What is Archaea?
- Archaea, one of the three domains of life, represent a group of single-celled organisms that are distinct from Bacteria and Eukarya. These microorganisms are considered some of the oldest life forms on Earth, showcasing primitive biological traits that have allowed them to survive in extreme environments.
- The term “Archaea” originates from the Greek word “archaios,” meaning ancient or primitive. This naming reflects their simple structural and metabolic features, as well as their ability to thrive in environments that are inhospitable to most other forms of life, such as hot springs, salt lakes, and deep-sea hydrothermal vents.
- Initially, Archaea were classified under the domain Prokaryota and referred to as Archaebacteria, due to their similarities with bacteria. Both groups share a lack of a nucleus and other membrane-bound organelles, and they exhibit similar shapes and sizes, typically ranging from 0.1 to 15 micrometers. Despite these similarities, Archaea differ significantly from bacteria in their genetic makeup and metabolic processes. This distinction was first recognized in 1977 by Carl Woese and George E. Fox, who proposed that Archaea form a separate domain based on differences in their ribosomal RNA (rRNA) sequences.
- Although Archaea resemble bacteria in many ways, they also share some characteristics with Eukaryotes, the domain that includes plants, animals, and fungi. For example, certain aspects of their genetic material and biochemical pathways are more closely related to those found in Eukaryotes than in Bacteria. This unique combination of features makes Archaea an important subject of study in understanding the evolution of life on Earth.
Definition of Archaea
Archaea are a domain of single-celled prokaryotic organisms distinct from bacteria, characterized by unique genetic, metabolic, and cellular features, and often found in extreme environments.
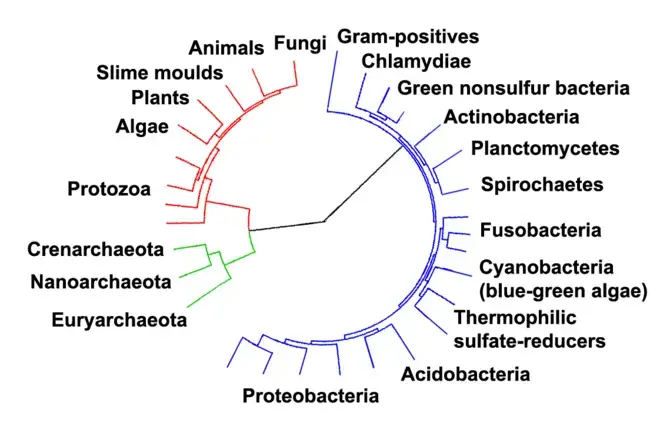
Habitat of Archaea
The habitat of Archaea is remarkably diverse, spanning from some of the most extreme environments on Earth to more common and moderate settings. Initially, Archaea were thought to exist exclusively in extreme conditions, but advances in culture-independent techniques have revealed that these microorganisms are ubiquitous across various ecosystems.
Here are some key habitats where Archaea thrive:
- Extreme Environments:
- Hypersaline Conditions: Archaea are often found in environments with high salt concentrations, such as salt and soda lakes. Halophilic species like Halobacterium, Haloquadratum, and Natronomonas dominate these regions, where they have adapted to survive and flourish despite the extreme salinity.
- High-Temperature Environments: Thermophilic Archaea are known to thrive in extremely hot environments, including hydrothermal vents, hot springs, and volcanic areas. Species like Pyrococcus furiosus, Thermococcus kodakarnesis, and Methanopyrus kandleri can survive temperatures exceeding 100°C, demonstrating their remarkable thermal stability.
- Cold Environments: Some Archaea are psychrophiles, thriving in extremely cold environments such as Antarctic lakes, Arctic oceans, and polar ice seas. Species like Halorubrum lacusprofundi, Psychromonas ingrahamii, and Methanogenium spp. are adapted to survive freezing temperatures, sometimes as low as -10°C to -20°C.
- Acidic and Alkaline Conditions: Archaea also thrive in environments with extreme pH levels. Acidophilic species like Picrophilus torridus can grow in highly acidic conditions with a pH as low as 0. On the other hand, alkaliphilic species such as Natronobacterium and Alkaliphilus spp. are found in environments with a pH greater than 11, where most life forms would struggle to survive.
- Oceanic Microflora:
- Marine Environments: Archaea constitute a significant portion of the ocean’s microflora, with an estimated 20% of oceanic microorganisms belonging to this domain. They play a crucial role in marine ecosystems, contributing to nutrient cycling and other essential processes.
Characteristics of Archaea
Archaea are a unique group of microorganisms with distinct molecular and physiological characteristics that set them apart from Bacteria and Eukarya. Their defining features are as follows:
- Cell Wall Composition:
- Unlike bacteria, Archaeal cell walls lack peptidoglycan, a key component of bacterial cell walls. Instead, Archaea exhibit a variety of cell wall compositions, often comprising polysaccharides and glycoconjugates.
- Certain methanogenic Archaea possess pseudo-murein in their cell walls, a polysaccharide similar to bacterial peptidoglycan but chemically distinct, as it lacks D-amino acids and N-acetylmuramic acid.
- Cell Membrane Structure:
- The cell membrane of Archaea is composed of glycerol-ether lipids, which are fundamentally different from the glycerol-ester lipids found in Bacteria and Eukarya.
- Archaeal membranes feature a sn-glycerol-1-phosphate backbone, in contrast to the sn-glycerol-3-phosphate backbone in other domains.
- The fatty acids in Archaeal membranes are highly branched isoprenoids and may contain rings of cyclopropane or cyclohexane, distinguishing them further from the straight-chain fatty acids of Bacteria and Eukarya. Additionally, these membranes include specialized proteins like rhodopsins, which play a role in light capture and proton gradient formation.
- Genetic Features:
- Archaea possess unique genes that encode proteins specific to their domain. Their rRNA sequences and tRNA genes are also distinct, with some Archaeal genes even containing introns.
- Horizontal gene transfer is a common phenomenon among Archaea, contributing to their genetic diversity and adaptability.
- RNA Polymerase Complexity:
- The RNA polymerases of Archaea are complex and share a closer resemblance to those of Eukaryotes than to bacterial RNA polymerases. This complexity underscores their distinct evolutionary pathway.
- Metabolic Capabilities:
- Archaea are capable of unique metabolic processes, such as methanogenesis, which involves the production of methane during metabolism and is exclusive to this domain.
- Besides methanogenesis, Archaea can engage in chemolithotrophy, photoautotrophy, and growth under hyperthermophilic conditions, highlighting their metabolic versatility.
- Adaptation to Extreme Environments:
- Archaea are renowned for their ability to inhabit extreme environments where most other life forms cannot survive. These environments include hyper-saline areas, highly acidic or basic conditions, and regions with extreme temperatures, both hot and cold.
Archaeal cell organisation, shape and size
Archaeal cells exhibit a wide range of organizational structures, shapes, and sizes, reflecting their adaptability to various environmental conditions. The following points detail the key aspects of archaeal cell organization, shape, and size:
- Cell Organization:
- Single Membrane Boundaries: Most archaea possess a simple cellular organization with a single bounding membrane. This membrane is composed of unique glycerol-ether lipids, differing from the glycerol-ester lipids found in bacteria and eukaryotes.
