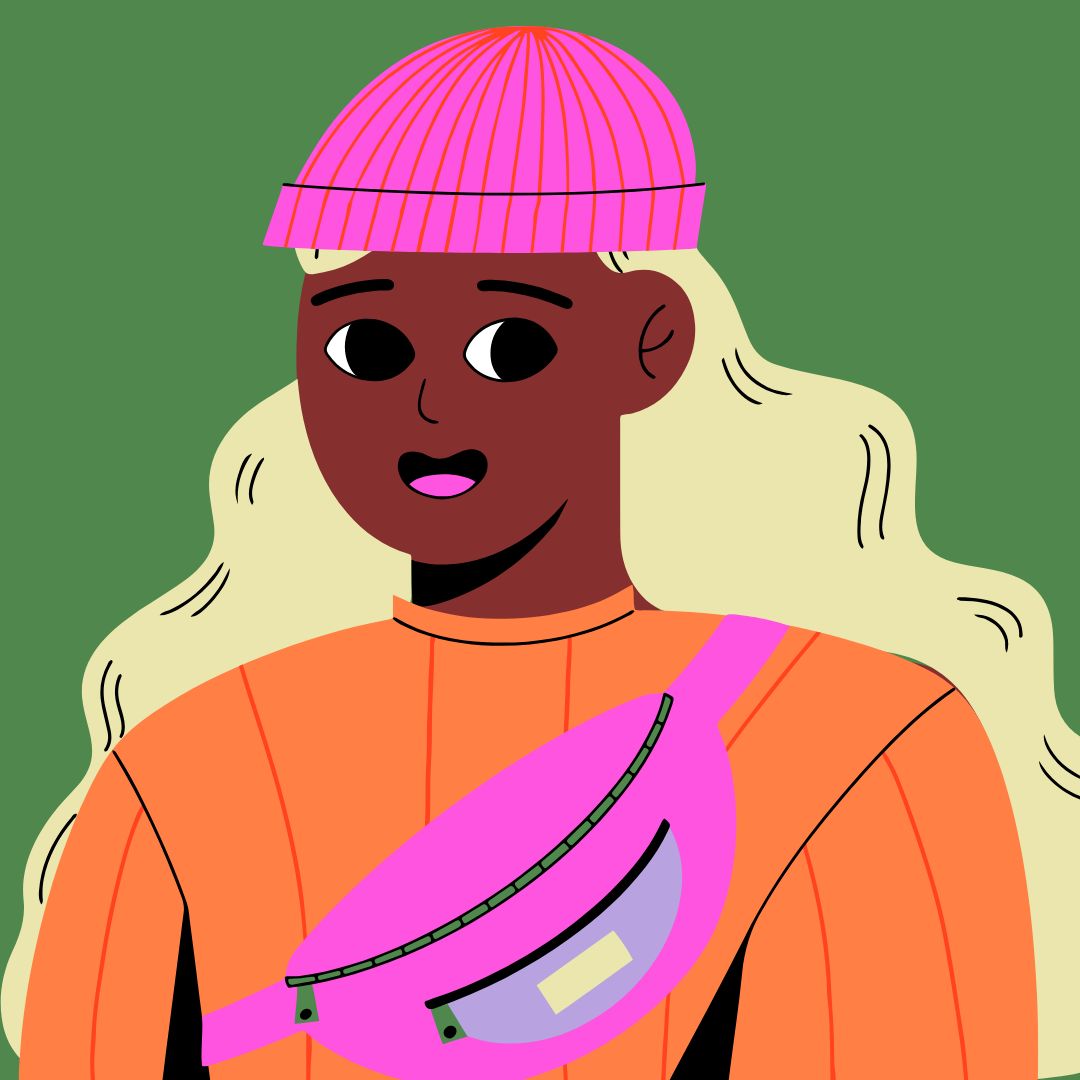
Sourav Pan
Transcript
The genetic basis of microevolution centers on changes in allele frequencies within a population over time.
But what exactly are alleles? Alleles are alternative versions or forms of the same gene.
Each gene can have multiple alleles that code for different versions of a trait. For example, a gene for flower color might have alleles for purple flowers or white flowers.
Let’s visualize a population of flowers. Initially, 70% of alleles in this population code for purple flowers, while 30% code for white flowers.
Over time, microevolution occurs as the frequency of alleles changes in the population. Various factors may cause white flowers to become more common.
We can visualize this change in allele frequencies over time. Note how the purple allele frequency decreases while the white allele frequency increases. This shift in allele frequencies is the essence of microevolution.
To summarize the genetic basis of microevolution: Microevolution occurs through changes in allele frequencies. Alleles are alternative forms of the same gene. These genetic changes lead to evolution at the population level and can occur over relatively short time periods.
