The nervous system relies on neurons to carry out various functions, ranging from basic motor reflexes to complex processes such as memory formation and decision-making. Unlike human communication through words and body language, neurons communicate through electrical and chemical signals. Similar to a committee member, a single neuron typically integrates and processes information from multiple neurons before transmitting the message to others.
Nerve Impulse Transmission within a Neuron
To enable the functioning of the nervous system, neurons require the ability to transmit and receive signals. This signal transmission is made possible by the presence of a charged cellular membrane within each neuron, which maintains a voltage difference between its interior and exterior. The membrane charge can be altered in response to neurotransmitter molecules released from neighboring neurons and external stimuli. To grasp the mechanism of neuronal communication, it is crucial to comprehend the fundamental concept of the resting membrane potential.
Neuronal Charged Membranes
- The neuronal charged membrane is a vital component of neurons, serving as a selectively permeable barrier that separates the intracellular and extracellular environments. Composed of a lipid bilayer, this membrane plays a crucial role in maintaining the delicate balance of ions within the neuron. While the lipid bilayer itself is impermeable to charged molecules or ions, specialized proteins known as ion channels are responsible for allowing ions to enter or exit the neuron.
- Ion channels are integral membrane proteins that span the lipid bilayer, creating passageways for specific ions. These channels exhibit different states or configurations, including open, closed, and inactive, as depicted in Figure 35.9. Some ion channels require activation to open up and facilitate the movement of ions across the membrane. These types of channels are highly sensitive to their surrounding environment and can adapt their shape accordingly.
- Voltage-gated ion channels represent a prominent class of ion channels that respond to changes in membrane potential. These channels undergo conformational changes in response to voltage fluctuations, altering their structure to permit or restrict ion flow. By regulating the relative concentrations of different ions inside and outside the cell, voltage-gated ion channels contribute to the establishment and maintenance of the membrane potential.
- The membrane potential refers to the difference in total charge between the interior and exterior of the cell. This potential arises due to the uneven distribution of ions across the neuronal membrane. Typically, the intracellular environment contains a higher concentration of negatively charged ions compared to the extracellular space. Consequently, the neuronal charged membrane maintains a negative resting membrane potential, typically around -70 millivolts.
- Through the coordinated action of ion channels, the membrane potential can be altered, resulting in electrical signals within the neuron. When the membrane potential changes, voltage-gated ion channels respond accordingly, allowing specific ions to flow across the membrane. This movement of ions generates electrical impulses, which propagate along the neuron, facilitating communication between different parts of the nervous system.
- In summary, the neuronal charged membrane, composed of a lipid bilayer, acts as a barrier that selectively controls the passage of ions. Ion channels, including voltage-gated ion channels, are essential components of this membrane, enabling the regulated movement of ions. These channels respond to changes in membrane potential, which contributes to the establishment of the membrane potential and facilitates the transmission of electrical signals within neurons. The dynamic interplay between ion channels and the neuronal charged membrane underlies the fundamental processes of neuronal function and communication.
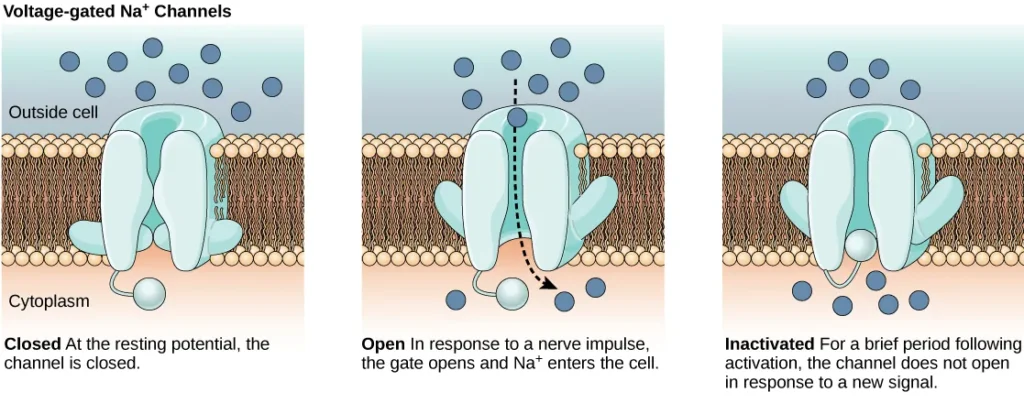
Resting Membrane Potential
The resting membrane potential of a neuron refers to its electrical charge when it is not actively transmitting signals. Typically, a neuron at rest maintains a negative charge, with the inside of the cell being approximately 70 millivolts more negative than the outside (denoted as -70 mV). It’s important to note that this value can vary among different neuron types and species.