- Cell Wall Structure: Unlike bacteria, most archaea lack peptidoglycan in their cell walls. Instead, many have an outer layer of glycosylated proteins called the S-layer, which contributes to their shape and mechanical protection.
- Cytoskeletal Elements: Archaea possess cytoskeletal filaments that help in maintaining cell shape and facilitating cellular processes. These structures are crucial for the control of local growth and division.
- Cell Shape:
- Diverse Morphologies: Archaeal cells can exhibit a variety of shapes. Common forms include cocci (spherical) and rods (elongated). However, some archaea display geometric shapes such as flat squares and triangles, which are less common in other organisms.
- Specialized Shapes:
- Sulfolobales: These archaea typically have irregular coccoid shapes.
- Thermoplasmatales and DPANN Archaea: Members of these groups often assume simple shapes, such as short rods or cocci.
- Ignicoccus: This genus is noted for its unique double-membrane structure, lacking the S-layer and pseudomurein, with membrane “tubes” connecting compartments.
- Haloarchaea: Species like Haloferax volcanii can change shapes from rods to flat disks and exhibit extreme variability under different growth conditions.
- Cell Size:
- General Size Range: Archaeal cell sizes typically range from 0.7 μm to 4 μm, comparable to many bacterial cells.
- Extremes in Size:
- Filamentous Forms: Some archaea, such as Methanobacterium thermoautotrophicum, can form filaments up to 100 μm in length, although individual cells are generally 2-3 μm long.
- Smallest Archaea: Certain archaea, such as Nanoarchaeum equitans and Nanopusillus acidilobi, are among the smallest known living organisms, with sizes as small as 300 nm.
- Influence of Morphology:
- Mechanical Sensitivity: The lack of peptidoglycan makes archaeal cells more sensitive to mechanical changes in their environment. Their shapes can be altered by external forces, reflecting their adaptability to extreme conditions.
- Functional Implications: The variety in shape and size is believed to play a role in how archaea interact with their environments, affecting processes such as nutrient uptake, protection, and motility.
Cell envelope of Archaea
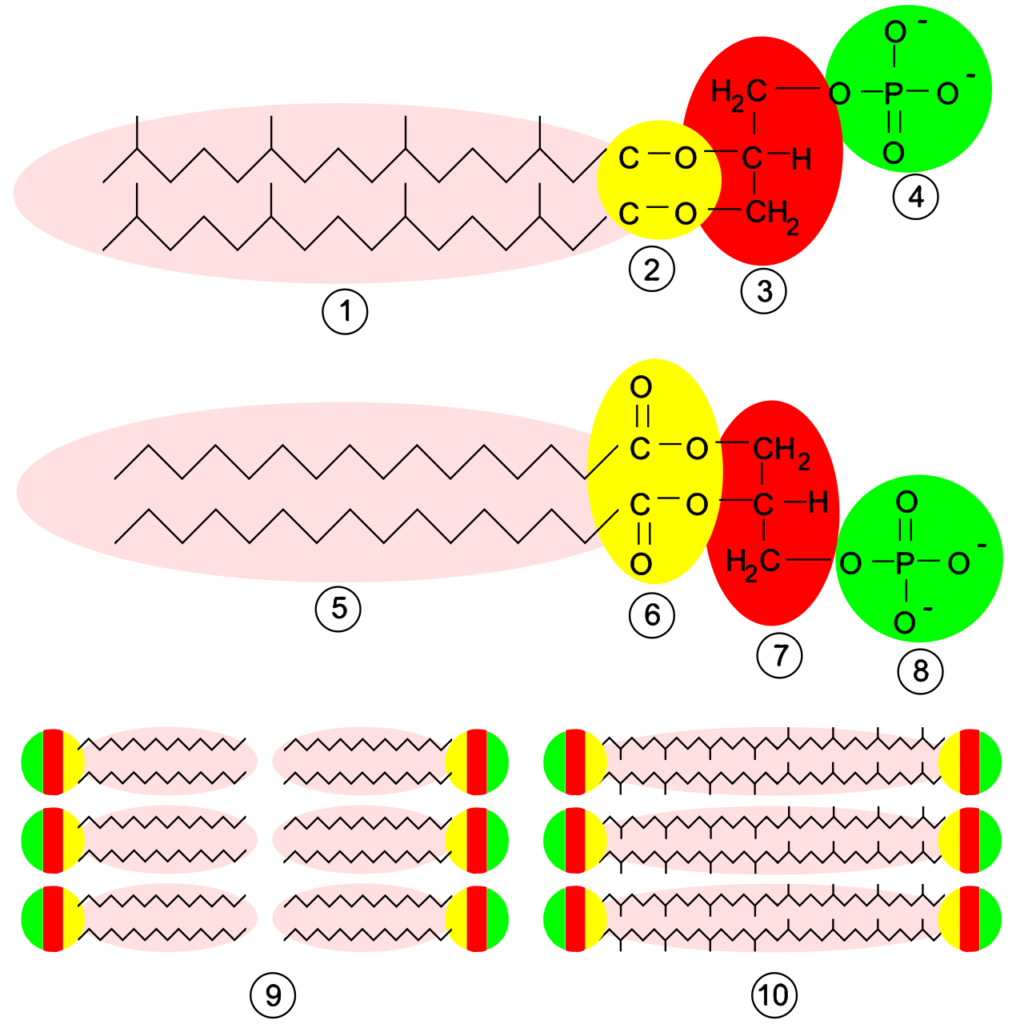
The cell envelope of archaea is a complex and varied structure that plays a crucial role in defining their shape, protecting against environmental stress, and facilitating various cellular functions. This section explores the different components of the archaeal cell envelope, including the surface layer, surface appendages, and the regulation of surface proteins.
1. Surface Layer (S-layer)
- Structure:
- Composition: The S-layer is a major component of the archaeal cell envelope, formed by one or two different protein subunits. These proteins self-assemble into para-crystalline lattices that cover the cell surface.
- Subunits: The S-layer proteins consist of a large lattice-forming segment and a smaller anchoring segment. The anchoring subunits integrate either into the cytoplasmic membrane or, in some species, into a polysaccharide layer such as pseudomurein or methanochondroitin.
- Function:
- Mechanical Stability: The S-layer provides mechanical stability and maintains cell shape. It acts as a molecular sieve, permitting the passage of molecules up to a certain size, and may protect cells from viruses and facilitate cell adhesion.
- Quasi-periplasm: The space between the cytoplasmic membrane and the S-layer forms a quasi-periplasm that can perform functions analogous to the periplasmic space in Gram-negative bacteria.
- Glycosylation:
- Modification: S-layer proteins are often modified by N-linked glycosylation and sometimes by O-linked glycosylation. These modifications can enhance protein stability and functionality.
- Role: Glycosylation of S-layer proteins is thought to contribute to cell surface recognition and adaptation to extreme environments.
2. Surface Appendages
- Types:
- Cannulae: Found in Pyrodictium species, these are hollow tubes that connect cells, facilitating communication and structural integrity within communities.
- Hami: In Altiarchaeum hamiconexum, hami are barbed-wire-like structures that anchor cells to surfaces and to one another, playing a role in biofilm formation.
