Ever wondered how your cells turn the food you eat into usable energy? Let’s break down the Krebs cycle—a cornerstone of cellular respiration—and answer the questions you’ve been searching for. Also known as the citric acid cycle, this process is a series of chemical reactions that extract energy from nutrients. But where does this critical cycle happen? If you’ve ever asked, “Where does the Krebs cycle take place?” the answer lies deep within your cells. Specifically, it occurs in the mitochondrial matrix, the innermost compartment of mitochondria, often dubbed the cell’s “powerhouse.”
Now, what exactly does the Krebs cycle do? It acts as a metabolic hub, breaking down acetyl-CoA (derived from carbs, fats, and proteins) into carbon dioxide while generating energy-rich molecules. Key products of the Krebs cycle include ATP, the cell’s energy currency, along with electron carriers like NADH and FADH2, which fuel later stages of energy production. Speaking of ATP, you might ask, “How many ATP are produced directly in the Krebs cycle?” The answer? Two ATP molecules per glucose molecule—modest on its own, but the real magic happens when NADH and FADH2 power the electron transport chain, producing far more ATP.
Here’s a crucial detail: the Krebs cycle is aerobic, meaning it requires oxygen indirectly. While oxygen isn’t used directly in the cycle itself, it’s essential for recycling NADH and FADH2 in later stages. Without oxygen, the cycle grinds to a halt—making it a hallmark of aerobic respiration.
What is the krebs cycle?
- The Krebs Cycle is also known as The citric acid cycle or TCA cycle (tricarboxylic acid cycle).
- Krebs Cycle is cellular respiration, where involves a series of chemical reactions that release the stored energy via oxidation of acetyl-CoA. The aerobic organisms perform this TCA cycle.
- The acetyl-CoA is generated from Pyruvate (End product of Glycolysis) by the enzyme Pyruvate dehydrogenase complex and in the presence of coenzymes TPP, NAD, FAD, Lipoate, acetyl CoA.
- In 1937, Hans Adolf Krebs discovered The citric acid cycle, for which he received Nobel Prize for Physiology or Medicine in 1953.
- The total amount of energy yield from the TCA cycle is three NADH, one FADH2, and one GTP.
- The Krebs cycle occurs in the Mitochondria of eukaryotic cells and in Prokaryotic cells (lacks mitochondria) or bacteria Krebs cycle takes place in the cytosol.
Where does krebs cycle occur?
- In eukaryotic organisms the Krebs cycle occurs in the mitochondrial matrix.
- Comprising the innermost section of the mitochondrion surrounded by the inner membrane, the mitochondrial matrix is
- Along with required coenzymes (NAD⁺, FAD), it includes all eight cycle enzymes as well as inorganic phosphates.
- In prokaryotic cells the Krebs cycle takes place in the cytoplasm.
- Prokaryotes lack mitochondria, hence the cytoplasmic fluid breaks down all cycle enzymes and substrates.
- Unlike a mitochondrial membrane, the proton gradient for oxidative phosphorylation spans the plasma membrane.
Krebs Cycle Steps
After glycolysis, in aerobic organisms, pyruvate is carboxylated to form acetyl CoA and CO2.
- The process is called oxidative decarboxylation.
- This step links glycolysis to the citric acid cycle.
- Pyruvate is oxidatively decarboxylated to acetyl CoA and CO2 by the pyruvate dehydrogenase complex, found in the mitochondrial matrix of eukaryotes and the cytoplasm of prokaryotes.
- From one glucose molecule, two pyruvate molecules are formed, each generating one acetyl CoA and one NADH.
- The acetyl CoA produced enters the citric acid cycle.
- Step 1: Condensation of acetyl CoA with oxaloacetate
- Acetyl CoA (a two-carbon compound) condenses with oxaloacetate (a four-carbon compound).
- This reaction, catalyzed by citrate synthase, forms the six-carbon compound citric acid.
- The process involves the formation of citryl-CoA, which is then cleaved to release coenzyme A and form citrate.
- Step 2: Isomerization of citrate to isocitrate
- Citrate is isomerized to isocitrate via the intermediate cis-aconitase.
- The reaction is reversible and is catalyzed by aconitase.
- The two-step process involves first the dehydration of citrate to cis-aconitase, followed by rehydration to form isocitrate.
- Step 3: Oxidative decarboxylation of isocitrate
- Isocitrate undergoes oxidative decarboxylation to form α-ketoglutarate, a five-carbon compound.
- This is the first oxidation-reduction reaction in the cycle, catalyzed by isocitrate dehydrogenase.
- In the first step, isocitrate is dehydrogenated to oxalosuccinate, which is then decarboxylated to form α-ketoglutarate.