The resting membrane potential arises from variations in ion concentrations inside and outside the cell. If the membrane were equally permeable to all ions, an equilibrium would be reached, and each type of ion would flow across the membrane. However, the membrane selectively allows certain ions to pass, leading to different ion concentrations inside and outside the cell, as indicated in Table 35.1.
The primary contributor to the resting membrane potential is the difference in the concentrations of positively charged potassium ions (K+) inside and outside the cell, as illustrated in Figure 35.10. At rest, K+ ions accumulate inside the cell due to a net movement along the concentration gradient. The negative charge within the cell is established and maintained by increasing the concentration of cations (positively charged ions) outside the cell relative to the inside.
In neurons, potassium ions are maintained at high concentrations inside the cell, while sodium ions are kept at high concentrations outside of the cell. The cell membrane is more permeable to potassium ion movement compared to sodium ion movement. Potassium and sodium leakage channels allow these cations to diffuse down their concentration gradient. However, neurons possess a larger number of potassium leakage channels compared to sodium leakage channels.
As a result, potassium ions diffuse out of the cell at a faster rate than sodium ions leak in, causing more cations to leave the cell than enter. This leads to a negative charge inside the cell relative to the outside. The movement of ions is facilitated by the actions of the sodium-potassium pump, which actively transports two potassium ions into the cell while removing three sodium ions per ATP consumed. This pump helps maintain the established resting potential.
In addition to potassium and sodium, chloride ions (Cl-) tend to accumulate outside of the cell. They are repelled by negatively charged proteins within the cytoplasm, contributing to the distribution of ions across the membrane.
The delicate balance of ion concentrations and the selective permeability of the neuronal membrane play a critical role in establishing and maintaining the resting membrane potential. This electrical charge differential sets the stage for the transmission of electrical signals and enables neurons to communicate and process information in the complex network of the nervous system.
Ion Concentration Inside and Outside Neurons | |||
---|---|---|---|
Ion | Extracellular concentration (mM) | Intracellular concentration (mM) | Ratio outside/inside |
Na+ | 145 | 12 | 12 |
K+ | 4 | 155 | 0.026 |
Cl− | 120 | 4 | 30 |
Organic anions (A−) | — | 100 |
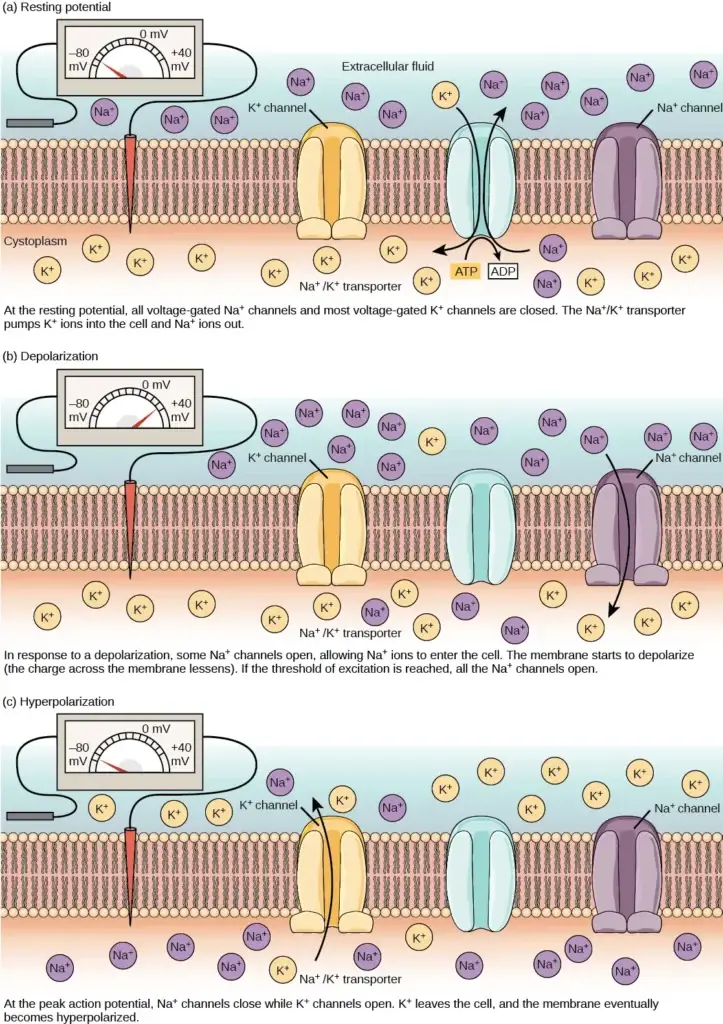
Action Potential
- Neurons possess the remarkable ability to receive signals from other neurons and, if the input is strong enough, transmit those signals to downstream neurons. While the transmission of signals between neurons primarily relies on neurotransmitters, the transmission of signals within a neuron occurs through a brief but crucial event known as an action potential.
