What is Beta-oxidation?
- Beta-oxidation is a fundamental process in biochemistry and metabolism that plays a crucial role in breaking down fatty acid molecules to generate energy. It involves a series of enzymatic reactions that occur in the cytosol of prokaryotes or the mitochondria of eukaryotes. The end products of beta-oxidation are acetyl-CoA, which enters the citric acid cycle, and NADH and FADH2, which serve as co-enzymes in the electron transport chain.
- The name “beta-oxidation” stems from the fact that the beta carbon of the fatty acid undergoes oxidation, resulting in the formation of a carbonyl group. This process is primarily facilitated by the mitochondrial trifunctional protein, an enzyme complex associated with the inner mitochondrial membrane. However, very long chain fatty acids are oxidized in peroxisomes.
- The overall reaction for one cycle of beta-oxidation involves the conversion of a Cn-acyl-CoA molecule, along with FAD, NAD+, water, and CoA, into a Cn-2-acyl-CoA molecule, FADH2, NADH, H+, and acetyl-CoA. This cycle continues iteratively until the acyl-CoA chain has been completely broken down, and two acetyl-CoA molecules are produced.
- To initiate beta-oxidation, fatty acids need to first enter the cell by traversing the cell membrane. Once inside, they bind to coenzyme A (CoA), forming fatty acyl CoA. In the case of eukaryotic cells, these fatty acyl CoA molecules then enter the mitochondria, where the beta-oxidation process takes place. In prokaryotic cells, beta-oxidation occurs in the cytosol.
- During beta-oxidation, long fatty acyl-CoA chains are progressively broken down into smaller acyl-CoA chains. This breakdown releases acetyl-CoA, FADH2, and NADH. These compounds subsequently enter the citric acid cycle or Krebs cycle, where further energy production occurs through ATP synthesis.
- Overall, beta-oxidation is a vital metabolic pathway that allows organisms to utilize fatty acids as a source of energy. It plays a significant role in lipid metabolism and is essential for maintaining energy balance within cells. Understanding the mechanisms and regulation of beta-oxidation contributes to our knowledge of metabolic disorders and provides insights into therapeutic approaches aimed at modulating lipid metabolism.
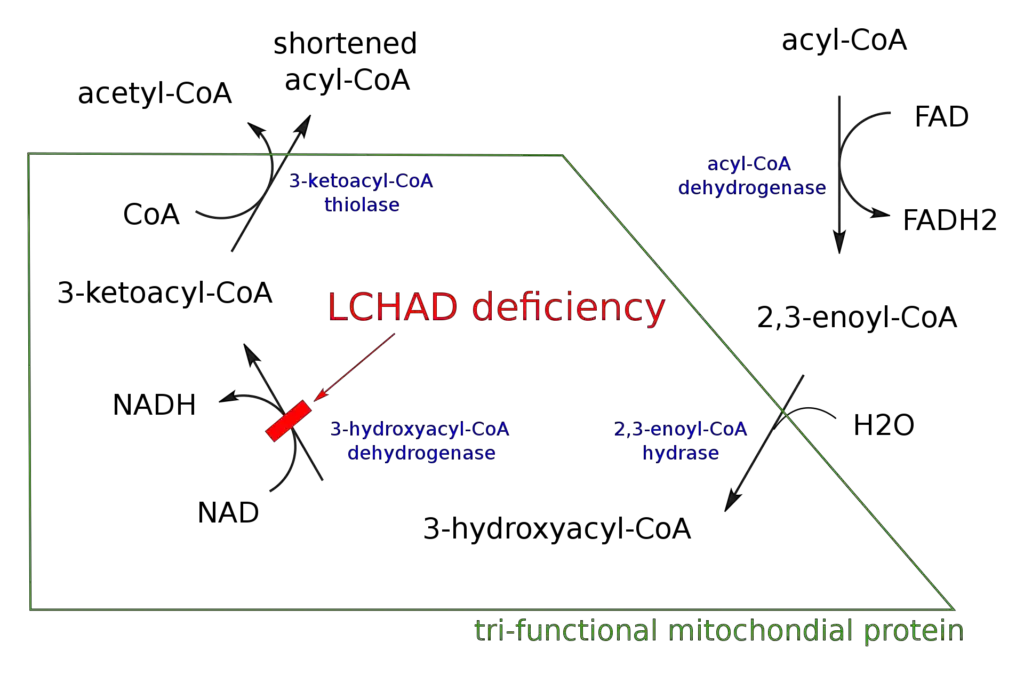
Definition of Beta-oxidation
Beta-oxidation is the metabolic process in cells where fatty acids are broken down into smaller units called acetyl-CoA, generating energy in the form of ATP.
Location of Beta-Oxidation
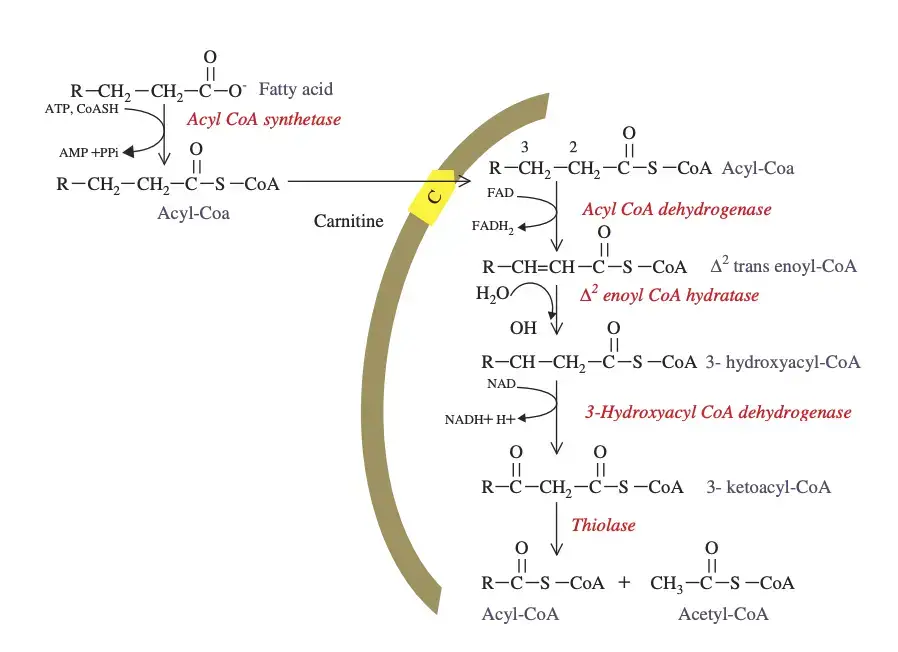
Beta-oxidation, a crucial metabolic pathway for the breakdown of fatty acids, occurs in specific cellular locations depending on the organism’s type. In eukaryotes, this process takes place primarily in the mitochondria, while in prokaryotes, it occurs in the cytosol. However, in certain cases, when fatty acid chains are too long, beta-oxidation can also occur in peroxisomes.