Mutations are changes in DNA sequences that create new alleles in a population.
These genetic changes are the ultimate source of all genetic variation, providing the raw material for evolution.
Let’s explore the three main types of mutations that can occur in DNA.
In a substitution mutation, one nucleotide base is replaced with a different base. This is like replacing one letter in a word.
An insertion mutation occurs when one or more extra nucleotide bases are added to the DNA sequence. This shifts all subsequent bases.
In a deletion mutation, one or more nucleotide bases are removed from the DNA sequence. This also affects the reading of all bases that follow.
Mutations occur randomly during DNA replication and can have various effects on organisms.
Mutations can be beneficial, increasing an organism’s fitness or ability to survive.
Many mutations are neutral, having no significant effect on the organism.
And some mutations are harmful, potentially causing disease or reduced survival.
Over time, mutations create new alleles that can spread through a population, changing allele frequencies and driving evolution.
Remember that while mutations occur randomly, whether they persist in a population depends on natural selection and other evolutionary forces.
Mutations are changes in DNA sequence that can drastically affect an organism’s traits.
There are three main categories of factors that can cause genetic mutations.
Environmental factors include various forms of radiation and chemical compounds.
Let’s look at an example of how UV radiation damages DNA. Ultraviolet light can cause adjacent thymine bases to form abnormal chemical bonds.
These thymine dimers distort the DNA structure and can lead to errors during replication.
Biological factors like viruses can insert their genetic material into host DNA, causing mutations.
During DNA replication, errors can occur when DNA polymerase incorrectly pairs nucleotides.
Cells have evolved sophisticated repair mechanisms to fix DNA damage and replication errors.
However, despite these repair systems, some mutations still escape detection and become permanent changes in the DNA.
Artificial selection is the process where humans deliberately breed organisms to select for desired traits.
Unlike natural selection, which is driven by environmental pressures, artificial selection is directed by human choice, allowing for much faster changes in populations.
Let’s look at some examples of artificial selection that demonstrate human-directed evolution.
Dog breeds evolved from wolves into hundreds of distinct varieties in just thousands of years, showing dramatic variation in size, coat, and behavior.
Agricultural crops like cabbage have been transformed into diverse varieties including broccoli, cauliflower, and kale – all from the same wild ancestor.
Livestock species have been selectively bred for specific traits like milk production, meat yield, or egg-laying efficiency.
The speed of artificial selection is dramatically faster than natural selection. What might take hundreds of generations in nature can be achieved in just a few generations with controlled breeding.
Artificial selection is characterized by human-directed selection pressure, dramatically accelerated evolution, potentially maladaptive traits, and forms the foundation of modern agriculture and breeding programs.
Gene flow is the transfer of genetic material between populations that occurs through migration.
It happens when individuals or their reproductive cells, known as gametes, move from one population to another.
When individuals migrate from one population to another, they bring their genes with them.
Gene flow has two main effects. First, it increases genetic diversity within the receiving population.
Second, gene flow makes different populations more similar to each other over time, as their gene pools mix.
One common mechanism of gene flow is through gametes like pollen in wind-pollinated plants.
Wind can carry pollen over long distances, allowing genes to flow between plant populations that are far apart.
Animals migrating between populations also facilitate gene flow.
When animals move to a new population and reproduce, they introduce their genes to the new gene pool.
Over time, gene flow causes allele frequencies to become more similar between populations.
As individuals migrate between populations, the frequencies begin to converge toward intermediate values.
To summarize: Gene flow connects isolated populations through migration, occurs via movement of individuals or gametes, and homogenizes allele frequencies over time.
Horizontal gene transfer is the movement of genetic material between organisms outside of reproduction.
While most organisms inherit genes vertically from parents to offspring, horizontal gene transfer allows DNA to move between unrelated organisms.
The first mechanism is transformation, where bacteria can take up free DNA directly from their environment.
The second mechanism is conjugation, where bacteria form a physical connection called a pilus to directly transfer DNA from a donor to a recipient cell.
The third mechanism is transduction, where viruses called bacteriophages can accidentally package bacterial DNA and transfer it to another bacterium during infection.
Horizontal gene transfer also occurs in eukaryotes, though less frequently than in bacteria.
In eukaryotes, horizontal gene transfer can occur through endosymbionts, viral vectors, and other mechanisms. These rare events can contribute to evolutionary innovation.
Horizontal gene transfer can rapidly spread adaptive traits such as antibiotic resistance through bacterial populations.
When one bacterium develops resistance, it can share that resistance gene with many others through horizontal gene transfer.
This process can lead to widespread antibiotic resistance much faster than would be possible through vertical inheritance alone.
Viral evolution represents one of the most rapid forms of microevolution in nature.
Viruses evolve so rapidly due to three key characteristics.