- When neurotransmitter molecules bind to receptors located on the dendrites of a neuron, ion channels open. At excitatory synapses, this opening allows positive ions to enter the neuron, leading to depolarization of the membrane. Depolarization refers to a decrease in the voltage difference between the inside and outside of the neuron.
- When a stimulus from a sensory cell or another neuron reaches the target neuron and depolarizes it to its threshold potential (-55 mV), a cascade of events is triggered. Sodium (Na+) channels located in the axon hillock, the junction between the cell body and the axon, open. This opening permits positive ions, primarily sodium ions, to rush into the cell.
- As a result, the neuron undergoes complete depolarization, reaching a membrane potential of approximately +40 mV. Action potentials are considered an “all-or-nothing” event, meaning that once the threshold potential is reached, the neuron consistently depolarizes in its entirety.
- Following complete depolarization, the cell must reset its membrane voltage back to the resting potential. The sodium channels close and enter a refractory period, rendering them unresponsive to further depolarization. Simultaneously, voltage-gated potassium (K+) channels open, enabling potassium ions to exit the cell. As K+ ions leave, the membrane potential becomes negative once again.
- The diffusion of K+ out of the cell induces hyperpolarization, where the membrane potential becomes more negative than the neuron’s normal resting potential. During this time, the sodium channels return to their resting state, poised to open again if the membrane potential surpasses the threshold.
- Gradually, the extra K+ ions diffuse out of the cell via potassium leakage channels, eventually restoring the neuron from its hyperpolarized state back to its resting membrane potential. At this point, the neuron is once again capable of generating another action potential when stimulated appropriately.
- The action potential serves as a rapid and efficient means of transmitting signals within a neuron, allowing for the swift propagation of information across the nervous system. This process of depolarization, repolarization, and returning to the resting potential ensures that the action potential is a precisely regulated event, maintaining the integrity and functionality of neural communication.
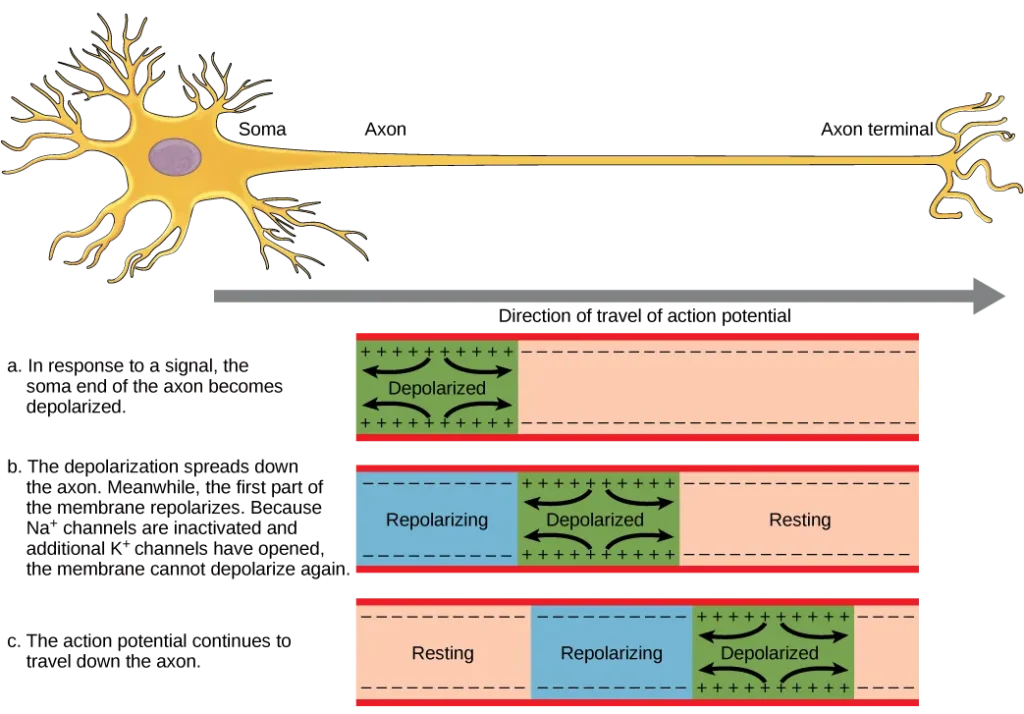
Myelin and the Propagation of the Action Potential
- The transmission of information between neurons requires the action potential to travel along the axon and reach the axon terminals, where it can initiate the release of neurotransmitters. The speed at which an action potential propagates along an axon is influenced by two factors: the diameter of the axon and the axon’s resistance to current leakage. Myelin, a specialized insulating substance, plays a critical role in enhancing the speed of action potential conduction.