To initiate beta-oxidation, fatty acids must first traverse the cell membrane and, in the case of eukaryotes, reach the mitochondria. Fatty acid protein transporters facilitate the entry of fatty acids into the cytosol. Since fatty acid chains carry a negative charge, these transporters are necessary because they cannot cross the cell membrane unaided. Once inside the cytosol, the enzyme fatty acyl-CoA synthase (FACS) adds a CoA group to the fatty acid chain, converting it to acyl-CoA.
Depending on the length of the acyl-CoA chain, it will enter the mitochondria using one of two mechanisms:
- Short-chain acyl-CoA molecules can freely diffuse through the mitochondrial membrane and enter the mitochondrial matrix where beta-oxidation occurs.
- Long-chain acyl-CoA molecules require the carnitine shuttle to transport them across the mitochondrial membrane. The enzyme carnitine palmitoyltransferase 1 (CPT1), bound to the outer mitochondrial membrane, catalyzes the conversion of the acyl-CoA chain to an acylcarnitine chain. This acylcarnitine chain can then be transported across the mitochondrial membrane by carnitine translocase (CAT). Once inside the mitochondria, another enzyme called carnitine palmitoyltransferase 2 (CPT2), bound to the inner mitochondrial membrane, converts the acylcarnitine back to acyl-CoA. At this stage, acyl-CoA is within the mitochondria and is ready to undergo beta-oxidation.
It is important to note that in situations where the acyl-CoA chain is too long to be processed within the mitochondria, it is broken down by beta-oxidation in the peroxisomes. Recent research suggests that very long acyl-CoA chains are broken down until they reach a length of eight carbons. Subsequently, they are transported and enter the beta-oxidation cycle within the mitochondria. In the peroxisomes, beta-oxidation yields hydrogen peroxide (H2O2) instead of FADH2 and NADH, leading to the generation of heat as a byproduct.
In summary, beta-oxidation occurs primarily in the mitochondria of eukaryotic cells and in the cytosol of prokaryotic cells. Fatty acids are transported into the cell and, if necessary, into the mitochondria using various mechanisms. The breakdown of fatty acids through beta-oxidation generates acetyl CoA, NADH, and FADH2, which are essential energy sources for cellular processes.
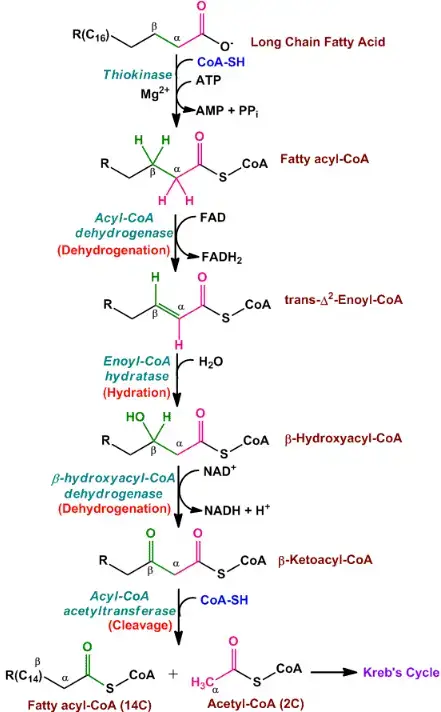
Steps of Beta-Oxidation
Beta-oxidation is a vital metabolic pathway responsible for the breakdown of fatty acids in organisms. This process occurs within the mitochondria of eukaryotic cells and the cytosol of prokaryotic cells. Let’s explore the steps involved in beta-oxidation.
- Activation: The first step of beta-oxidation occurs in the cytosol for both eukaryotes and prokaryotes. Fatty acids are activated by the enzyme fatty acyl-CoA synthase (FACS), which adds a CoA group to the fatty acid chain, converting it to acyl-CoA. This activation allows the fatty acid to undergo further processing.
- Entry into the Mitochondria: In eukaryotes, long-chain acyl-CoA molecules need to enter the mitochondria to undergo beta-oxidation. The acyl-CoA chain is converted to an acylcarnitine chain by the enzyme carnitine palmitoyltransferase 1 (CPT1) located on the outer mitochondrial membrane. The acylcarnitine chain is transported across the mitochondrial membrane by carnitine translocase (CAT). Inside the mitochondria, carnitine palmitoyltransferase 2 (CPT2) converts the acylcarnitine back to acyl-CoA.
- Beta-Oxidation Cycle: Once inside the mitochondria, beta-oxidation begins. The acyl-CoA chain undergoes a series of four repeated reactions until it is fully oxidized. Each cycle removes a two-carbon group from the fatty acid chain in the form of acetyl CoA. The reactions involved in each cycle are:a. Oxidation: An acyl-CoA dehydrogenase enzyme catalyzes the removal of two hydrogen atoms from the beta-carbon of the acyl-CoA chain. This creates a trans-double bond between the alpha- and beta-carbons and forms a double bond with FAD, resulting in the production of FADH2.b. Hydration: The trans-double bond formed in the previous step is converted to a hydroxyl group (-OH) through the addition of a water molecule. This is catalyzed by an enoyl-CoA hydratase enzyme.c. Oxidation: A second oxidation step occurs, where another acyl-CoA dehydrogenase enzyme removes two hydrogen atoms from the beta-carbon, forming a beta-ketoacyl-CoA compound. NAD+ is reduced to NADH during this process.d. Thiolysis: The beta-ketoacyl-CoA compound is cleaved by a thiolase enzyme, breaking it into an acetyl CoA molecule and a shortened acyl-CoA chain, two carbons shorter than the original chain.