- Type IV Pili: Many archaea possess type IV pili used for adhesion, biofilm formation, and as anchor points for archaeal viruses. These appendages share similarities with bacterial pili in their assembly pathway.
- Archaellum:
- Function: The archaellum, or archaeal flagellum, is essential for motility in swimming archaea. Unlike bacterial flagella, archaella are not homologous but share a similar assembly mechanism with type IV pili.
- Anchoring: The archaellum is anchored in the S-layer, where it translates ATP-driven motion into propulsion. Proteins such as ArlG and ArlF anchor the archaellum and contribute to its motor complex.
3. Regulation of the Cell Surface
- Protein Secretion:
- Sec System: Most archaeal proteins are secreted via the Sec system, which transports proteins across the membrane. Some proteins, especially cofactor-containing ones, use the TAT (twin-arginine translocation) pathway to transport folded proteins.
- Halophiles: Extreme halophiles predominantly use the TAT pathway to avoid precipitation of proteins in high-salt environments.
- Secretion Pathways:
- Pre-proteins: Secreted proteins are initially produced as pre-proteins with signal peptides. Signal Peptidase I processes these proteins, although the existence of a Signal Peptidase II equivalent in archaea is still under investigation.
- Type IV Pili and Archaellum: The assembly machinery for type IV pili and the archaellum is homologous to bacterial systems but lacks some bacterial-specific components.
- Glycosylation:
- Types: Archaeal proteins are commonly modified by N- and/or O-linked glycosylation. These modifications are important for protein stability, folding, and functionality.
- Examples: Glycosylation pathways have been extensively studied in model organisms like H. volcanii and M. maripaludis. The presence of the AglB oligosaccharyltransferase (OST) and homologs of eukaryotic OST components indicates a complex glycosylation system.
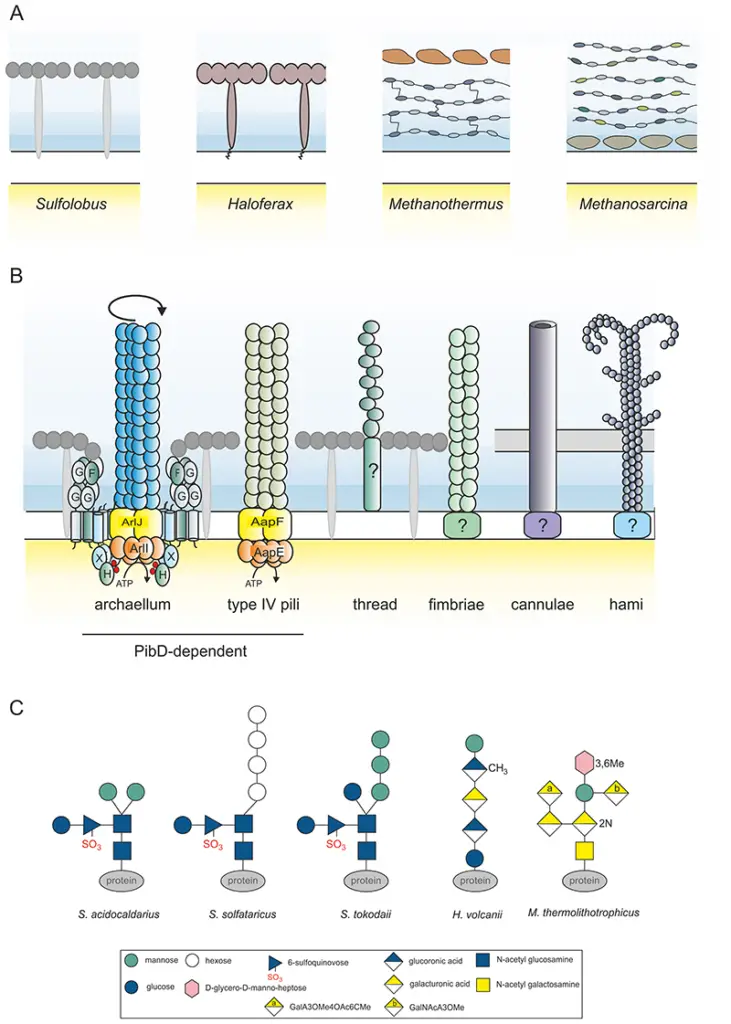
(A) Cell Envelope Structures:
Sulfolobus: S-layer proteins
Haloferax: S-layer protein with lipid anchor
Methanothermus: pseudopeptidoglycan layer with S-layer on top
Methanosarcina: S-layer and methanochondoitin layer
(B) Surface Appendages:
Archaellum
Type IV pili
Threads
Fimbriae
Cannulae
Hami(
C) N-glycan Structures:
Found on surface proteins of:
S. acidocaldarius
S. solfataricus
S. tokodaii
H. volcanii
M. thermolithotrophicus
Image Source: https://doi.org/10.1038%2Fs41564-022-01215-8
Internal organization of Archaea
Archaea, similar to bacteria, exhibit a unique internal organization marked by the presence of non-membrane-bound compartments where specific cellular functions are concentrated. This organization, although less understood, includes several key features that define the internal structure and functional dynamics of archaea.
1. Internal Compartments
- Molecular Condensates: Recent research suggests that archaea may have molecular condensates, akin to bacterial compartments, where specific cellular activities are localized. Evidence points to asymmetries in ribosome distribution, implying possible localized sites for protein synthesis or ribosomal assembly within the cell.
- Genome Localization: In archaea such as Sulfolobus and Nitrosopumulus, the genome is not uniformly distributed throughout the cytoplasm but is confined to specific regions for much of the cell cycle. This spatial organization may play a role in cellular processes such as DNA replication and transcription.
2. Membrane Organization
- Complex Membrane Structures: Some archaea, particularly crenarchaeota like I. hospitalis, possess complex membrane systems that include an inner membrane connected to an external membrane. The extent and genetic basis of this complexity across different archaeal species are still under investigation.
- Membrane Trafficking Proteins: Archaea often contain homologues of the ESCRT-III and Vps4 machinery, which are involved in membrane remodeling and vesicle formation. In Asgard archaea, additional homologues of ubiquitin and other ESCRT proteins suggest advanced membrane trafficking capabilities. The exact mechanisms and their parallels with eukaryotic systems remain an area of active research.
3. Genome Organization
- Circular Genomes: Archaea generally have small, circular genomes organized into functional groups. The replication and organization of these genomes vary among species. For instance, Haloferax species have multiple nucleoid copies, whereas Sulfolobales and Nitrosopumulus maintain a single-copy genome, with synchronized replication origins regulated by homologues of the eukaryotic AAA ATPase Cdc6.
- Chromosomal Packing: In many archaea, the genome is organized into distinct cytoplasmic regions rather than being diffusely located. High-resolution imaging and HiC experiments have shown that the genome in Sulfolobus has a higher-order structure, facilitated by proteins like Coalescin, which is part of the Smc condensin/conhesin family. This structure plays a role in global transcription regulation and genome evolution.