- By acting as an insulator, myelin prevents the leakage of current from the axon, allowing the action potential to travel more efficiently. This insulation is particularly important in maintaining the speed of conduction. In demyelinating diseases such as multiple sclerosis, where the myelin sheath is damaged, the conduction of action potentials slows down due to current leakage from previously insulated areas of the axon.
- The nodes of Ranvier, which are gaps in the myelin sheath along the axon, play a crucial role in the propagation of the action potential, as depicted in Figure 35.13. These unmyelinated spaces, approximately one micrometer long, contain voltage-gated sodium (Na+) and potassium (K+) channels. The flow of ions through these channels, especially the Na+ channels, allows for the regeneration of the action potential along the axon.
- This phenomenon, known as saltatory conduction, involves the “jumping” of the action potential from one node to the next. It significantly speeds up the propagation of the action potential compared to continuous conduction along the entire axon. In the absence of nodes of Ranvier, the action potential would propagate very slowly because Na+ and K+ channels would have to continuously regenerate action potentials at every point along the axon instead of specific points.
- Furthermore, the presence of nodes of Ranvier contributes to energy efficiency for the neuron. Since the channels required for action potential regeneration are only necessary at the nodes, energy is conserved as they do not need to be present along the entire length of the axon.
- In summary, myelin plays a crucial role in enhancing the speed of action potential conduction by acting as an insulator that prevents current leakage. The nodes of Ranvier, unmyelinated gaps along the axon, allow for the regeneration of the action potential and facilitate saltatory conduction. This specialized mode of propagation, in which the action potential jumps from node to node, significantly speeds up the transmission of neural signals while also conserving energy for the neuron.
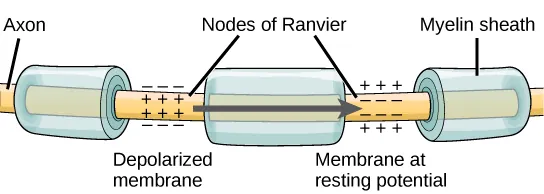
Synaptic Transmission
Synaptic transmission is the process through which information is transmitted from one neuron to another. The synapse, also known as the “gap,” serves as the site where this transmission occurs. While synapses typically form between the axon terminals of one neuron and the dendritic spines of another, it’s important to note that other types of synapses exist as well. These include axon-to-axon, dendrite-to-dendrite, and axon-to-cell body synapses, expanding the diversity of synaptic connections.
In the synaptic transmission process, the neuron that sends the signal is referred to as the presynaptic neuron, while the neuron that receives the signal is known as the postsynaptic neuron. It’s worth mentioning that these designations are relative to a specific synapse, as most neurons can function as both presynaptic and postsynaptic neurons depending on the synapse under consideration.
There are two main types of synapses: chemical and electrical synapses.
- Chemical Synapses: Chemical synapses are the most common type of synapse in the nervous system. They involve the release of chemical messengers, called neurotransmitters, from the presynaptic neuron. When an action potential reaches the axon terminals of the presynaptic neuron, it triggers the release of neurotransmitters into the synaptic cleft, a narrow space between the presynaptic and postsynaptic neurons. The neurotransmitters then bind to specific receptors located on the postsynaptic neuron’s dendrites, initiating a cascade of events that lead to the transmission of the signal. This transfer of information from the presynaptic neuron to the postsynaptic neuron is crucial for neuronal communication and information processing in the brain.
- Electrical Synapses: In contrast to chemical synapses, electrical synapses involve the direct flow of electrical current between neurons. They are characterized by specialized channels called gap junctions, which connect the cytoplasm of adjacent neurons. These gap junctions allow ions to pass freely from one neuron to another, facilitating rapid and synchronous electrical communication. Electrical synapses are particularly prevalent in certain regions of the brain and play a crucial role in the coordination of neural activity, such as in the synchronization of neuronal firing.
Both chemical and electrical synapses contribute to the complex network of connections in the nervous system, enabling the transmission of signals and the integration of information across neurons. The balance between these two types of synapses and their specific properties contribute to the intricate functionality of the brain and its ability to process and respond to stimuli.
Chemical Synapse
- The chemical synapse serves as a crucial junction for transmitting information between neurons. When an action potential reaches the axon terminal of the presynaptic neuron, it triggers a series of events that lead to the release of neurotransmitters and subsequent communication with the postsynaptic neuron.