- Citric Acid Cycle and Electron Transport Chain: The acetyl CoA molecules produced during beta-oxidation enter the citric acid cycle (also known as the Krebs cycle or TCA cycle), where they undergo further oxidation to produce NADH and FADH2. These electron carriers enter the electron transport chain, generating ATP through oxidative phosphorylation. Each acetyl CoA molecule entering the citric acid cycle can produce around 12 ATP.
- Propionyl CoA Conversion: In some cases, if the fatty acid chain contains an odd number of carbons, the last cycle of beta-oxidation results in the production of propionyl CoA instead of acetyl CoA. Propionyl CoA can be further converted to succinyl CoA through a series of three enzymatic reactions that require biotin and vitamin B12 as cofactors. Succinyl CoA then enters the citric acid cycle to generate additional ATP.
The cycle of beta-oxidation repeats until the entire long-chain fatty acid is converted into acetyl CoA or propionyl CoA, depending on the chain length and composition. This process allows organisms to utilize fatty acids as an energy source, contributing to ATP production and supporting various cellular functions.
1. Activation of fatty acids
The activation of fatty acids is an essential step in the metabolism of long-chain fatty acids. It takes place in the cytosol of the cell for long-chain fatty acids and in the mitochondria for short-chain fatty acids. Let’s delve into the process of fatty acid activation.
- Long-Chain Fatty Acid Activation: In the cytosol, long-chain fatty acids undergo activation through a series of reactions involving ATP and coenzyme A (CoA). The process begins with the fatty acid reacting with ATP and forming a complex with AMP (adenosine monophosphate) and pyrophosphate (PPi). This reaction is catalyzed by an enzyme called acyl-CoA synthetase or fatty acyl-CoA synthetase.
- Formation of Fatty Acyl-CoA: The activated complex from the previous step interacts with coenzyme A (CoA), leading to the formation of fatty acyl-CoA. Coenzyme A acts as a carrier molecule, attaching to the fatty acid chain and facilitating its transportation and utilization within cellular processes. As a result of this reaction, two inorganic phosphates (2 Pi) are released.
- Cleavage of Pyrophosphate: The formation of fatty acyl-CoA from ATP and CoA involves the cleavage of pyrophosphate (PPi). The released pyrophosphate is then further cleaved by the enzyme pyrophosphatase, resulting in the production of two inorganic phosphate molecules (2 Pi). This step is crucial because it allows the energy stored in the high-energy phosphate bonds of ATP to be utilized for fatty acid activation.
The activation of long-chain fatty acids requires the expenditure of energy in the form of ATP. The cleavage of two high-energy phosphate bonds during the formation of fatty acyl-CoA represents the equivalent of two molecules of ATP being consumed. This ATP investment is necessary to activate the fatty acids and prepare them for subsequent metabolic processes such as beta-oxidation.
In contrast, short-chain fatty acids are activated within the mitochondria. The specific details of short-chain fatty acid activation involve their transportation into the mitochondria, where they undergo a similar process of activation with CoA.
2. Transport of fatty acyl-CoA from the cytosol into mitochondria
The transport of fatty acyl-CoA from the cytosol into the mitochondria is a crucial step in the metabolism of fatty acids. Let’s explore the process involved in this transportation:
- Formation of Fatty Acylcarnitine: In the outer mitochondrial membrane, fatty acyl-CoA reacts with carnitine to form fatty acylcarnitine. This conversion is catalyzed by an enzyme known as carnitine acyltransferase I (CAT I), which is also referred to as carnitine palmitoyltransferase I (CPT I). This reaction occurs on the outer side of the mitochondrial membrane.
- Transfer of Fatty Acylcarnitine: The formed fatty acylcarnitine is then transported across the inner mitochondrial membrane. It passes through a carnitine-acylcarnitine translocase or carnitine transporter system, which facilitates its movement into the mitochondrial matrix.
- Reformation of Fatty Acyl-CoA: Once inside the mitochondrial matrix, fatty acylcarnitine is converted back into fatty acyl-CoA. This reconversion is catalyzed by an enzyme called carnitine acyltransferase II (CAT II). The fatty acyl-CoA is now in its active form and ready to undergo beta-oxidation.
It is important to note that the activity of carnitine acyltransferase I (CPT I) is regulated by the presence of malonyl-CoA, an intermediate molecule in fatty acid synthesis. When fatty acids are being synthesized in the cytosol, malonyl-CoA levels increase. Malonyl-CoA acts as an inhibitor of CPT I, preventing the transport of fatty acyl-CoA into the mitochondria. This mechanism ensures that fatty acids are not simultaneously synthesized and degraded, preventing a futile cycle.
Once inside the mitochondria, fatty acyl-CoA can undergo beta-oxidation. This process involves a series of enzymatic reactions that result in the gradual breakdown of fatty acids, ultimately producing acetyl CoA units, NADH, and FADH2. These energy-rich products further participate in the citric acid cycle and the electron transport chain, leading to ATP generation and providing energy for cellular processes.
In summary, the transport of fatty acyl-CoA from the cytosol into the mitochondria involves the formation of fatty acylcarnitine in the outer mitochondrial membrane, facilitated by CAT I. Fatty acylcarnitine then moves across the inner mitochondrial membrane through a carnitine transporter system. Inside the mitochondrial matrix, CAT II converts fatty acylcarnitine back into fatty acyl-CoA, allowing it to enter beta-oxidation and participate in energy production. The regulation of CPT I by malonyl-CoA prevents simultaneous fatty acid synthesis and degradation.
3. β-Oxidation of even-chain fatty acids
The β-oxidation of even-chain fatty acids is a cyclic process that occurs in the mitochondria, involving a series of sequential steps. This pathway breaks down the fatty acyl-CoA molecule and generates acetyl-CoA units, which can further enter the citric acid cycle to produce energy. Let’s explore the steps involved in β-oxidation of even-chain fatty acids:
- Step 1: Oxidation by Acyl-CoA Dehydrogenase: The first step involves the oxidation of the fatty acyl-CoA molecule. Acyl-CoA dehydrogenase, which exists in multiple variants, facilitates this reaction. During oxidation, FAD (flavin adenine dinucleotide) accepts hydrogens from the fatty acyl-CoA, resulting in the formation of a double bond between the α- and β-carbons of the molecule. This process generates FADH2, which can enter the electron transport chain and contribute to ATP production.
- Step 2: Hydration by Enoyl-CoA Hydratase: In the second step, water (H2O) adds across the double bond created in the previous step. This addition of water leads to the formation of a β-hydroxyacyl-CoA molecule. Enoyl-CoA hydratase is the enzyme responsible for catalyzing this hydration reaction.