4. Cytoskeleton
- Cytoskeletal Filaments: Archaea possess various cytoskeletal filaments, including homologues of actin, tubulin, and ESCRT-III. These filaments are essential for cell shape and division. For instance:
- Actin Homologues: Archaeal actins, such as crenactin, are structurally similar to eukaryotic actin and may contribute to cell shape and morphology. In rod-shaped archaea, actin homologues likely play a role in maintaining cellular shape.
- MreB and Tubulin Homologues: MreB, an actin homologue, and tubulin-like proteins such as CetZ are involved in shaping the cell and organizing the cytoskeleton. CetZ, for example, is crucial for transitioning cell shape in H. volcanii.
- Regulation and Function: The dynamic behavior of archaeal cytoskeletal filaments, including their interactions with other cellular components like the S-layer, needs further exploration. The role of these filaments in controlling cell shape and division is an ongoing area of study.
Cell division of Archaea
Archaeal cell division involves distinct mechanisms that highlight the complexity and diversity within this domain. Two principal systems are observed: the FtsZ-based system and the ESCRT-III-based system. Each system employs specific proteins and mechanisms to accomplish cell division, reflecting the evolutionary adaptations of archaea.
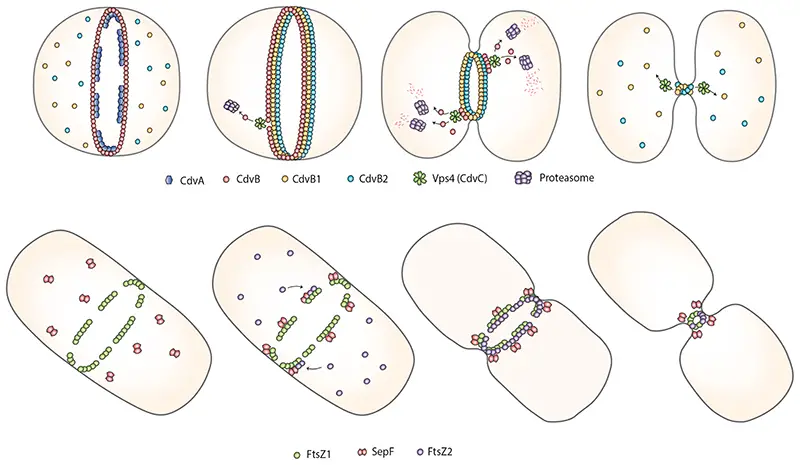
Lower panel: the FtsZ based cell division process of H. volcanii as displayed in four stages: (1) FtsZ1 forms a ring at mid-cell. (2) SepF dimers localize in an FtsZ1-dependent manner to the future cell division site, and SepF recruits FtsZ2. (3). FtsZ2 forms a ring. (4) Constriction of the divisome results in cell division. Image Source: https://doi.org/10.1038%2Fs41564-022-01215-8
1. FtsZ-Based Cell Division
- FtsZ Proteins:
- Role and Mechanism: Most archaea outside the Crenarchaeota possess FtsZ, a tubulin homologue essential for cytokinesis. FtsZ forms a ring structure at the cell’s midsection, which constricts to facilitate division. This process is analogous to bacterial cell division, where FtsZ also guides cell wall synthesis.
- Localization and Function: In Haloferax volcanii, FtsZ forms a mid-cell ring that contracts during cytokinesis. This archaeon contains two FtsZ proteins, FtsZ1 and FtsZ2, which have complementary roles. FtsZ1 helps scaffold the Z-ring, while FtsZ2 initiates constriction.
- Experimental Models: Methanobrevibacter smithii, another archaeal model, has a single FtsZ protein and a pseudopeptidoglycan cell wall. FtsZ1 in this organism also forms a ring at the mid-cell but with unique features compared to bacterial systems.
- Recent Findings: New studies reveal that SepF, a protein homologous to bacterial SepF, anchors the Z-ring to the membrane. However, differences in SepF structure between archaea and bacteria suggest distinct evolutionary paths and functional adaptations.
- FtsZ Dynamics:
- Protein Interactions: The interaction between FtsZ and other proteins, such as SepF, is critical for proper Z-ring formation and function. Despite similarities to bacterial systems, differences in protein structures and functions underscore the evolutionary divergence between archaea and bacteria.
- Cytokinesis Defects: Deletions of FtsZ1 or FtsZ2 in H. volcanii lead to severe defects in cell division, highlighting the essential role of these proteins in maintaining cellular integrity during division.
2. ESCRT-III-Based Cell Division
- ESCRT-III Complex:
- Components and Function: In Sulfolobus acidocaldarius, ESCRT-III proteins, including CdvA, CdvB, CdvB1, and CdvB2, drive cell division. CdvA defines the midzone, and the ESCRT-III complex forms a ring that constricts to mediate membrane scission. This system parallels the ESCRT machinery in eukaryotic cells, which is involved in membrane remodeling and vesicle formation.
- Process: The assembly of the ESCRT-III complex is followed by disassembly, which is crucial for membrane remodeling. Vps4, an AAA ATPase, is involved in the disassembly of the ESCRT-III ring, facilitating cell division by providing energy for membrane constriction.
- System Characteristics:
- Role of CdvA and CdvB: CdvA initiates the formation of a non-contractile ring of CdvB, which is subsequently replaced by CdvB1 and CdvB2. The dynamic assembly and disassembly of this complex are critical for successful cytokinesis.
- Cytokinesis Arrest: Truncation mutants of ESCRT-III proteins that cannot interact with Vps4 exhibit division arrest, indicating the importance of this interaction for completing cell division.
3. Diverse Mechanisms Across Archaeal Groups
- Asgard and Thaumarchaeota:
- Dual Systems: Some Asgard and Thaumarchaeota archaea possess both FtsZ and ESCRT-III homologues. In Nitrosopumilus maritimus, CdvA, CdvB, and CdvC localize to mid-cell, whereas the role of the FtsZ-like protein remains unclear due to its diffuse localization.
- Functional Uncertainty: The presence of both division systems in these archaea raises questions about whether they function together or independently.
- Thermoprotales:
- Alternative Division Mechanisms: Members of the Thermoprotales lack FtsZ and ESCRT-III proteins. Instead, these archaea may use crenactin and arcadins, components of the cytoskeleton, to facilitate division. This suggests an alternative division mechanism that may parallel bacterial systems lacking FtsZ.
Metabolism of Archaea
Navigating through the metabolic pathways of Archaea unveils a myriad of biochemical reactions, some of which are universally exhibited across all archaeal species, while others are taxon-specific, reflecting the metabolic diversity and adaptability of this domain of life.
- Nutritional Diversity and Chemotrophy: Archaea manifest a remarkable nutritional diversity, categorizing them into three primary nutritional groups: phototrophic, lithotrophic, and organotrophic. Lithotrophic and organotrophic archaea, often collectively referred to as chemotrophs, engage in a spectrum of roles within their ecological niches. These include functioning as nitrifiers, methanogens, and anaerobic methane oxidizers, the latter being predominant inhabitants of anaerobic environments.
- Phototrophic Metabolism: Phototrophic archaea, while capable of harnessing sunlight through chemiosmosis, do not fix atmospheric carbon, differentiating them from photosynthetic organisms. Their energy acquisition from light does not translate into the conventional photosynthetic pathway observed in other domains of life.