- NADH is produced in the dehydrogenation step, while CO2 is released during decarboxylation.
- Step 4: Oxidative decarboxylation of α-ketoglutarate
- α-Ketoglutarate is oxidatively decarboxylated to succinyl-CoA, a four-carbon compound.
- This reaction is catalyzed by α-ketoglutarate dehydrogenase and involves the reduction of NAD+ to NADH.
- Like the decarboxylation of pyruvate, CO2 is released in this reaction.
- Step 5: Conversion of succinyl-CoA to succinate
- Succinyl-CoA is cleaved to form succinate, generating GTP from GDP.
- GTP then transfers its terminal phosphate to ADP, forming ATP.
- This energy-conserving reaction is catalyzed by succinyl-CoA synthase.
- Step 6: Dehydration of succinate to fumarate
- Succinate is dehydrogenated to fumarate by succinate dehydrogenase.
- This reaction involves the reduction of FAD to FADH2, which enters the electron transport chain.
- FADH2 transfers electrons to ubiquinone, eventually forming 2 ATPs.
- Step 7: Hydration of fumarate to malate
- Fumarate is hydrated to form L-malate by the enzyme fumarate hydratase.
- This is a reversible reaction, with hydration of fumarate forming malate and dehydration of malate forming fumarate.
- Step 8: Dehydrogenation of L-malate to oxaloacetate
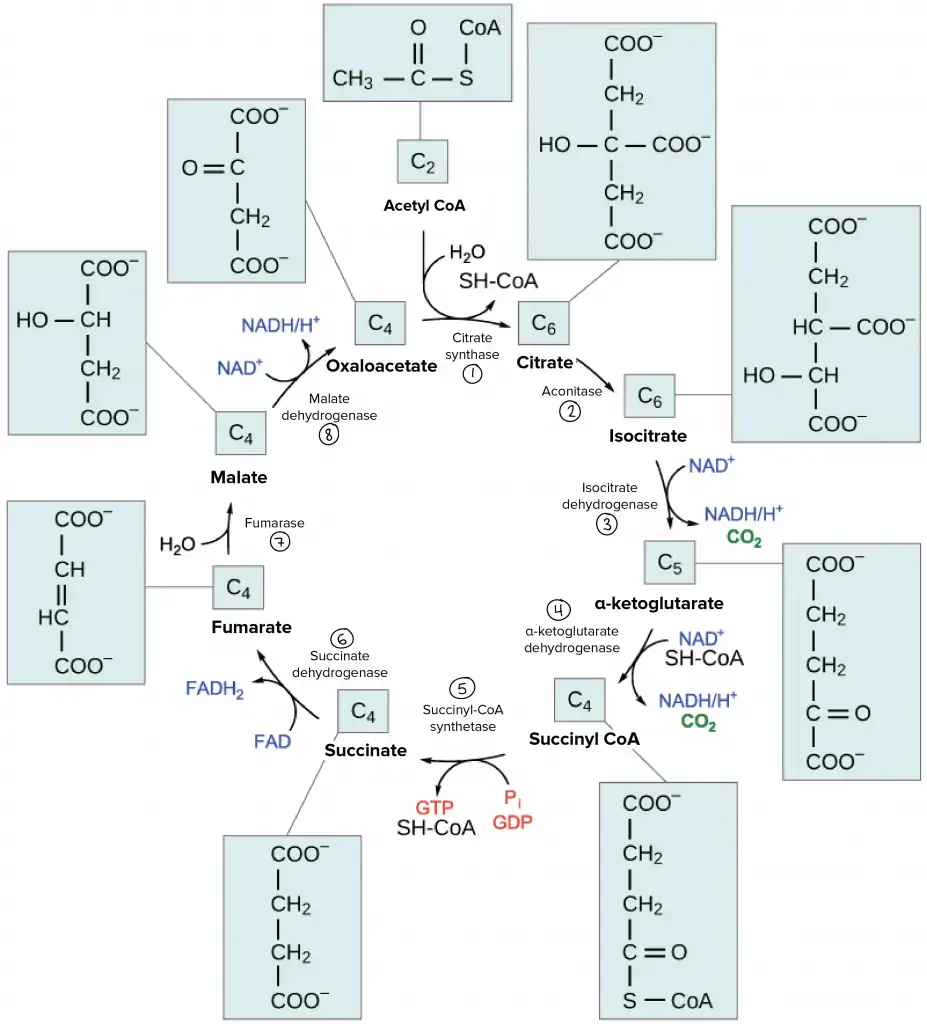
The Overall Krebs cycle equation is;
2 acetyl groups + 6 NAD+ + 2 FAD + 2 ADP + 2 Pi = 4 CO2 + 6 NADH + 6 H+ + 2 FADH2 + 2 ATP
Krebs Cycle Enzymes
Citrate synthase
- Forms citrate and CoA‑SH by catalyzing the irreversible condensation of acetyl‑CoA with oxaloacetate.