- Upon reaching the axon terminal, the action potential causes depolarization of the presynaptic membrane, resulting in the opening of voltage-gated Na+ channels. As a result, Na+ ions flow into the cell, further depolarizing the membrane. This depolarization, in turn, triggers the opening of voltage-gated Ca2+ channels.
- The entry of calcium ions into the presynaptic neuron initiates a signaling cascade that ultimately leads to the fusion of synaptic vesicles with the presynaptic membrane. These small membrane-bound vesicles, known as synaptic vesicles, contain neurotransmitter molecules. Fusion of a vesicle with the presynaptic membrane causes the release of neurotransmitters into the synaptic cleft, the extracellular space between the presynaptic and postsynaptic membranes.
- The neurotransmitter molecules then diffuse across the synaptic cleft and bind to specific receptor proteins located on the postsynaptic membrane. The binding of a particular neurotransmitter to its receptors triggers the opening of ion channels on the postsynaptic membrane, specifically ligand-gated channels. Depending on the specific neurotransmitter and receptor, the postsynaptic response can be excitatory or inhibitory.
- For example, the release of acetylcholine at the neuromuscular junction between a nerve and a muscle triggers the opening of Na+ channels on the postsynaptic membrane, causing depolarization. This depolarization, known as an excitatory postsynaptic potential (EPSP), increases the likelihood of the postsynaptic neuron firing an action potential. On the other hand, release of inhibitory neurotransmitters, such as GABA, leads to the opening of Cl- channels, causing hyperpolarization of the membrane and resulting in an inhibitory postsynaptic potential (IPSP).
- To prepare for subsequent signals, the neurotransmitter must be removed from the synaptic cleft. This can occur through diffusion away from the cleft, degradation by enzymes in the cleft, or reuptake by the presynaptic neuron. Some drugs, like those used for Alzheimer’s disease, inhibit the degradation of neurotransmitters, thereby enhancing neurotransmission at synapses involving those specific neurotransmitters.
- Chemical synapses play a vital role in neural communication and information processing in the central nervous system (CNS) and peripheral nervous system (PNS). They allow for precise and dynamic interactions between neurons, contributing to the complex functioning of the nervous system.
- Neurotransmitters, such as acetylcholine, biogenic amines (dopamine, serotonin, norepinephrine), amino acids (glycine, glutamate, aspartate, gamma-aminobutyric acid), and neuropeptides (substance P, endorphins), are involved in neurotransmission at various locations within the CNS and/or PNS. These neurotransmitters exhibit diverse functions and regulate different physiological processes within the nervous system.
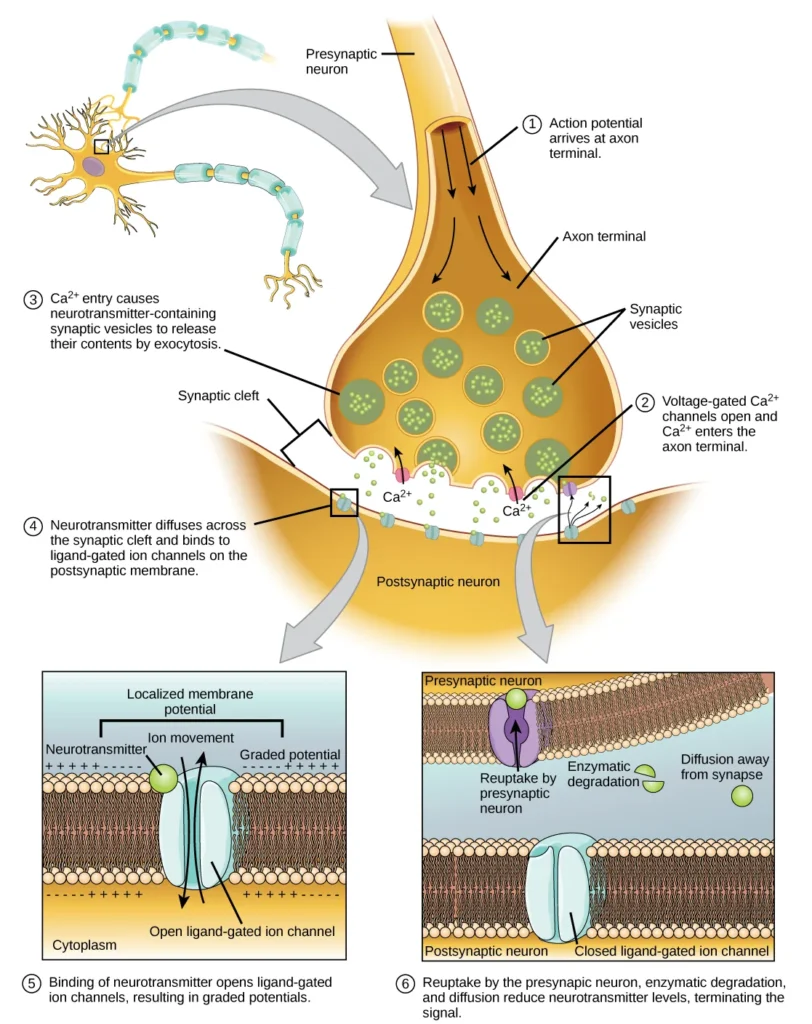
Neurotransmitter Function and Location | ||
---|---|---|
Neurotransmitter | Example | Location |
Acetylcholine | — | CNS and/or PNS |
Biogenic amine | Dopamine, serotonin, norepinephrine | CNS and/or PNS |
Amino acid | Glycine, glutamate, aspartate, gamma aminobutyric acid | CNS |
Neuropeptide | Substance P, endorphins | CNS and/or PNS |
Electrical Synapse
- While less common compared to chemical synapses, electrical synapses are present throughout the nervous system and serve distinct and vital functions. Unlike chemical synapses, electrical synapses involve direct electrical communication between cells, facilitated by specialized proteins called gap junctions. These gap junctions physically connect the presynaptic and postsynaptic membranes, allowing the flow of current and certain molecules, such as ATP, between the cells.