- Respiration and Glycolysis: Archaea execute both aerobic and anaerobic respiration, adapting to the availability of oxygen in their respective habitats. The glycolytic pathway within archaea is a modified variant of the processes observed within eukarya and bacteria, reflecting the evolutionary divergence and adaptation of archaea to varied environmental niches.
- Citric Acid Cycle: Archaea engage in the citric acid cycle, albeit with variations that may encompass complete or partial cycles, depending on the specific metabolic requirements and ecological niches of the organisms.
- Methanogenesis: A hallmark of certain archaea, particularly those residing in anaerobic environments, is methanogenesis – the production of methane. This metabolic pathway is not only significant for the organisms and their environments but also posits a fascinating hypothesis regarding the evolutionary history of life on Earth. The evolution of methanogenesis is hypothesized to be an ancient metabolic pathway, potentially indicative of the metabolic characteristics of some of the earliest free-living organisms on the planet.
- Unique Coenzymes: Archaea utilize a distinctive set of coenzymes for methanogenesis, such as methanofuran and coenzyme M, which are not observed in other domains of life. The chemical structure of methanofuran, for instance, underscores the unique biochemical adaptations of methanogenic archaea, facilitating their survival and metabolic activity in specific ecological contexts.
- In synthesizing the above, it is evident that the metabolic pathways of Archaea are not merely a reflection of their survival strategies but also a testament to their evolutionary history and ecological significance. Their metabolic diversity and adaptability not only enable them to inhabit a plethora of environments, ranging from the mundane to the extreme but also play pivotal roles in global biogeochemical cycles, thereby influencing both their immediate habitats and the global ecosystem.
Genetics of Archaea
The genetic framework of Archaea, while maintaining its prokaryotic nature, reveals a fascinating amalgamation of characteristics that are uniquely archaeal, while also sharing certain features with both bacterial and eukaryotic domains. The exploration of archaeal genetics provides insights into their adaptability, evolutionary history, and their capacity to inhabit diverse ecological niches.
- Chromosomal Characteristics: Archaea typically harbor a single, circular chromosome, with the largest known archaeal genome being attributed to Methanosarcina acetivorans (5,751,492 base pairs) and the smallest to Nanoarchaeum equitans (490,885 base pairs). This variation in genome size among archaeal species underscores the genetic diversity within this domain, reflecting their adaptability and specialized functionalities across varied habitats.
- Plasmid Presence and Conjugation: Archaea, akin to bacteria, possess plasmids—small, often circular, fragments of DNA that can be transferred between cells through a process analogous to bacterial conjugation. This inter-cell transfer mechanism facilitates the exchange of genetic material, thereby potentially contributing to the adaptive capabilities and genetic diversity within archaeal populations.
- Genetic Distinctiveness: Despite the prokaryotic cellular organization, archaeal genetics exhibit a distinctiveness that sets them apart from bacteria and eukaryotes. This distinctiveness is manifested in their transcriptional and post-transcriptional processes, which bear more resemblance to eukaryotic systems than to bacterial ones.
- Transcriptional Mechanisms: The transcriptional machinery of Archaea, particularly the RNA polymerase (RNAP), shares a closer resemblance to eukaryotic RNAP II, highlighting an intriguing evolutionary relationship between these two domains. While certain archaeal transcription factors (TFs) bear similarity to bacterial TFs, the overall transcriptional process aligns more closely with eukaryotic mechanisms, offering a unique perspective into the evolutionary divergence and commonalities among these domains of life.
- Post-Transcriptional Modifications (PTMs): Archaea exhibit post-transcriptional modifications that are notably similar to those observed in eukaryotes. This similarity extends to various aspects of mRNA processing and modification, further emphasizing the shared ancestral traits and divergent evolutionary pathways between Archaea and Eukarya.
Archaeal Groups Inhabiting Different Extreme Habitats
Archaea, renowned for their resilience, have evolved to thrive in some of the most inhospitable environments on Earth. These extremophilic archaea have developed unique physiological and metabolic adaptations that allow them to survive and flourish under conditions that would be lethal to most other life forms. Here, we delve into the various groups of archaea that inhabit different extreme habitats:
- Halophiles:
- Habitat: These archaea are specially adapted to survive in environments with high salt concentrations, such as salt flats, salt mines, and hypersaline lakes.
- Example: One of the most studied halophiles is the genus Halobacterium spp., which thrives in salt ponds and can even survive in saturated salt solutions.
- Adaptation: Halophiles maintain osmotic balance in their cells by accumulating high concentrations of potassium ions or by synthesizing or accumulating organic solutes.
- Thermophiles:
- Habitat: Thermophiles are adapted to life in extremely hot environments, including geothermal springs, deep-sea hydrothermal vents, and areas around volcanoes.
- Example: Methanopyrus kandleri is a notable thermophile that can thrive at temperatures exceeding 100°C.
- Adaptation: These organisms possess heat-stable enzymes and specialized lipid membranes that remain fluid and functional at high temperatures.
- Alkaliphiles:
- Habitat: Alkaliphiles are found in environments with extremely high pH levels, such as soda lakes and certain marine hydrothermal systems.
- Example: Thermococcus alcaliphilus is a marine archaeon that thrives in alkaline conditions.
- Adaptation: Alkaliphiles maintain a stable internal pH by actively pumping protons into their cells and possess enzymes that function optimally at high pH levels.
- Acidophiles:
- Habitat: These archaea thrive in highly acidic environments, such as acid mine drainages, acidic peat bogs, and volcanic sites.
- Example: Picrophilus torridus is known to survive in environments with a pH close to zero.
- Adaptation: Acidophiles have evolved mechanisms to keep their internal pH near neutrality, often by actively pumping out protons or by synthesizing protective molecules.
It’s worth noting that the boundaries between these categories are not always distinct. Many archaea exhibit polyextremophilic traits, meaning they can survive in multiple extreme conditions simultaneously. For instance, an organism might be both a thermophile and an acidophile, thriving in hot, acidic springs.
Classification of Archaea
The classification of Archaea is based on their phylogenetic relationships, which reveal a variety of distinct phyla within this domain. Here is a detailed explanation of some of the key phyla:
- Euryarchaeota:
- Euryarchaeota is the largest and most diverse phylum of Archaea. It includes a wide range of genera capable of thriving in extreme environments, such as highly alkaline, saline, and thermophilic conditions.
- This phylum is known for housing numerous methanogens—organisms that produce methane as a byproduct of their metabolism.
- While Euryarchaeota was initially believed to consist solely of extremophiles, many of its genera have been isolated from more moderate environments, such as soil, water, and even the intestines of animals.
- Thermoproteota (formerly Crenarchaeota):
- Thermoproteota, previously known as Crenarchaeota, includes some of the most abundant marine Archaea. Many species within this phylum are sulfur-dependent and thrive in high-temperature environments.
- This group is composed predominantly of thermophiles and extreme thermophiles, which are organisms that require very high temperatures to survive.
- Nitrososphaerota:
- Nitrososphaerota is a proposed phylum that emerged from the reclassification of certain species initially placed under Thermoproteota.
- This phylum includes species such as Cenarchaeum symbiosum and members of the Nitrosopumilus genus. The differentiation is based on distinct rRNA gene sequences and topoisomerase enzyme structures, leading to the proposal of this new phylum in 2008.