- Step 3: Oxidation by L-3-Hydroxyacyl-CoA Dehydrogenase: The β-hydroxyacyl-CoA molecule generated in step 2 undergoes oxidation in the third step. NAD+ (nicotinamide adenine dinucleotide) acts as an electron acceptor, oxidizing the β-hydroxyacyl-CoA and converting it into a β-ketoacyl-CoA molecule. This oxidation reaction results in the production of NADH, which can participate in the electron transport chain to generate ATP. L-3-hydroxyacyl-CoA dehydrogenase is the enzyme responsible for this oxidation step and is specific to the L-isomer of β-hydroxyacyl-CoA.
- Step 4: Cleavage by β-Ketothiolase: In the final step of each cycle, the bond between the alpha and beta carbons of the β-ketoacyl-CoA molecule is cleaved by the enzyme β-ketothiolase. Coenzyme A (CoA) participates in this reaction. The result is the production of acetyl-CoA from the two carbons located at the carboxyl end of the original fatty acyl-CoA molecule. The remaining carbons form a shortened fatty acyl-CoA molecule that is two carbons shorter than the original one.
The shortened fatty acyl-CoA molecule then goes through the same four-step process again, repeating the cycle until all the carbons of the original even-chain fatty acyl-CoA have been converted into acetyl-CoA units. These acetyl-CoA molecules can enter the citric acid cycle (also known as the Krebs cycle) to generate additional ATP through oxidative phosphorylation.
In summary, the β-oxidation of even-chain fatty acids involves a spiral pathway consisting of four sequential steps. These steps include oxidation, hydration, further oxidation, and cleavage. Through repeated cycles of these steps, the fatty acyl-CoA molecule is gradually broken down, resulting in the production of acetyl-CoA units and generating energy for cellular processes.
Energy Yield for Even-chain Fatty Acids
The energy yield for even-chain fatty acids during β-oxidation is significant, providing a substantial amount of ATP for cellular energy needs. Let’s examine the energy yield for the oxidation of a 16-carbon palmitoyl-CoA molecule:
- Seven repetitions of β-Oxidation: The 16-carbon palmitoyl-CoA molecule undergoes seven repetitions of the β-oxidation cycle, resulting in the production of seven FADH2, seven NADH, and eight acetyl-CoA molecules.
- FADH2: Each FADH2 molecule generated from β-oxidation can generate approximately 1.5 ATP through the electron transport chain. Therefore, seven FADH2 molecules would yield around 10.5 ATP.
- NADH: Each NADH molecule produced during β-oxidation can generate about 2.5 ATP through the electron transport chain. With seven NADH molecules, the total ATP yield would be approximately 17.5 ATP.
- Acetyl-CoA: The eight acetyl-CoA molecules formed from the final cleavage of a 4-carbon fatty acyl-CoA (butyryl-CoA) can enter the citric acid cycle (TCA cycle) to produce ATP. Each acetyl-CoA molecule entering the TCA cycle can generate about 10 ATP. Therefore, eight acetyl-CoA would yield approximately 80 ATP.
- Total ATP production: Adding up the ATP yields from FADH2, NADH, and acetyl-CoA, the total ATP production from the oxidation of one palmitoyl-CoA molecule would be approximately 108 ATP (10.5 ATP + 17.5 ATP + 80 ATP).
- Net ATP yield: However, it’s important to consider that the activation of palmitate, the process that converts it to palmitoyl-CoA, requires the equivalent of 2 ATP. Therefore, the net ATP yield from the oxidation of palmitate that enters the cell from the blood is approximately 106 ATP (108 ATP – 2 ATP).
It’s worth noting that the oxidation of other fatty acids with different chain lengths will yield varying amounts of ATP since the number of acetyl-CoA units produced will depend on the length of the fatty acid chain.
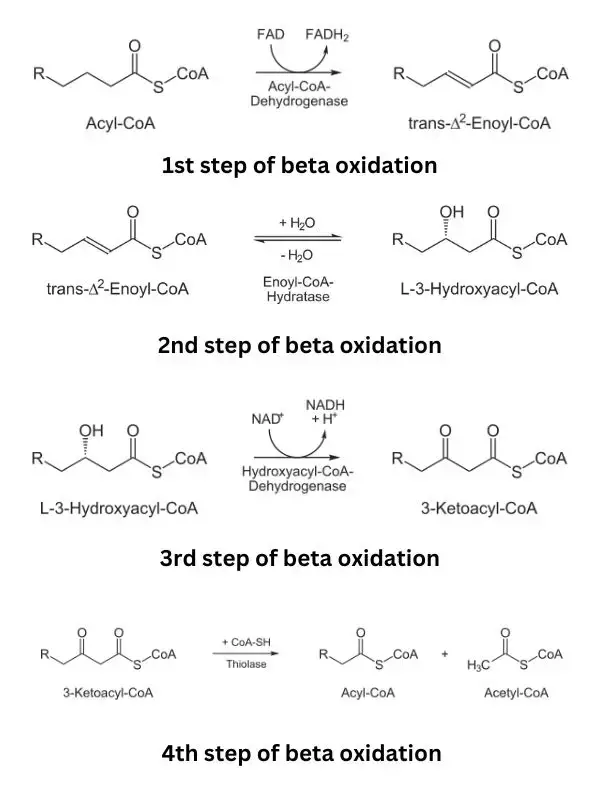
In summary, the β-oxidation of even-chain fatty acids, such as palmitate, generates a substantial amount of ATP. The process produces ATP through the generation of FADH2 and NADH, which enter the electron transport chain, as well as through the entry of acetyl-CoA into the citric acid cycle. The net ATP yield from the oxidation of palmitate is approximately 106 ATP after considering the energy expended during activation. The oxidation of other fatty acids will result in different ATP yields based on their chain lengths.
4. Oxidation of odd-chain and unsaturated fatty acids
The oxidation of odd-chain and unsaturated fatty acids involves some variations compared to the β-oxidation of even-chain fatty acids. Let’s explore these differences:
- Odd-chain fatty acids: Odd-chain fatty acids have an odd number of carbon atoms in their chain. During β-oxidation, they undergo the same four-step spiral as even-chain fatty acids, producing acetyl-CoA. However, in the last cleavage step, instead of two acetyl-CoA molecules, three carbons are released as propionyl-CoA. Propionyl-CoA can enter further metabolic pathways and be converted to glucose through a series of enzymatic reactions. This ability to contribute to gluconeogenesis sets odd-chain fatty acids apart from even-chain fatty acids.