First, viruses, especially RNA viruses, have extremely high mutation rates. This is because RNA viral replication lacks error-checking mechanisms found in DNA replication.
Second, viruses exist in enormous population sizes. A single infected person can harbor billions of viral particles, providing countless opportunities for mutations.
Third, viruses reproduce extremely quickly. Many viruses complete their entire replication cycle in just hours, allowing mutations to accumulate rapidly across generations.
The mutation rates of RNA viruses are dramatically higher than those of DNA viruses.
HIV and influenza, both RNA viruses, have particularly high mutation rates, making them difficult targets for our immune system and medical interventions.
Viral generation times are remarkably short compared to other organisms.
Let’s examine antigenic drift in influenza viruses. This is the gradual accumulation of mutations in the surface proteins of the virus.
In 2020, the virus has a particular set of surface proteins. Your immune system produces antibodies that recognize and bind to these proteins.
By 2021, mutations have slightly altered the surface proteins, but vaccines may still be partially effective.
By 2022, further mutations have significantly changed the virus’s surface proteins. Antibodies from earlier infections or vaccines may no longer recognize the virus effectively.
Rapid viral evolution creates several significant medical challenges.
Vaccines can quickly become ineffective as viruses mutate, particularly in their surface proteins.
Antiviral drugs may lose effectiveness as viruses evolve resistance mechanisms.
New viral variants may be more transmissible, more virulent, or better at evading the immune system.
This is why we need new influenza vaccines every year – to match the constantly evolving viral strains.
Over time, viral populations accumulate genetic diversity through mutations. This graph shows how genetic diversity increases in viral populations.
Influenza shows a steady increase in genetic diversity over time due to its high mutation rate and seasonal pressure.
HIV demonstrates an even steeper increase in genetic diversity due to its extremely high mutation rate and the selective pressure from the immune system and antiviral drugs.
Let’s compare microevolution and macroevolution, two different scales of evolutionary change.
Microevolution refers to small-scale changes within species over relatively short time periods, such as changes in allele frequencies within a population.
Macroevolution, on the other hand, involves large-scale changes leading to new species and higher taxonomic groups, typically occurring over much longer time periods.
These evolutionary processes operate on very different time scales, from days to millions of years.
Microevolutionary processes include changes in allele frequencies, natural selection within populations, genetic drift, and adaptations to local environments.
Macroevolutionary processes include speciation, which is the formation of new species, adaptive radiation where species diversify rapidly, major evolutionary transitions, and extinction events.
Microevolutionary changes can accumulate over time, potentially leading to macroevolutionary patterns.
To summarize, microevolution and macroevolution represent different scales of the same evolutionary processes. While they occur on different timescales, microevolutionary changes can accumulate over time to produce macroevolutionary patterns.
The relationship between microevolution and macroevolution represents one of the most important concepts in evolutionary biology.
Microevolution involves small-scale changes in allele frequencies within populations, while macroevolution encompasses large-scale changes that lead to the formation of new species. Though these processes operate on different time scales, they are fundamentally connected.
Microevolution occurs over generations, population divergence over thousands of years, and macroevolution over millions of years.
This branching diagram illustrates how microevolution can lead to macroevolution over time. First, genetic changes accumulate within an ancestral population.
When the population splits and experiences different environmental pressures or genetic drift, populations begin to diverge.
Eventually, enough differences accumulate that the populations can no longer interbreed, resulting in the formation of new species – a macroevolutionary change.
Several key mechanisms bridge microevolution and macroevolution.
First, small genetic mutations accumulate over time. Geographic isolation allows populations to evolve independently. Different selection pressures in various environments favor different traits.
Genetic drift can cause significant random differences between populations. Finally, reproductive isolation marks the point of speciation, when populations can no longer interbreed.
Darwin’s finches provide a classic example of how microevolution leads to macroevolution.
At the microevolutionary level, finch beak sizes change in response to seed availability. These accumulated changes have led to macroevolutionary outcomes: more than fourteen distinct species evolved from a single ancestral population.
To summarize the relationship between micro and macroevolution: Microevolution provides the raw material for macroevolution. The distinction is mainly one of time scale and outcome, with the same evolutionary mechanisms operating at both levels. Small changes accumulate over time to create large-scale differences between species.
Modern tools have revolutionized how scientists study microevolution, allowing us to see genetic changes at unprecedented detail.
These advanced techniques include next-generation DNA sequencing, whole genome analysis, and bioinformatics.
DNA sequencing technology has evolved dramatically. In the 1980s, Sanger sequencing could read short DNA fragments.
By the 2000s, next-generation sequencing increased throughput, and modern techniques can sequence millions of base pairs rapidly.
Genomic analysis allows scientists to compare DNA sequences across populations, identifying mutations and tracking microevolutionary changes.