- Nanoarchaeota:
- Nanoarchaeota is a relatively recent addition to the Archaeal domain. It primarily consists of Nanoarchaeum equitans, one of the few well-recognized and culturable species within this group.
- This phylum is still under investigation, with other genera being proposed but not yet fully characterized.
- Korarchaeota (also known as Xenarchaeota):
- Korarchaeota represents a deep branching lineage within Archaea, distinguished by unique rRNA and protein sequences that set it apart from other phyla.
- This group appears to exhibit characteristics of both Euryarchaeota and Thermoproteota, indicating a complex evolutionary history.
- Aigarchaeota:
- Aigarchaeota is another proposed phylum that includes the species Caldiarchaeum subterraneum. However, this classification is still debated within the scientific community.
- The ongoing debate centers around the similarities between Aigarchaeota and members of the Nitrososphaerota phylum, which has led to questions about whether Aigarchaeota should be considered a distinct phylum.
Ecological Significances of Archaea
Archaea, a diverse group of microorganisms, play vital roles in various ecological processes across the globe, from extreme environments to more moderate habitats. Here are some of their key ecological significances:
- Methanogenesis:
- Archaea are the sole biological entities capable of methanogenesis, a metabolic process that produces methane gas. This process is crucial in anaerobic environments like wetlands, sediments, and the guts of ruminants, where it contributes significantly to the global methane budget.
- Nutrient Cycling in Extreme Habitats:
- Archaea’s ability to thrive in extreme environments, such as hot springs, hydrothermal vents, and acidic or saline conditions, makes them essential players in nutrient cycling in these areas.
- They contribute to the breakdown of organic matter and the transformation of inorganic minerals, thus enriching these harsh environments with essential nutrients and paving the way for the development of more complex ecosystems.
- Biogeochemical Cycling:
- Archaea are integral to various biogeochemical cycles, including the nitrogen, carbon, and sulfur cycles.
- For instance, they participate in nitrification, where ammonia is oxidized to nitrite, a key step in the nitrogen cycle. Their involvement in carbon fixation and methane production also influences the global carbon cycle.
- Symbiotic Relationships:
- Many Archaea engage in symbiotic relationships with other organisms. They can be found in the roots of plants, where they assist in nutrient uptake, in coral reefs, contributing to the reef’s health, and in the intestines of animals, where they aid in digestion and other metabolic processes.
- Maintenance of Marine Ecosystems:
- Archaea constitute about 20% of marine microflora, underscoring their importance in marine ecosystems.
- They play a critical role in maintaining biogeochemical cycles, particularly in the production and decomposition of organic matter. Their activities help regulate the balance of nutrients in marine environments, supporting the diverse life forms that inhabit these waters.
Applications of Archaea
Archaea, a group of microorganisms known for their ability to thrive in extreme environments, have found numerous applications across various fields due to their unique properties. Here are some of the key applications of Archaea:
- Industrial Enzymes:
- Enzymes derived from extremophilic Archaea are highly stable and functional under extreme conditions, such as high temperatures, salinity, and acidity.
- These enzymes are employed in several industrial processes, including food processing, where they aid in the production of certain flavors and textures, and in the detergent industry, where they enhance the effectiveness of cleaning agents under various conditions.
- Additionally, these enzymes are utilized in leather processing to break down tough proteins and in the synthesis of thermophilic enzymes that are used in molecular biology and other biochemical applications.
- Biogas Production:
- Certain Archaea, particularly methanogens, are instrumental in the production of biogas.
- These microorganisms metabolize organic matter in anaerobic environments, leading to the production of methane, a renewable energy source. This process is utilized in waste treatment facilities to convert organic waste into usable biogas, thereby contributing to energy generation and waste management.
- Bioremediation:
- Archaea play a crucial role in bioremediation, the process of using microorganisms to remove or neutralize pollutants from contaminated environments.
- They are capable of breaking down harmful chemical wastes and metals, a process known as bioleaching, which is particularly useful in mining and waste management.
- Furthermore, Archaea are employed to degrade pollutants in environments that are inhospitable to other organisms, such as highly acidic or saline areas, thereby restoring these ecosystems.
- Fermentation and Probiotics:
- Archaea are involved in fermentation processes, particularly in the production of cheese and other fermented foods.
- Their unique metabolic pathways contribute to the development of specific flavors and textures that are characteristic of certain fermented products.
- Additionally, some Archaea are being explored for their potential as probiotics, where they can promote gut health and enhance digestion.
- Biorefinery Processes:
- Researchers are investigating the use of Archaea in biorefinery processes, which involve the production of valuable products such as amino acids, enzymes, and biofuels from biomass.
- The robust nature of Archaea makes them suitable for these processes, where they can efficiently convert raw materials into desired products, contributing to sustainable industrial practices.
- Bioplastic Production:
- Some species of Archaea have been found to produce polyhydroxyalkanoates (PHAs), a type of biodegradable plastic.
- PHAs produced by Archaea are being explored as an eco-friendly alternative to conventional plastics, offering the potential for reducing plastic pollution and contributing to the development of sustainable materials.
Differences between Archaea and Bacteria
Archaea and Bacteria, both prokaryotic in nature, exhibit distinct differences in their cellular, metabolic, and genetic characteristics. While they share some similarities, their differences underscore the unique evolutionary paths they have taken. Here, we elucidate the primary distinctions between these two domains of life:
1. Membrane Composition:
- Archaea: Their cell membranes predominantly consist of ether-linked lipids.
- Bacteria: Their membranes are characterized by ester-linked lipids, similar to Eukarya.
2. Cell Wall Composition:
- Archaea: They lack peptidoglycan in their cell walls but possess pseudopeptidoglycan.
- Bacteria: Their cell walls contain peptidoglycan, a unique structural molecule.
3. RNA Polymerases:
- Archaea: They possess multiple types of RNA polymerases.
- Bacteria: Typically, they have only one type of RNA polymerase.
4. Transcription and Translation:
- Archaea: Their transcription and translation mechanisms closely resemble those of Eukarya.
- Bacteria: They possess unique transcription and translation mechanisms distinct from both Archaea and Eukarya.
5. Initiation Codon for Protein Synthesis:
- Archaea: Methionine serves as the initiation codon.
- Bacteria: Formylmethionine is the initiation codon.
6. Reproductive Strategies:
- Archaea: Predominantly reproduce through binary fission, budding, and fragmentation.
- Bacteria: While they also employ binary fission, budding, and fragmentation, some bacteria can form spores as a survival strategy.
7. Environmental Resilience:
- Archaea: They are exceptionally robust and can withstand extreme environmental conditions.
- Bacteria: Generally, they are more fragile in comparison to archaea, especially under harsh conditions.
8. Metabolic Activities:
- Archaea: Notable for diazotrophy, chemotrophy, and methanogenesis—a unique form of anaerobic respiration.
- Bacteria: Exhibit a wide range of metabolic activities including photosynthesis, respiration, autotrophy, fermentation, and diazotrophy.
9. Genetic Affinity to Eukarya:
- Archaea: They share a greater genetic similarity to Eukarya.
- Bacteria: Their genetic makeup is less similar to Eukarya.