- One key distinction between electrical and chemical synapses lies in their mode of neurotransmission. In chemical synapses, the signal transmission involves the release of neurotransmitter molecules from synaptic vesicles, which introduces a delay of approximately one millisecond between the arrival of the action potential at the presynaptic terminal and the subsequent opening of ion channels in the postsynaptic membrane. However, electrical synapses enable near-instantaneous signaling, making them particularly important for synapses involved in rapid reflexes. Furthermore, while chemical synapses are unidirectional, electrical synapses can be bidirectional, allowing for bi-directional flow of electrical signals between cells.
- Electrical synapses offer certain advantages over chemical synapses. They exhibit higher reliability, as they are less likely to be blocked or disrupted. Additionally, electrical synapses play a crucial role in synchronizing the electrical activity of groups of neurons. For instance, in the thalamus, electrical synapses are believed to regulate slow-wave sleep, and disturbances in these synapses can lead to seizures.
- Despite being less prevalent, electrical synapses are significant in facilitating rapid and synchronized communication within the nervous system. They provide a direct pathway for the transmission of electrical signals and contribute to the coordination and efficiency of neural circuitry.
Signal Summation
- In order for a postsynaptic neuron to generate an action potential, it often requires the combined influence of multiple presynaptic inputs. While a single excitatory postsynaptic potential (EPSP) can be sufficient to trigger an action potential in some cases, it is more common for EPSPs to summate, or accumulate, at the axon hillock to reach the necessary threshold for firing. This process of signal summation allows the postsynaptic neuron to integrate inputs from various presynaptic neurons occurring simultaneously or in close succession.
- Signal summation takes place at the axon hillock, a region where the postsynaptic potentials are integrated. Multiple EPSPs generated by different presynaptic inputs can summate, leading to a cumulative depolarization of the postsynaptic membrane. This combined effect increases the likelihood of the postsynaptic neuron reaching its threshold of excitation and generating an action potential.
- It’s important to note that a postsynaptic neuron typically receives inputs from numerous presynaptic neurons, which can include both excitatory and inhibitory synapses. Inhibitory postsynaptic potentials (IPSPs) can counteract the depolarizing effects of EPSPs, and vice versa. In this way, IPSPs and EPSPs can interact and modulate the net change in postsynaptic membrane voltage. The overall balance between excitatory and inhibitory inputs determines whether the postsynaptic cell reaches the threshold for excitation required to initiate an action potential.
- Signal summation and the threshold for excitation together function as a filtering mechanism, ensuring that only relevant and significant information is transmitted. By integrating multiple inputs and considering the net change in membrane voltage, the postsynaptic neuron can differentiate meaningful signals from random background noise, enhancing the precision and selectivity of neural communication.
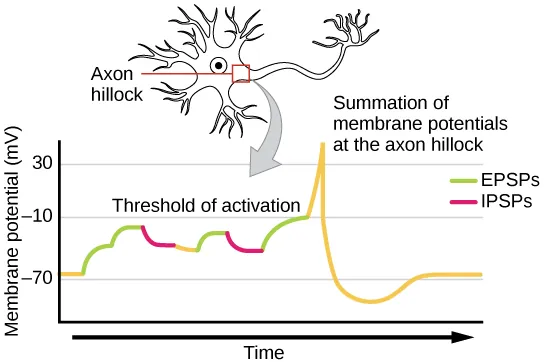
Synaptic Plasticity
Synapses, the junctions between neurons, are not fixed entities but rather highly dynamic structures capable of undergoing changes in their strength and connectivity. This ability of synapses to be modified is known as synaptic plasticity, a fundamental process that underlies the functioning of the nervous system. Synaptic plasticity allows for the strengthening, weakening, formation, and elimination of synapses, all of which play crucial roles in learning, memory, and overall neural circuitry.