- Unsaturated fatty acids: Unsaturated fatty acids, which contain one or more double bonds in their carbon chain, require additional enzymes to facilitate their oxidation. The reaction pathway depends on whether the double bond is at an even- or odd-numbered carbon position.
- Even-numbered double bond: If the double bond is located at an even carbon position (e.g., 4, 6, 8, etc.), a specific enzyme called 2,4-dienoyl-CoA reductase is involved. This enzyme utilizes NADPH and reduces the trans-Δ2, cis-Δ4 fatty acyl-CoA to produce a trans-Δ3-acyl-CoA and NADP+. An isomerase then converts the trans-Δ3 fatty acyl-CoA to a trans-Δ2 fatty acyl-CoA, allowing β-oxidation to proceed.
- Odd-numbered double bond: If the double bond is present at an odd carbon number (e.g., 3, 5, 7, etc.), an isomerase enzyme comes into play. It converts the cis-Δ3 fatty acyl-CoA produced during β-oxidation to a trans-Δ2 fatty acyl-CoA. This conversion enables the continuation of β-oxidation.
These additional enzymatic steps for unsaturated fatty acids with double bonds ensure that the oxidation process can proceed effectively and accommodate the presence of these chemical modifications in the fatty acid chain.
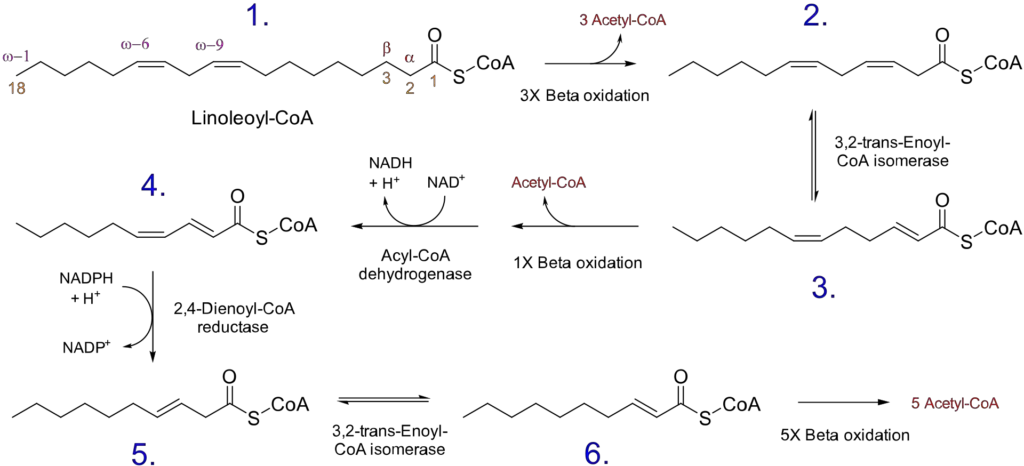
In summary, the oxidation of odd-chain fatty acids results in the production of acetyl-CoA and propionyl-CoA, with propionyl-CoA being capable of being converted to glucose. Unsaturated fatty acids require additional enzymes to handle the presence of double bonds, and the reaction pathway differs depending on whether the double bond is at an even or odd carbon position. These variations in the oxidation process accommodate the structural differences of odd-chain and unsaturated fatty acids, ensuring their efficient utilization for energy production and other metabolic pathways.
ATP yield
The ATP yield from beta-oxidation of fatty acids is an important aspect of energy generation in cells. The actual ATP yield varies depending on the specific fatty acid being oxidized, its length, saturation, and whether it is even or odd-numbered. Here are some key points regarding ATP yield in beta-oxidation:
- Even-Numbered Saturated Fatty Acids: For even-numbered saturated fatty acids (Cn), the ATP yield can be calculated using the formula 7n-6. This equation takes into account the number of oxidations required (0.5 * n – 1), the ATP produced from FADH2 and NADH, the ATP generated from acetyl-CoA in the citric acid cycle, and the ATP lost during fatty acid activation. As an example, the ATP yield for palmitate (C16) is calculated as 7 * 16 – 6, resulting in a total of 106 ATP.
- Odd-Numbered Saturated Fatty Acids: For odd-numbered saturated fatty acids (Cn), the ATP yield is slightly different due to the presence of propionyl-CoA, which is generated in the last step of beta-oxidation. In addition to the ATP yield from FADH2, NADH, and acetyl-CoA, the propionyl-CoA can be converted to succinyl-CoA, producing an additional 5 ATP (4 ATP net gain). The ATP yield for odd-numbered saturated fatty acids can be calculated using the formula 7n-19. For example, the ATP yield for margaric acid (C17) is 7 * 17 – 19, resulting in a total of 100 ATP.
- Unsaturated Fatty Acids: The ATP yield for unsaturated fatty acids may differ from saturated fatty acids due to the involvement of additional enzymes and reactions. The presence of double bonds in unsaturated fatty acids requires specific enzymes to isomerize or reduce the double bonds, which can affect the overall ATP production. The exact ATP yield for unsaturated fatty acids depends on the position and number of double bonds and the specific metabolic pathway involved.
It is important to note that the calculated ATP yields are theoretical maximum values, and in practice, the actual ATP yield may be slightly lower due to factors such as energy requirements for transport and enzyme activities. The ATP yield mentioned above provides an estimate of the energy generated during the process of beta-oxidation and serves as a general guideline for understanding the energy efficiency of fatty acid metabolism.
Overall, beta-oxidation of fatty acids is a highly efficient process for generating ATP and plays a vital role in meeting the energy demands of cells and tissues. The precise ATP yield depends on various factors related to the fatty acid structure, and understanding these yields helps in comprehending the energy balance and metabolic significance of beta-oxidation.
Overall Reaction of Beta oxidation
The overall reaction of one cycle of beta oxidation involves the stepwise oxidation of a fatty acyl-CoA molecule, resulting in the production of acetyl-CoA, reducing equivalents in the form of FADH2 and NADH, and a shortened fatty acyl-CoA chain. The reaction can be summarized as follows:
Cn-acyl CoA + FAD + NAD+ + H2O + CoA → Cn-2-acyl CoA + FADH2 + NADH + H+ + acetyl CoA
In this reaction, the fatty acyl-CoA with a chain length of “n” carbons reacts with FAD (flavin adenine dinucleotide) and NAD+ (nicotinamide adenine dinucleotide) as electron acceptors. Water (H2O) and CoA (coenzyme A) are also involved in the process.