10. Sensitivity to Toxins:
- Archaea: Sensitive to diphtheria toxin.
- Bacteria: Generally resistant to diphtheria toxin.
11. Representative Organisms:
- Archaea: An example is Halobacterium spp.
- Bacteria: A commonly studied bacterium is Escherichia coli.
Characteristics | Archaea | Bacteria |
---|---|---|
Membrane Composition | Ether-linked lipids | Ester-linked lipids |
Cell Wall Composition | Lacks peptidoglycan, has pseudopeptidoglycan | Contains peptidoglycan |
RNA Polymerases | Multiple types | One type |
Transcription and Translation | Resembles Eukarya | Unique to Bacteria |
Initiation Codon for Protein Synthesis | Methionine | Formylmethionine |
Reproductive Strategies | Binary fission, budding, fragmentation | Binary fission, budding, fragmentation, spore formation |
Environmental Resilience | Very robust | Relatively fragile |
Metabolic Activities | Diazotrophy, chemotrophy, methanogenesis | Photosynthesis, respiration, autotrophy, fermentation, diazotrophy |
Genetic Affinity to Eukarya | Greater similarity | Less similarity |
Sensitivity to Toxins | Sensitive to diphtheria toxin | Resistant to diphtheria toxin |
Representative Organisms | Halobacterium spp. | Escherichia coli |
Archaea vs. Bacteria vs. Eukarya
The three primary domains of life – Archaea, Bacteria, and Eukarya – exhibit distinct characteristics that set them apart from one another. Here, we present a comparative analysis of these domains based on various cellular and molecular attributes:
- Cell Type:
- Archaea: Prokaryotic
- Bacteria: Prokaryotic
- Eukarya: Eukaryotic
- Habitat/Ecology:
- Archaea: Predominantly found in extreme environments, but also present in normal conditions.
- Bacteria: Primarily inhabit normal environments, with a few species in extreme conditions.
- Eukarya: Inhabit a wide range of moderate or normal environments.
- Cellular Organization:
- Archaea: Unicellular
- Bacteria: Unicellular
- Eukarya: Both unicellular and multicellular organisms.
- Cell Organelles:
- Archaea: Lack membrane-bound organelles.
- Bacteria: Lack membrane-bound organelles.
- Eukarya: Possess membrane-bound organelles.
- Cell Wall Composition:
- Archaea: Composed of pseudopeptidoglycan, various polysaccharides, and glycoconjugates.
- Bacteria: Primarily composed of peptidoglycan.
- Eukarya: Composed of cellulose, hemicellulose, other polysaccharides, and chitin.
- Cell Membrane Composition:
- Archaea: Characterized by ether-linked lipids with branched side-chains and a sn-glycerol-1-phosphate backbone.
- Bacteria: Comprise ester-linked lipids with unbranched side-chains and a sn-glycerol-3-phosphate backbone.
- Eukarya: Contain ester-linked lipids with unbranched side-chains and a sn-glycerol-3-phosphate backbone.
- Horizontal Gene Transfer:
- Archaea: Common in many species.
- Bacteria: Occurs in some species.
- Eukarya: Rare, but observed in certain species.
- Histone and Histone-like Proteins:
- Archaea: Presence of histone-like proteins.
- Bacteria: Absent.
- Eukarya: Characterized by the presence of histone proteins.
- Introns:
- Archaea: Present.
- Bacteria: Absent.
- Eukarya: Present.
- Ribosomal RNA (rRNA):
- Archaea: Distinct rRNA sequence.
- Bacteria: Distinct rRNA sequence.
- Eukarya: Distinct rRNA sequence.
- Mode of Reproduction:
- Archaea: Primarily asexual, through fission.
- Bacteria: Primarily asexual, through fission.
- Eukarya: Both sexual and asexual modes of reproduction.
- RNA Polymerases:
- Archaea: Possess multiple and complex RNA polymerases.
- Bacteria: Characterized by a single RNA polymerase.
- Eukarya: Have multiple and complex RNA polymerases.
- Methanogenesis:
- Archaea: Present.
- Bacteria: Absent.
- Eukarya: Absent.
Characteristics | Archaea | Bacteria | Eukarya |
---|---|---|---|
Cell Type | Prokaryotic (lacks a nucleus) | Prokaryotic (lacks a nucleus) | Eukaryotic (contains a nucleus) |
Habitat/Ecology | Thrive in extreme environments (e.g., hot springs, salt lakes) & normal conditions | Primarily in normal conditions (e.g., soil, water), some in extreme environments | Diverse environments ranging from forests to oceans |
Cellular Organization | Unicellular organisms | Unicellular organisms | Includes unicellular (e.g., yeast) and multicellular organisms (e.g., humans) |
Cell Organelles | Absence of membrane-bound organelles | Absence of membrane-bound organelles | Presence of membrane-bound organelles (e.g., mitochondria, Golgi apparatus) |
Cell Wall Composition | Pseudopeptidoglycan, diverse polysaccharides, and glycoconjugates | Peptidoglycan dominates | Varies: plants (cellulose), fungi (chitin), animals (absent) |
Cell Membrane Composition | Ether-linked lipids with branched side-chains | Ester-linked lipids with straight side-chains | Ester-linked lipids with straight side-chains |
Horizontal Gene Transfer | Common, especially in adaptation to extreme environments | Common, leading to antibiotic resistance and other traits | Less common, but observed in some species |
Histone Proteins | Histone-like proteins present, aiding in DNA packaging | Absent, but have other DNA-binding proteins | Histones present, forming nucleosomes for DNA packaging |
Introns | Present in some genes | Typically absent | Commonly present in genes |
Ribosomal RNA (rRNA) | Unique rRNA sequence differentiating them from other domains | Distinct rRNA sequence, used for bacterial classification | Distinct rRNA sequence, used in studying eukaryotic evolution |
Mode of Reproduction | Asexual (binary fission) | Asexual (binary fission) | Both asexual (mitosis) and sexual (meiosis) |
RNA Polymerases | Multiple types, similar to eukaryotic RNA polymerases | Single type, simpler than those in Archaea and Eukarya | Multiple types, involved in various transcription processes |
Methanogenesis | Unique ability to produce methane during metabolism | Methane production absent | Methane production absent |
Importance of Archaea
Archaea, once thought to be a subset of bacteria, have been recognized as a distinct domain of life due to their unique genetic, biochemical, and structural characteristics. These microorganisms play several crucial roles in various ecosystems and have potential applications in biotechnology. Here are some of the key importances of Archaea:
- Biogeochemical Cycling:
- Methanogenesis: Certain archaea, known as methanogens, are the only organisms that produce methane as a metabolic byproduct. This process plays a significant role in the carbon cycle.
- Nitrogen Cycling: Some archaea are involved in the nitrogen cycle, particularly in the process of nitrification.
- Extremophiles and Understanding Life’s Limits:
- Many archaea are extremophiles, thriving in environments like high salinity (halophiles), extreme temperatures (thermophiles and psychrophiles), and high acidity or alkalinity (acidophiles and alkaliphiles). Studying these organisms provides insights into the limits of life and the potential for life on other planets.