These genetic tools let researchers track changes in allele frequencies over time across different populations, revealing evolutionary responses to selection pressures.
The data can show how quickly adaptive mutations spread or how genetic drift affects isolated groups.
One landmark study is the E. coli Long-Term Evolution Experiment, where researchers have tracked bacterial evolution for over sixty thousand generations.
The Human Genetic Diversity Project has used genomic tools to map human migrations, identify selective adaptations, and reveal recent evolutionary changes in human populations.
The field continues to advance with emerging technologies like single-cell genomics, CRISPR-based lineage tracing, and environmental DNA analysis.
These tools are making it possible to observe microevolution in real-time and at unprecedented scales.
Microevolution has significant practical implications for many human challenges.
These challenges span medical, agricultural, conservation, and climate adaptation domains.
In medicine, antibiotic resistance is a prime example of microevolution. Bacteria rapidly evolve resistance to drugs, creating a significant public health challenge.
Bacteria can evolve resistance in just a few generations. Multiple drug resistance is increasing, while new antibiotic development struggles to keep pace. Evolutionary medicine proposes solutions based on evolutionary principles.
Antibiotic stewardship programs that carefully manage antibiotic use can slow the evolution of resistance by reducing selection pressure.
In agriculture, pests rapidly evolve resistance to pesticides, reducing crop yields and threatening food security.
More than 600 insect species now show pesticide resistance. Crop losses cost billions of dollars annually. Agricultural ecosystems create strong selection pressures, and single-approach pest control inevitably fails due to evolution.
Integrated Pest Management or IPM combines multiple approaches to minimize selection pressures, working with evolutionary principles rather than against them.
Conservation biology relies on understanding microevolution to protect endangered species and maintain genetic diversity.