- stabilizes the enolate intermediate and active site conformation by use of Mg²⁺ as a cofactor.
- allosterically inhibited by ATP, NADH and succinyl-CoA; product-inhibited by citrate to control cycle flow
- located in the mitochondrial matrix, indicating the acetyl-CoA entrance site into the cycle
- acts as a fundamental control point combining substrate availability with energy status by means of feedback inhibition.
Aconitase
- uses the intermediate cis-aconitate to drive the reversible isomerization of citrate to isocitrate.
- includes a [4Fe–4S] iron–sulfur cluster essential for catalysis and substrate binding.
- susceptible to fluorocitrate, an antimetabolite that compromises the cluster, and oxidative inactivation—e.g., by superoxide
- linked to the inner mitochondrial membrane in eukaryotes, therefore enabling substrate channeling
- guarantees the right stereochemistry for the oxidative decarboxylation phase that comes next.
Isocitrate dehydrogenase
- generates NADH and CO₂ by catalyzing the oxidative decarboxylation of isocitrate to α‑ketoglutarate.
- employs NAD⁺ (or NADP⁺ in certain isoforms) as electron acceptor and needs Mn²⁺, or Mg²⁺, for catalytic activity.
- in various circumstances, triggered allosteristically by ADP and inhibited by ATP and NADH, hence limiting the pace of the cycle.
- found in the mitochondrial matrix to connect redox homeostasis with energy production.
- uses cues of cellular energy charge to control cycle throughput.
α‑Ketoglutarate dehydrogenase complex
- catalyzes the irreversible oxidative decarboxylation of α‑ketoglutarate toward succinyl‑CoA, CO₂, and NADH
- three subunit multienzyme complex needing thiamine pyrophosphate (E₁), lipoic acid and CoA (E₂), FAD and NAD⁺ (E₃)
- limited by its products NADH and succinyl‑CoA through feedback systems to stop downstream intermediary overaccumulation
- live in the mitochondrial matrix to help cofactor recycling and substrate channeling.
- acts as a metabolic crosspoint between amino acid/nitrogen metabolism and energy generation.
Succinyl‑CoA synthetase
- catalyzes the reversible cleavage of succinyl‑CoA to succinate, CoA and GTP (or ATP), conserving energy via substrate‑level phosphorylation
- requires Mg²⁺ for enzyme stability and phosphate binding
- no major allosteric regulation reported; activity driven by substrate availability and GTP/ATP demand
- localized in the mitochondrial matrix to directly contribute to the local nucleotide pool
- links the citric acid cycle with GTP‑dependent biosynthetic processes such as protein synthesis
Succinate dehydrogenase
- catalyzes the oxidation of succinate to fumarate while reducing FAD to FADH₂, feeding electrons into the electron transport chain
- contains covalently bound FAD and multiple iron–sulfur clusters as prosthetic groups
- competitive inhibitors include malonate, malate and oxaloacetate, which bind the active site to modulate activity
- embedded in the inner mitochondrial membrane as Complex II, linking the Krebs cycle with oxidative phosphorylation
- defects or mutations in SDH subunits are implicated in certain tumors and neurodegenerative diseases
Fumarase
- catalyzes the reversible hydration of fumarate to L‑malate, ensuring stereospecific addition of water
- does not require a cofactor beyond ordered water and Mg²⁺ for structural stability
- no prominent allosteric regulators; reaction driven by substrate/product concentrations
- localized in the mitochondrial matrix and, in some organisms, dual‑targeted to the cytosol/nucleus for other functions
- mutations in FH cause fumarase deficiency, leading to severe metabolic and neurological disorders
Malate dehydrogenase
- catalyzes the oxidation of L‑malate to oxaloacetate with reduction of NAD⁺ to NADH, completing the cycle
- requires NAD⁺ and Mg²⁺ for enzymatic function and stabilization of transition state
- inhibited by high NADH/NAD⁺ ratios and regulated via multienzyme complex interactions for efficient flux
- located in the mitochondrial matrix to replenish oxaloacetate for citrate synthase and link to gluconeogenesis
- activity modulation integrates redox state with anaplerotic and cataplerotic pathways
Krebs Cycle Products
2 molecules of CO₂ produced per acetyl‑CoA oxidized
3 NADH generated per cycle turn by oxidation of intermediates
1 FADH₂ produced per cycle turn via succinate dehydrogenase
1 GTP (convertible to ATP) synthesized per turn by substrate‑level phosphorylation
Oxaloacetate regenerated at the end of the cycle allowing continuous cycling
Net cycle yields per glucose molecule (two acetyl‑CoA oxidations)
- 4 CO₂
- 6 NADH
- 2 FADH₂
- 2 GTP (ATP)
Approximate ATP yield per cycle turn
- NADH oxidation yields ~7.5 ATP (3 × 2.5)
- FADH₂ oxidation yields ~1.5 ATP
- GTP contributes 1 ATP
- Total ~10 ATP per turn
Contribution to overall cellular ATP production
- Cycle alone generates ~20 ATP per glucose (2 turns × 10 ATP)
- Combined with glycolysis and oxidative phosphorylation theoretical yield ~30–38 ATP per glucose Wikipedia
Provides reducing equivalents to the electron transport chain linking metabolism of carbohydrates, fats and proteins to ATP synthesis
Regulation of Krebs Cycle
- The high ratio of [ATP]/[ADP], [NADH]/[NAD], and [acetyl-CoA]/[CoA] allosterically inhibits the PDH complex. If these ratios are decrease, the allosteric activation of pyruvate oxidation results.