As a result of the beta oxidation cycle, the fatty acyl-CoA chain is shortened by two carbon atoms, leading to the formation of Cn-2-acyl CoA. Additionally, FAD is reduced to FADH2, and NAD+ is reduced to NADH, generating reducing equivalents that can be used in the electron transport chain to produce ATP. One molecule of acetyl-CoA is also produced, which can enter the citric acid cycle (also known as the Krebs cycle) to further generate ATP through oxidative phosphorylation.
The overall reaction of beta oxidation is repeated iteratively until the entire fatty acyl-CoA molecule is converted into acetyl-CoA units. The number of cycles required depends on the initial length of the fatty acid chain. Each cycle produces acetyl-CoA, reducing equivalents (FADH2 and NADH), and a shorter fatty acyl-CoA chain, contributing to energy production and the gradual breakdown of the fatty acid molecule.
Tissues Involved in Beta-Oxidation
Beta-oxidation, a pivotal metabolic pathway, is instrumental in the catabolism of fatty acids to generate energy. This process is intricately regulated and is executed in distinct tissues based on the organism’s metabolic status and energy requisites. Herein, we delve into the specific tissues that actively participate in beta-oxidation and their respective roles.
- Liver: Recognized as a central hub for metabolic activities, the liver stands out as a primary site for fatty acid oxidation, especially during periods of fasting. Its role is indispensable in the liberation of fatty acids from triglyceride reserves in response to energy deficits. Furthermore, the liver is adept at synthesizing ketone bodies, which serve as an auxiliary energy substrate, especially for the brain during prolonged fasting.
- Skeletal Muscle: Skeletal muscle, comprising a significant portion of the body’s mass, is a vital locus for beta-oxidation. In scenarios of heightened energy demand, such as physical exertion, skeletal muscles preferentially resort to fatty acids to fulfill their ATP requirements, underscoring the importance of beta-oxidation in muscular function.
- Adipose Tissue: While primarily recognized as the body’s lipid reservoir, adipose tissue is not merely a passive storage site. In situations of caloric scarcity or extended fasting, it actively mobilizes its stored triglycerides through a process termed lipolysis. The liberated fatty acids are subsequently available for uptake and oxidation by energy-demanding tissues.
- Cardiac Muscle: The heart, with its incessant rhythmic contractions, has an unwavering energy demand. To meet this demand, cardiac muscle predominantly relies on fatty acid oxidation. Beta-oxidation in the heart ensures a steady supply of ATP, facilitating the perpetual contraction and relaxation cycles of the cardiac tissue.
Transport of Acyl-CoA into Mitochondria
The mitochondrial matrix serves as the primary site for beta-oxidation, a crucial metabolic pathway for fatty acid catabolism. However, for fatty acids to participate in this process, they must first be transported into the mitochondria. This transport is facilitated by a sophisticated system known as the carnitine shuttle. Here, we elucidate the sequential steps and key players involved in this transport mechanism:
- Fatty Acid Activation:
- Prior to mitochondrial entry, fatty acids undergo an activation process in the cellular cytoplasm. This involves their conversion to fatty acyl-CoA molecules.
- The enzyme responsible for this transformation is fatty acyl-CoA synthetase, which catalyzes the linkage of fatty acids with coenzyme A (CoA), resulting in the formation of fatty acyl-CoA.
- Acylcarnitine Synthesis:
- The mitochondrial inner membrane poses a barrier to the direct entry of fatty acyl-CoA due to its size and charge.
- To circumvent this, fatty acyl-CoA undergoes a conversion to acylcarnitine. This reaction is orchestrated by the enzyme carnitine palmitoyltransferase I (CPT-I), situated on the outer mitochondrial membrane.
- During this conversion, the acyl group of the fatty acyl-CoA is transferred to carnitine, yielding acylcarnitine and concurrently releasing free CoA.
- Acylcarnitine Translocation:
- The acylcarnitine molecule, being more amenable to transport, traverses the mitochondrial inner membrane via the carnitine-acylcarnitine translocase (CACT).
- This translocase not only facilitates the entry of acylcarnitine into the mitochondrial matrix but also concurrently exports free carnitine to the cytoplasm. This bidirectional transport ensures a consistent availability of carnitine for subsequent acylcarnitine synthesis.
- Reconversion to Acyl-CoA:
- Within the mitochondrial matrix, acylcarnitine is reverted to its precursor, acyl-CoA. This reaction is mediated by carnitine palmitoyltransferase II (CPT-II), an enzyme anchored to the inner mitochondrial membrane.
- The acyl group of acylcarnitine is transferred back to CoA, regenerating acyl-CoA. Concurrently, the liberated carnitine is shuttled back to the cytoplasm, readying it for another cycle of transport.
The carnitine shuttle system is paramount for the efficient delivery of fatty acids to the mitochondrial matrix, enabling their subsequent beta-oxidation. This intricate transport mechanism, especially the enzymatic activity of CPT-I, is under stringent regulatory control. Factors such as hormonal cues and the cell’s metabolic status influence its operation, ensuring optimal fatty acid utilization and maintaining metabolic equilibrium within the organism.
Important Enzymes
Several important enzymes are involved in the process of beta oxidation. These enzymes play crucial roles in catalyzing specific reactions that facilitate the breakdown of fatty acids. Here are some of the key enzymes involved:
- Acyl CoA dehydrogenase: This enzyme initiates beta oxidation by catalyzing the formation of a double bond between the α and β carbon atoms in the fatty acid chain. This step is essential for subsequent reactions. Acyl CoA dehydrogenase also produces one molecule of FADH2 as a byproduct.
- Enoyl CoA hydratase: Once the double bond is formed, enoyl CoA hydratase comes into play. It incorporates a water molecule into the fatty acid chain, leading to the hydration of the double bond. This step breaks the bond between the α and β carbon atoms.
- 3-Hydroxy-acyl CoA dehydrogenase: Following hydration, the fatty acid chain undergoes dehydrogenation again, facilitated by 3-hydroxy-acyl CoA dehydrogenase. This enzyme removes hydrogen from the β carbon and the hydroxyl group, resulting in the formation of a double bond between the β carbon and an oxygen molecule. During this process, one molecule of NADPH is generated.