- Evolutionary Significance:
- Archaea provide insights into the early evolutionary history of life on Earth. They represent one of the three primary domains of life and help us understand the evolutionary relationships between prokaryotes and eukaryotes.
- Biotechnological Applications:
- Enzymes: The extremophilic nature of many archaea means they produce enzymes that function under extreme conditions. These enzymes have applications in industries like biofuel production, food processing, and pharmaceuticals.
- Biogas Production: Methanogenic archaea are used in anaerobic digesters to produce biogas, a renewable energy source.
- Bioremediation: Some archaea can metabolize or transform toxic compounds, making them useful in bioremediation efforts to clean up polluted environments.
- Ecological Roles in Extreme Environments:
- Archaea play vital roles in ecosystems where few other organisms can survive, such as deep-sea hydrothermal vents, acidic lakes, and high-salinity ponds. They contribute to the productivity and functioning of these ecosystems.
- Symbiotic Relationships:
- Some archaea live in symbiotic relationships with other organisms. For instance, methanogenic archaea in the guts of ruminants help in digesting complex polysaccharides, producing methane as a byproduct.
- Understanding Cellular Mechanisms:
- While archaea are prokaryotic, many of their cellular processes, especially those related to gene expression, are more similar to eukaryotes than bacteria. Studying archaea can provide insights into the evolution and functioning of more complex cellular mechanisms.
Examples of Archaea
Archaea are a diverse group of prokaryotic microorganisms that are distinct from bacteria. They are often found in extreme environments, but they also inhabit more common environments. Here are some examples of Archaea:
- Halophiles (Salt-loving Archaea)
- Halobacterium salinarum: Found in salt ponds and salt flats.
- Halococcus morrhuae: Isolated from salted fish.
- Thermophiles (Heat-loving Archaea)
- Thermoproteus tenax: Found in hot springs.
- Pyrodictium occultum: Discovered in deep-sea hydrothermal vents.
- Methanogens (Methane-producing Archaea)
- Methanobacterium formicicum: Produces methane from formate.
- Methanosarcina barkeri: Can produce methane from several substrates, including acetate, methylamines, and methanol.
- Methanococcus jannaschii: Isolated from deep-sea hydrothermal vents.
- Acidophiles (Acid-loving Archaea)
- Ferroplasma acidarmanus: Found in acidic, iron-rich environments.
- Hyperthermophiles (Extreme heat-loving Archaea)
- Pyrococcus furiosus: Thrives at temperatures above 100°C.
- Methanopyrus kandleri: Found in hydrothermal vents and can survive at temperatures up to 122°C.
- Psychrophiles (Cold-loving Archaea)
- Methanogenium frigidum: Found in cold marine sediments.
- Alkaliphiles (Base-loving Archaea)
- Natronomonas pharaonis: Thrives in highly alkaline environments like soda lakes.
- Piezophiles (Pressure-loving Archaea)
- Pyrococcus abyssi: Found in deep-sea hydrothermal vents and can withstand high pressures.
These are just a few examples, and the world of Archaea is vast and diverse. Many archaeal species are yet to be discovered and characterized.
Quiz
References
- Grogan, D. W. (2013). Archaea. Brenner’s Encyclopedia of Genetics, 180–182. doi:10.1016/b978-0-12-374984-0.00092-9
- Casamayor, E.O. (2009). Encyclopedia of Inland Waters || Archaea. , (), 167–181. doi:10.1016/B978-012370626-3.00120-4
- McLain, J.E.T. (2005). Encyclopedia of Soils in the Environment || ARCHAEA. , (), 88–94. doi:10.1016/b0-12-348530-4/00525-7
- DasSarma, S. (2009). Encyclopedia of Microbiology || Archaea (overview). , (), 1–23. doi:10.1016/b978-012373944-5.00108-5
- Madigan Michael T, Bender, Kelly S, Buckley, Daniel H, Sattley, W. Matthew, & Stahl, David A. (2018). Brock Biology of Microorganisms (15th Edition). Pearson.
- Berthold, E. (2018, August 13). What are archaea? Curious. https://www.science.org.au/curious/earth-environment/what-are-archaea
- Bruslind, L. (n.d.). Archaea. In General Microbiology. Oregon State University. Retrieved June 10, 2021, from https://open.oregonstate.education/generalmicrobiology/chapter/archaea/
- Introduction to the Archaea. Retrieved June 10, 2021, from https://ucmp.berkeley.edu/archaea/archaea.html
- Society, M. Why don’t archaea cause disease? Retrieved June 10, 2021, from https://microbiologysociety.org/blog/why-dont-archaea-cause-disease.html
- Archaea vs Bacteria- Definition, 15 Major Differences, Examples. Retrieved June 10, 2021, from https://microbenotes.com/archaea-vs-bacteria/
- Howland JL (2000). The Surprising Archaea: Discovering Another Domain of Life. Oxford: Oxford University Press. pp. 25–30. ISBN 978-0-19-511183-5.
- Woese CR, Fox GE (November 1977). “Phylogenetic structure of the prokaryotic domain: the primary kingdoms”. Proceedings of the National Academy of Sciences of the United States of America. 74 (11): 5088–90. Bibcode:1977PNAS…74.5088W. doi:10.1073/pnas.74.11.5088. PMC 432104. PMID 270744
- Ciccarelli FD, Doerks T, von Mering C, Creevey CJ, Snel B, Bork P (March 2006). “Toward automatic reconstruction of a highly resolved tree of life”. Science. 311 (5765): 1283–87.
- Bibcode:2006Sci…311.1283C. CiteSeerX 10.1.1.381.9514. doi:10.1126/science.1123061. PMID 16513982. S2CID 1615592
- Tortora, G.J., Funke, B.R., Case, C.L. and Johnson, T.R., 2004. Microbiology: an introduction (Vol. 9). San Francisco, CA: Benjamin Cummings.
- Gaci, N., Borrel, G., Tottey, W., O’Toole, P. W., & Brugère, J. F. (2014). Archaea and the human gut: new beginning of an old story. World journal of gastroenterology, 20(43), 16062–16078. https://doi.org/10.3748/wjg.v20.i43.16062
- I.BergmannI. Bergmann, E.NettmannE. Nettmann, K.MundtK. Mundt, and M.KlockeM. Klocke. Determination of methanogenic Archaea abundance in a mesophilic biogas plant based on 16S rRNA gene sequence analysis. Canadian Journal of Microbiology. 56(5): 440-444. https://doi.org/10.1139/W10-021
- Wagner, A., Whitaker, R., Krause, D. et al. Mechanisms of gene flow in archaea. Nat Rev Microbiol 15, 492–501 (2017). https://doi.org/10.1038/nrmicro.2017.41
- Fröls, S., Ajon, M., Wagner, M., Teichmann, D., Zolghadr, B., Folea, M., Boekema, E. J., Driessen, A. J., Schleper, C., & Albers, S. V. (2008). UV-inducible cellular aggregation of the hyperthermophilic archaeon Sulfolobus solfataricus is mediated by pili formation. Molecular microbiology, 70(4), 938–952. https://doi.org/10.1111/j.1365-2958.2008.06459.x
- Pace, N. R. (May 2006). “Time for a change”. Nature. 441 (7091): 289. doi:10.1038/441289a