- Increased rate of Calcium ion in mitochondrial matrix activates the pyruvate dehydrogenase complex, isocitrate dehydrogenase, and α-ketoglutarate dehydrogenase.
- The Long-chain acetyl CoA and ATP allosterically inhibits the Citrate synthase.
- NADH and ATP inhibits the Isocitrate dehydrogenase.
- NADH and succinyl CoA inhibits the α-ketoglutarate dehydrogenase.
- Oxaloacetate inhibits the succinate dehydrogenase enzyme.
- The citrate blocks citrate synthase.
Difference between glycolysis and krebs cycle
Characters | Krebs Cycle | Glycolysis |
Definition | Krebs cycle, also known as the citric acid cycle or tricarboxylic acid cycle (TCA cycle), refers to the series of chemical reactions in which pyruvate is converted to acetyl-CoA and is completely oxidized into carbon dioxide and water. | Glycolysis refers to the series of chemical reaction in which a glucose molecule is converted into two pyruvic acid molecules. |
Location | Krebs cycle occurs inside the mitochondria of eukaryotes. | Glycolysis occurs in the cytoplasm. |
Process | The Krebs cycle is involved in the complete oxidation of pyruvate into carbon dioxide and water. | The glycolysis is involved in the degradation of glucose into two molecules of pyruvate. |
Aerobic/Anaerobic Respiration | The Krebs cycle only occurs in aerobic respiration. | The glycolysis occurs in both aerobic and anaerobic respiration. |
Step | Krebs cycle is the second step of the cellular respiration. | Glycolysis is the first step of the cellular respiration. |
Carbon Dioxide | Carbon dioxide is released during the process of Krebs cycle. | No carbon dioxide is released during the process of glycolysis. |
End Product | The end product of Krebs cycle is an inorganic carbon substance. | The end product of glycolysis is an organic substance. |
Net Gain of Energy | The net gain of energy of the Krebs cycle is equal to 24 ATP molecules. | The net gain of energy of the glycolysis is equal to 8 ATP molecules. |
Net Gain | Krebs cycle produces six NADH molecules and two FADH2 molecules. | Glycolysis produces two pyruvate molecules, two ATP molecules, two NADH molecules. |
Oxidative Phosphorylation | Krebs cycle is connected with the oxidative phosphorylation. | Glycolysis is not connected with the oxidative phosphorylation. |
Linear/Cyclic | The Krebs cycle is a cyclic process. | The glycolysis is a linear process. |
Consumption of ATP | Krebs cycle consumes no ATP. | Glycolysis consumes two ATP molecules. |
Oxygen | The Krebs cycle uses oxygen as the terminal oxidant. | Glycolysis does not require oxygen. |
- Cooper GM. The Cell: A Molecular Approach. 2nd edition. Sunderland (MA): Sinauer Associates; 2000. The Mechanism of Oxidative Phosphorylation. Available from: https://www.ncbi.nlm.nih.gov/books/NBK9885/
- https://www.vaia.com/en-us/textbooks/chemistry/principles-of-biochemistry-4-edition/chapter-13/problem-3-calculate-the-number-of-atp-molecules-generated-by/
- https://en.wikipedia.org/wiki/Citric_acid_cycle
- https://www.jove.com/science-education/v/10977/products-of-the-citric-acid-cycle-nadh-fadh2-atp-and-co2
- https://pediaa.com/difference-between-krebs-cycle-and-glycolysis/
- https://en.wikipedia.org/wiki/Citric_acid_cycle
- https://www.khanacademy.org/science/biology/cellular-respiration-and-fermentation/pyruvate-oxidation-and-the-citric-acid-cycle/a/the-citric-acid-cycle