- Acyl CoA acyltransferase: The final step of each beta oxidation cycle involves acyl CoA acyltransferase. This enzyme cleaves an acetyl CoA molecule from the end of the fatty acid chain, simultaneously attaching a CoA molecule to the β carbon. This reaction results in the release of acetyl CoA and the formation of a shortened fatty acyl CoA chain.
These enzymes work together in a coordinated manner to repetitively break down the fatty acid chain, generating acetyl CoA units, reducing equivalents (such as FADH2 and NADPH), and shorter fatty acyl CoA molecules. This iterative process continues until the entire fatty acid is converted into acetyl CoA, which can enter the citric acid cycle for further energy production. The proper functioning of these enzymes is crucial for efficient beta oxidation and energy metabolism.
Regulation of Beta-oxidation of Fatty Acids
Beta-oxidation, a central metabolic pathway for fatty acid catabolism, is intricately regulated to ensure the harmonious balance between energy storage and expenditure within the organism. This regulation is achieved through a multifaceted interplay of hormones, rate-limiting enzymes, and essential cofactors. Here, we elucidate the primary regulatory mechanisms that modulate beta-oxidation:
- Hormonal Regulation:
- Hormonal cues play a pivotal role in modulating beta-oxidation based on the organism’s metabolic state. During heightened energy requirements, hormones such as glucagon and adrenaline act as stimulants, augmenting the rate of beta-oxidation.
- Conversely, insulin, released postprandially, acts as an inhibitor, dampening beta-oxidation to favor energy storage.
- Role of Carnitine in Fatty Acid Transport:
- The translocation of long-chain fatty acyl-CoA molecules into the mitochondrial matrix is contingent upon the molecule carnitine. The availability of carnitine is thus a determining factor for the rate of beta-oxidation.
- Regulation by Carnitine Palmitoyltransferase I (CPT-I):
- CPT-I, a rate-limiting enzyme, governs the ingress of fatty acids into the mitochondria. Its activity is modulated by the relative concentration of malonyl-CoA.
- Elevated levels of malonyl-CoA, indicative of a state favoring energy storage, inhibit CPT-I. This results in a concomitant reduction in the transport of fatty acids into the mitochondria, thereby attenuating beta-oxidation.
- In contrast, scenarios such as fasting or physical exertion lead to diminished malonyl-CoA levels, thereby enhancing CPT-I activity and consequently amplifying beta-oxidation.
- Influence of Cofactors and Substrates:
- The enzymatic machinery orchestrating beta-oxidation is reliant on the presence of specific cofactors, including NAD+, FAD, and CoA.
- The availability of these cofactors is paramount for the optimal progression of the beta-oxidation pathway. Their adequate concentrations ensure the seamless and efficient catabolism of fatty acids.
In summation, the regulation of beta-oxidation is a sophisticated process, harmonized by a myriad of factors that respond to the organism’s metabolic cues. This ensures that energy production via beta-oxidation is tailored to meet the specific demands of the organism, thereby maintaining metabolic equilibrium.
Role of Fatty Acids in Regulation of Beta-Oxidation
Fatty acid beta-oxidation, a cornerstone of lipid metabolism, is intricately modulated by the availability and type of fatty acids. The supply of these fatty acids is contingent upon multiple factors, encompassing dietary sources, endogenous synthesis, and mobilization from adipose reserves. Here, we delineate the multifaceted role of fatty acids in the regulation of beta-oxidation:
- Dietary Origins:
- Dietary lipids serve as a primary exogenous reservoir of fatty acids. The nature of these fatty acids, specifically their saturation status and chain length, can modulate beta-oxidation rates. For instance, saturated fatty acids, devoid of double bonds, undergo oxidation more efficiently than their unsaturated counterparts. Furthermore, fatty acids with shorter carbon chains are preferentially oxidized over longer-chain variants.
- Mobilization from Adipose Deposits:
- Adipose tissue, the body’s lipid storehouse, becomes a pivotal source of fatty acids during caloric deficits or fasting states. Hormonal cues, notably glucagon and adrenaline, instigate the lipolytic release of fatty acids from adipose triglycerides. Enzymes like hormone-sensitive lipase (HSL) and adipose triglyceride lipase (ATGL) orchestrate this lipolytic cascade. The liberated fatty acids subsequently serve as substrates for beta-oxidation in peripheral tissues.
- De Novo Lipogenesis:
- Beyond exogenous sources and adipose mobilization, fatty acids can also be synthesized endogenously via de novo lipogenesis (DNL). Predominantly occurring in the liver, DNL is modulated by both nutritional and hormonal stimuli. Excess dietary carbohydrates, when metabolized, yield acetyl-CoA, a precursor for fatty acid synthesis. Depending on the body’s metabolic state, these newly synthesized fatty acids can either be stored or channeled into the beta-oxidation pathway.
- Hormonal Modulation:
- Hormonal interplay significantly influences fatty acid availability and subsequent beta-oxidation. Insulin, secreted postprandially, curtails fatty acid release by inhibiting adipose lipolysis. Conversely, hormones like glucagon and adrenaline, predominant during energy-depleted states, amplify lipolysis, bolstering fatty acid availability for oxidation.
- Feedback by Metabolic Intermediates:
- The beta-oxidation pathway is also subject to feedback regulation by its metabolic intermediates. Malonyl-CoA, an intermediate in fatty acid synthesis, acts as an inhibitor, precluding fatty acid entry into the mitochondria for oxidation. This feedback mechanism ensures that when energy requirements are satiated, fatty acids are directed towards storage. However, during energy-deprived states, a decline in malonyl-CoA levels alleviates this inhibition, favoring mitochondrial fatty acid uptake and oxidation.
In summation, the regulation of beta-oxidation by fatty acids is a testament to the body’s metabolic adaptability. Ensuring a harmonious balance between energy production and storage, this regulation is pivotal for metabolic homeostasis. Disruptions in these regulatory mechanisms can precipitate metabolic pathologies, underscoring the importance of understanding the intricate interplay between fatty acids and beta-oxidation.
Significance of Beta-oxidation of Fatty Acids
Beta-oxidation stands as a cornerstone in the metabolic framework, playing an indispensable role in energy production and metabolic homeostasis. This process is integral to the body’s adaptive mechanisms, especially during conditions of glucose scarcity. Here, we elucidate the multifaceted significance of beta-oxidation:
- Energy Production:
- Beta-oxidation is paramount during scenarios of diminished glucose availability, such as prolonged fasting or intense physical exertion. Under these conditions, the body predominantly relies on fatty acids as its energy substrate.