Long-term potentiation (LTP) and long-term depression (LTD) are two essential forms of synaptic plasticity that occur within the hippocampus, a region of the brain crucial for memory storage. LTP is characterized by the long-lasting strengthening of synaptic connections, resulting in increased synaptic efficacy. When a synapse undergoes LTP, it becomes more responsive to presynaptic input, and the postsynaptic neuron becomes more likely to generate an action potential in response to stimulation. This enhanced synaptic transmission is believed to be a cellular mechanism underlying the processes of learning and memory formation.
On the other hand, LTD involves the long-lasting weakening of synaptic connections. It is characterized by a decrease in synaptic strength, leading to a reduced postsynaptic response to presynaptic input. LTD plays a crucial role in synaptic pruning and refining neural circuits by eliminating unnecessary or weak connections. This process is vital for sculpting neural networks during development and allowing for more efficient information processing.
The mechanisms underlying synaptic plasticity, including LTP and LTD, are complex and involve intricate cellular processes. They often rely on changes in the strength and number of neurotransmitter receptors, alterations in synaptic vesicle release and recycling, and modifications in the structure and function of dendritic spines—the tiny protrusions on the postsynaptic neuron where most excitatory synapses occur.
Synaptic plasticity is not limited to the hippocampus but occurs throughout the brain in various regions and neural circuits. It is a dynamic and ongoing process that allows the nervous system to adapt, learn, and store information based on experiences and environmental changes. The ability of synapses to undergo plastic changes provides the basis for our capacity to acquire new knowledge, form memories, and continually adapt our behaviors and responses.
Long-term Potentiation (LTP)
- Long-term potentiation (LTP) is a phenomenon characterized by the persistent strengthening of a synaptic connection between neurons. It plays a fundamental role in synaptic plasticity, learning, and memory formation. LTP is guided by the Hebbian principle, which states that cells that fire together wire together, implying that synapses are strengthened when they are repeatedly and synchronously activated.
- The mechanisms underlying LTP are complex and not fully understood, but one well-known mechanism involves the activation of a specific type of glutamate receptor known as the NMDA (N-Methyl-D-aspartate) receptor. Normally, these receptors are blocked by magnesium ions, preventing the influx of calcium ions into the postsynaptic neuron. However, when the postsynaptic neuron is depolarized due to the simultaneous and repetitive activation of presynaptic inputs, the depolarization drives the magnesium ions out of the NMDA receptors, allowing calcium ions to enter the postsynaptic cell.
- The entry of calcium ions triggers a cascade of signaling events within the postsynaptic neuron, leading to the insertion of another type of glutamate receptor called AMPA (α-amino-3-hydroxy-5-methyl-4-isoxazolepropionic acid) receptors into the postsynaptic membrane. The activation of AMPA receptors allows the influx of positive ions into the postsynaptic cell upon binding to glutamate. As a result, subsequent release of glutamate from the presynaptic terminal leads to larger excitatory postsynaptic potentials (EPSPs) in the postsynaptic neuron.
- The insertion of additional AMPA receptors and the strengthening of the synapse increase the efficiency of synaptic transmission. The postsynaptic neuron becomes more responsive to presynaptic input, making it more likely to generate an action potential in response to neurotransmitter release. This synaptic strengthening and enhanced synaptic efficacy are believed to be crucial for the encoding and storage of information in the brain.
- It is important to note that LTP is not limited to a single synapse but can occur in networks of interconnected neurons, contributing to the formation of functional circuits. The persistence of LTP can last for extended periods, allowing the strengthened connections to persist and contribute to long-term changes in neural activity.
- Understanding the mechanisms of LTP has significant implications beyond basic neuroscience. Drugs of abuse, for instance, can exploit the LTP pathway, leading to the strengthening of certain synapses associated with reward and addiction. This synaptic strengthening can contribute to the development of addictive behaviors.
- Although many aspects of LTP remain to be elucidated, its role in synaptic plasticity and its link to learning and memory highlight its importance in shaping the functionality of the nervous system and our ability to acquire and retain information.
Long-term Depression (LTD)
- Long-term depression (LTD) is a process that involves the long-term weakening of a synaptic connection between neurons. It is considered the opposite of long-term potentiation (LTP) and serves as a crucial mechanism for synaptic plasticity and the refinement of neural circuits.
- One known mechanism underlying LTD involves the modulation of AMPA (α-amino-3-hydroxy-5-methyl-4-isoxazolepropionic acid) receptors. Similar to LTP, LTD also involves the activation of NMDA (N-Methyl-D-aspartate) receptors by presynaptic release of glutamate, leading to calcium ion influx into the postsynaptic neuron. However, the subsequent signaling cascade triggered by calcium entry differs from that of LTP.
- In the case of LTD, the signaling cascade initiated by calcium influx through NMDA receptors results in the removal of AMPA receptors from the postsynaptic membrane. This reduction in the number of AMPA receptors decreases the responsiveness of the postsynaptic neuron to glutamate released by the presynaptic neuron. Consequently, the synaptic strength weakens, and the transmission efficiency decreases.
- Although it may initially appear counterintuitive, LTD is believed to play a crucial role in learning and memory processes. The weakening and pruning of unused or less important synaptic connections through LTD allow for the elimination of unnecessary information and the refinement of neural networks. By selectively weakening synapses, LTD helps to sharpen the specificity and precision of synaptic transmission, enabling the retention of essential information while eliminating noise or irrelevant connections.
- The balance between LTD and LTP is essential for synaptic plasticity and the optimization of neural circuits. While LTP strengthens synapses and promotes the formation of memory traces, LTD ensures the removal of weak or unimportant connections, preventing the saturation of the neuronal network. The interplay between LTD and LTP allows for the establishment of efficient and adaptive neural connections, facilitating learning, memory storage, and cognitive processes.
- Overall, the process of LTD demonstrates that synaptic plasticity involves not only the strengthening but also the weakening of synapses. By selectively weakening connections, LTD contributes to the optimization and refinement of neural circuits, promoting efficient information processing in the brain.
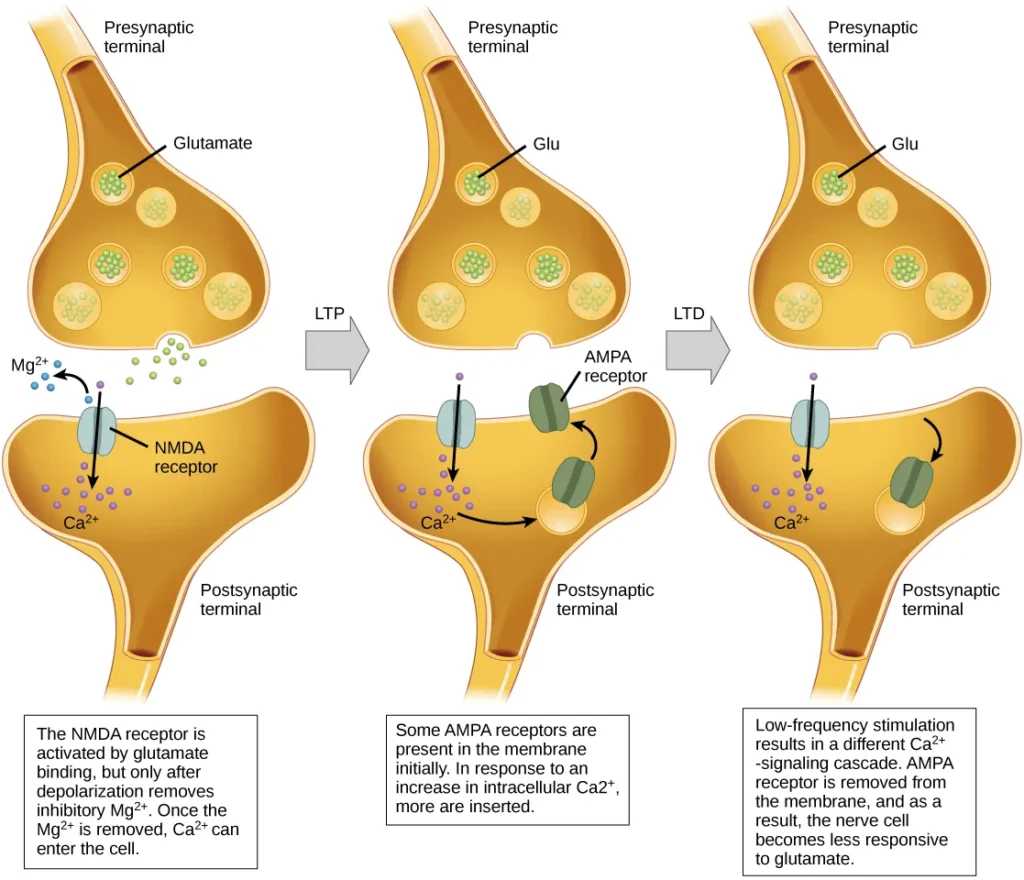