- The catabolism of fatty acids via beta-oxidation yields acetyl-CoA. This molecule subsequently enters the citric acid cycle, leading to the generation of reducing equivalents, namely NADH and FADH2.
- These reducing equivalents are channeled into the electron transport chain, culminating in the synthesis of ATP through oxidative phosphorylation. Thus, beta-oxidation is a major contributor to the body’s ATP reservoir, underscoring its role in energy sustenance.
- Metabolic Disorders and Beta-Oxidation:
- The enzymatic machinery of beta-oxidation is not infallible. Mutations or defects in enzymes associated with this pathway can give rise to fatty acid oxidation disorders (FAODs).
- FAODs are characterized by the accumulation of fatty acyl-CoA intermediates, stemming from an impaired breakdown of fatty acids. This accumulation can manifest in a spectrum of clinical symptoms, ranging from hypoglycemia and hepatic dysfunction to muscular debility and cardiomyopathies.
- Implications in Metabolic Syndrome and Obesity:
- Beyond rare metabolic disorders, beta-oxidation has garnered attention in the context of prevalent metabolic ailments, notably metabolic syndrome and obesity.
- Dysregulated fatty acid metabolism, coupled with defective beta-oxidation, can lead to an aberrant accumulation of fatty acids in non-adipose tissues, such as the liver and skeletal muscles.
- This ectopic fat deposition is implicated in a cascade of metabolic derangements, including insulin resistance, chronic inflammation, and the onset of associated metabolic diseases.
In conclusion, beta-oxidation is not merely a metabolic pathway but a linchpin in the body’s energy dynamics and metabolic health. Its seamless operation ensures energy equilibrium, while its dysregulation can precipitate a gamut of metabolic pathologies. Understanding the nuances of beta-oxidation offers insights into the intricate tapestry of energy metabolism and provides avenues for therapeutic interventions in metabolic disorders.
Quiz
FAQ
What is beta-oxidation of fatty acids?
Beta-oxidation is the metabolic process that breaks down fatty acids into acetyl-CoA molecules, which can be further utilized for energy production.
Where does beta-oxidation of fatty acids occur?
Beta-oxidation primarily occurs in the mitochondria of cells, where fatty acids are metabolized to generate ATP.
What are the products of beta-oxidation?
The main products of beta-oxidation are acetyl-CoA, which enters the citric acid cycle (also known as the Krebs cycle), and reduced electron carriers such as NADH and FADH2.
What is the role of beta-oxidation in energy production?
Beta-oxidation plays a crucial role in energy production by breaking down fatty acids, which are an important source of fuel. The acetyl-CoA produced through beta-oxidation enters the citric acid cycle, where it undergoes further oxidation to generate ATP.
What types of fatty acids can undergo beta-oxidation?
Beta-oxidation can occur for various types of fatty acids, including both saturated and unsaturated fatty acids. However, unsaturated fatty acids require additional enzymes to handle the presence of double bonds.
How is beta-oxidation regulated?
Beta-oxidation is regulated based on the energy needs of the cell. Hormones like epinephrine can activate beta-oxidation, while insulin inhibits the process. The regulation involves phosphorylation and dephosphorylation of key enzymes.
What is the ATP yield of beta-oxidation?
The ATP yield of beta-oxidation varies depending on the length and saturation of the fatty acid. For saturated fatty acids, the ATP yield ranges from approximately 106 ATP for a 16-carbon fatty acid to 100 ATP for a 17-carbon fatty acid.
Can odd-chain fatty acids undergo beta-oxidation?
Yes, odd-chain fatty acids can undergo beta-oxidation. However, the final product of beta-oxidation for odd-chain fatty acids is propionyl-CoA, which can be further metabolized to produce additional ATP through the conversion to succinyl-CoA.
Are there any diseases associated with beta-oxidation defects?
Yes, defects in beta-oxidation enzymes can lead to metabolic disorders known as fatty acid oxidation disorders (FAODs). These disorders can result in the inability to properly break down fatty acids, leading to various symptoms and health complications.
How is beta-oxidation different from alpha-oxidation?
Beta-oxidation refers to the breakdown of fatty acids from the carboxyl end, whereas alpha-oxidation involves the breakdown of fatty acids from the alpha-carbon end. Alpha-oxidation is a less common pathway that occurs in certain specialized tissues and is specifically involved in the metabolism of branched-chain fatty acids.
References
- Houten, S.M., Wanders, R.J.A. (2010). A general introduction to the biochemistry of mitochondrial fatty acid β-oxidation. Journal of Inherited Metabolic Disease, 33, 5: 469–477.
- Nelson, D.L., Cox, M.M. (2012). Lehninger Principles of Biochemistry, 6th Edition. New York: W. H. Freeman.
- Talley JT, Mohiuddin SS. Biochemistry, Fatty Acid Oxidation. [Updated 2023 Jan 16]. In: StatPearls [Internet]. Treasure Island (FL): StatPearls Publishing; 2023 Jan-. Available from: https://www.ncbi.nlm.nih.gov/books/NBK556002/
- Schulz, H. (2013). Encyclopedia of Biological Chemistry || Fatty Acid Oxidation. , (), 281–284. doi:10.1016/B978-0-12-378630-2.00071-2
- Kumari, Asha (2018). Sweet Biochemistry || Beta Oxidation of Fatty Acids. , (), 17–19. doi:10.1016/B978-0-12-814453-4.00004-2
- Calder, P.C. (2016). Encyclopedia of Food and Health || Fatty Acids: Metabolism. , (), 632–644. doi:10.1016/b978-0-12-384947-2.00276-2
- https://www.abcam.com/pathways/fatty-acid-oxidation
- https://lipidlibrary.aocs.org/chemistry/physics/animal-lipids/fatty-acid-beta-oxidation
- https://reactome.org/content/detail/R-HSA-77289
- https://www.slideshare.net/YESANNA/betaoxidation-of-fatty-acids
- https://old.amu.ac.in/emp/studym/99999218.04.2020.pdf
- http://adpcollege.ac.in/online/attendence/classnotes/files/1625570709.pdf
- https://www.assaygenie.com/blog/beta-oxidation-overview