Small populations lose genetic diversity quickly. Inbreeding depression reduces fitness in endangered species. Genetic rescue can restore diversity, and a species’ potential to adapt depends on its genetic variation.
Conservation strategies like habitat corridor networks maintain gene flow between populations, preserving genetic diversity and adaptive potential.
As climate change accelerates, species must adapt quickly or face extinction. Microevolution will determine which species survive.
Rapid environmental changes often exceed natural adaptation rates. Species with short generation times adapt faster. Range shifts follow temperature gradients, and assisted migration may help some species survive climate change.
Evolutionary rescue programs preserve genetic diversity and promote adaptation to changing conditions, giving species a better chance of survival.
By applying evolutionary principles to human challenges, we can develop more effective and sustainable strategies.
Study Materials
Microevolution - Definition, Causes, Examples
Helpful: 0%
Related Videos
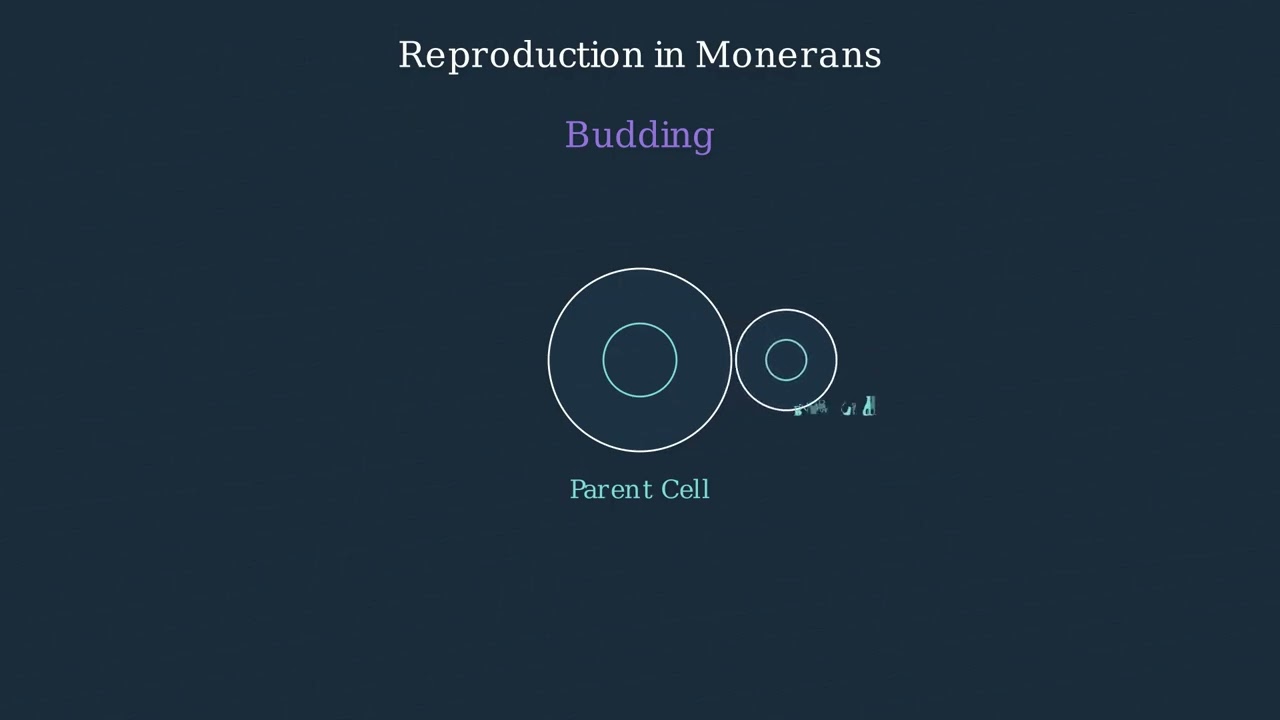
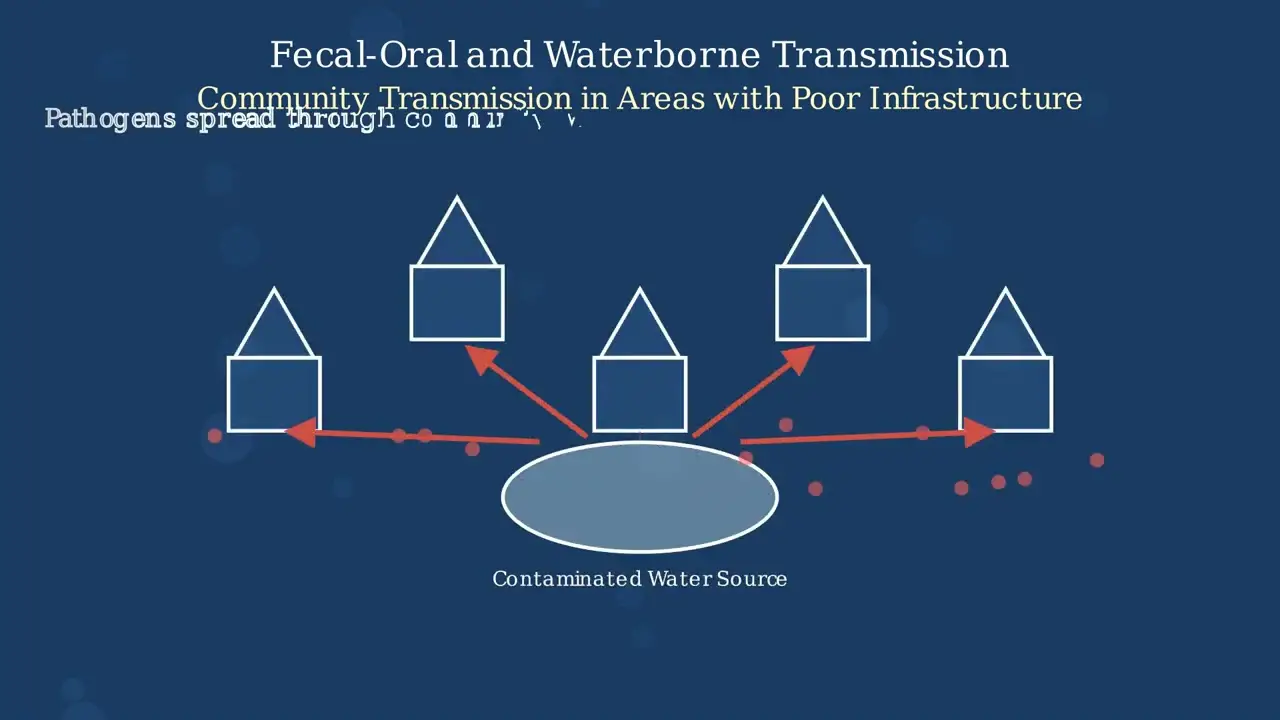
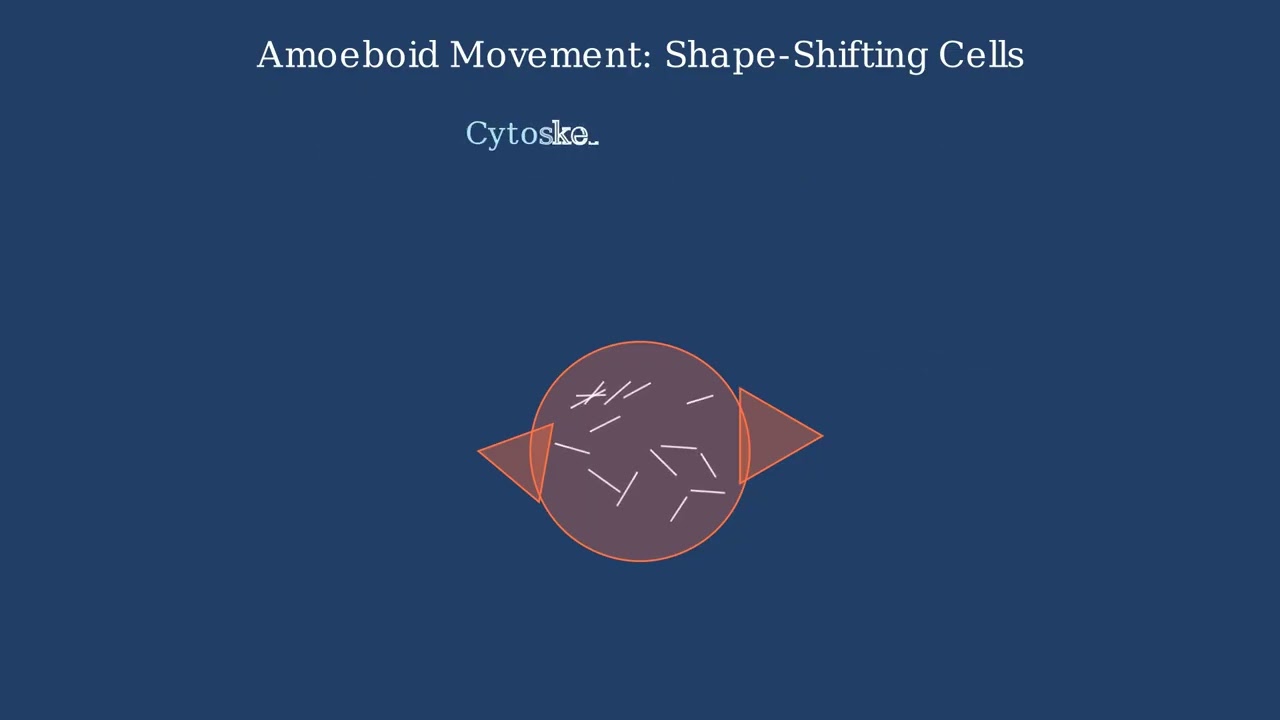
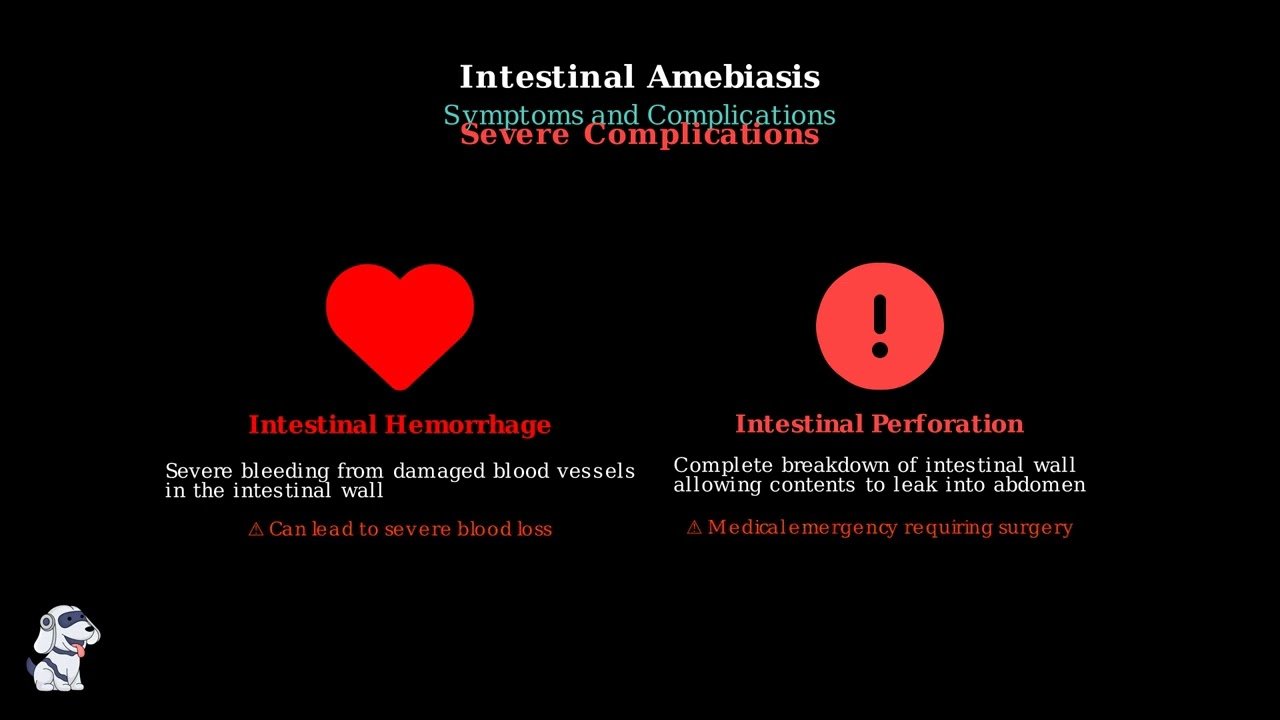
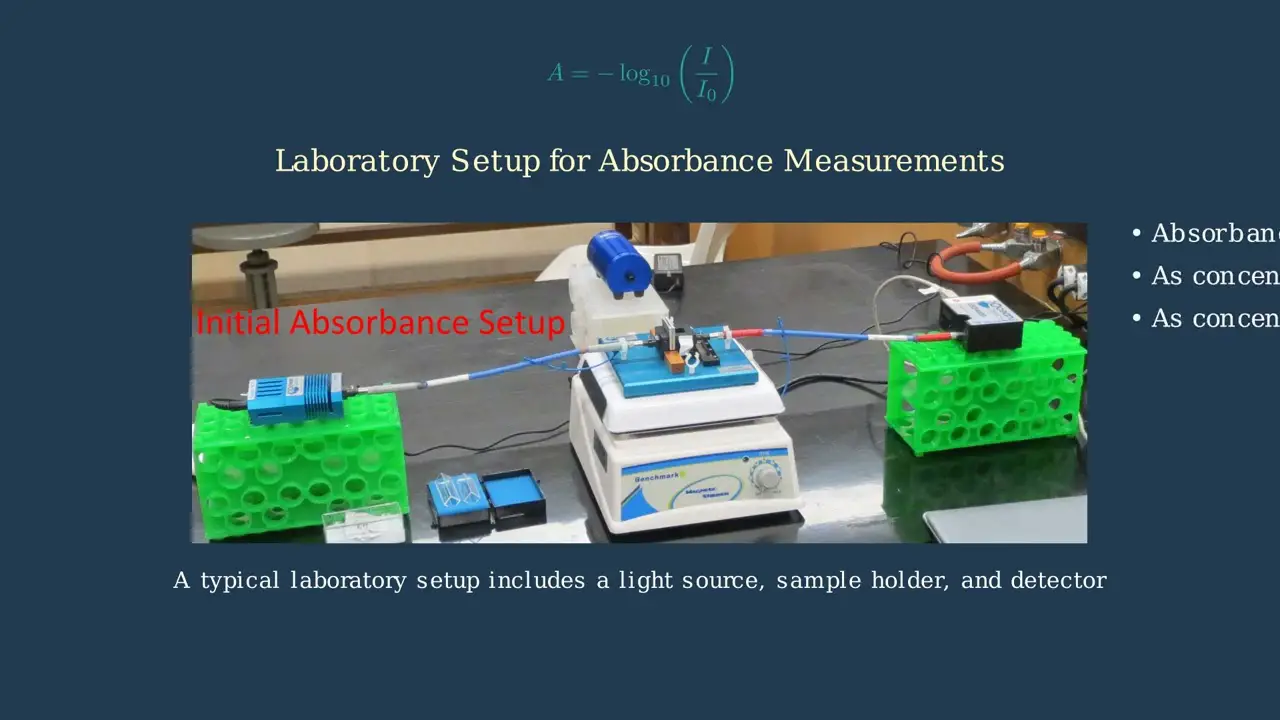
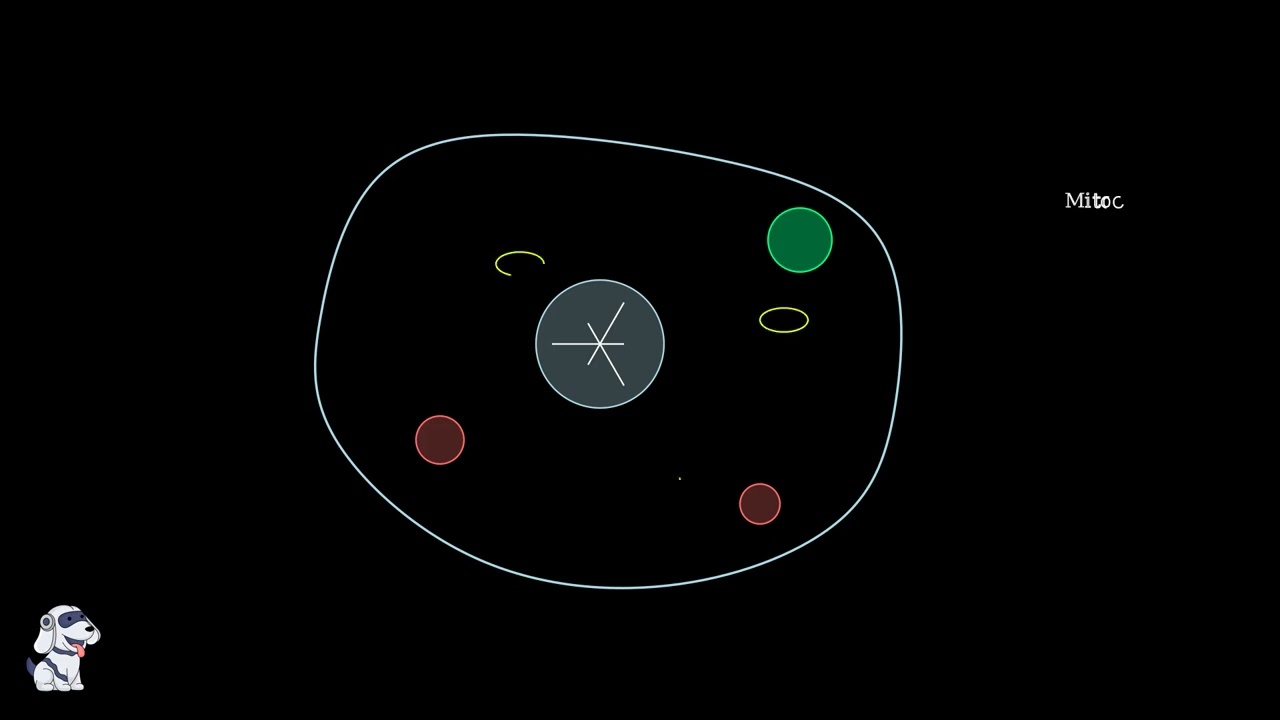
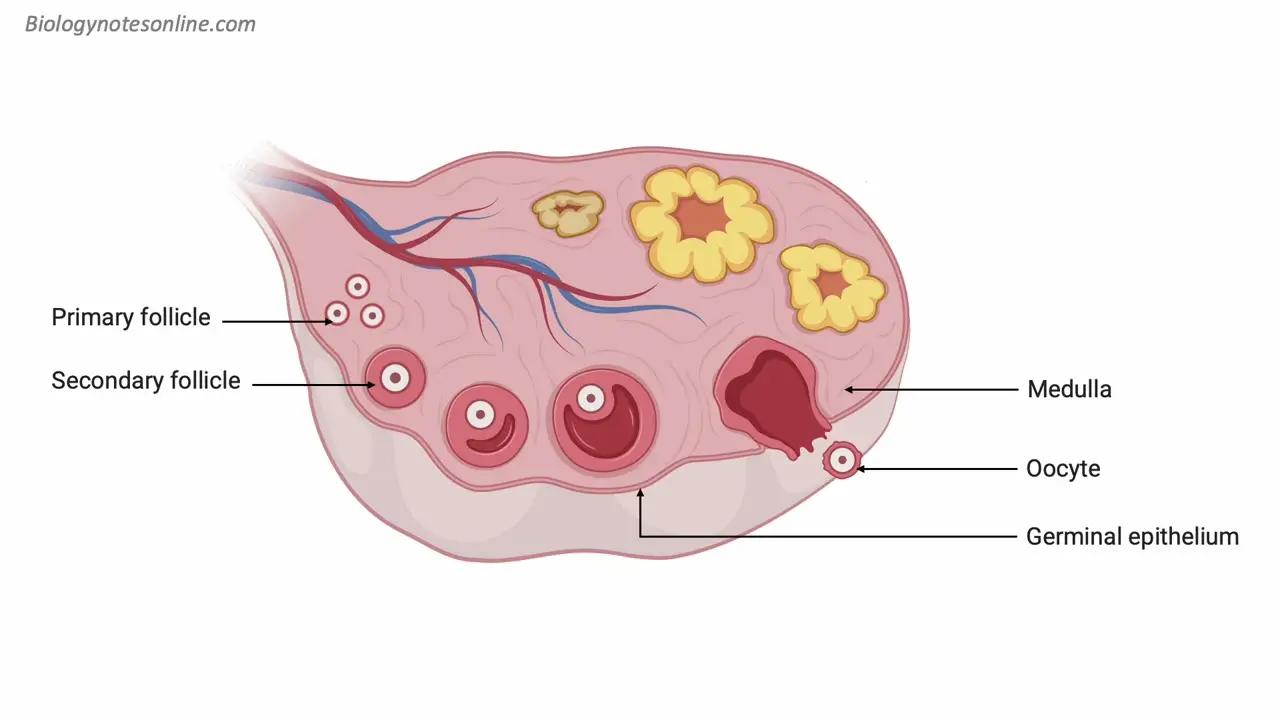
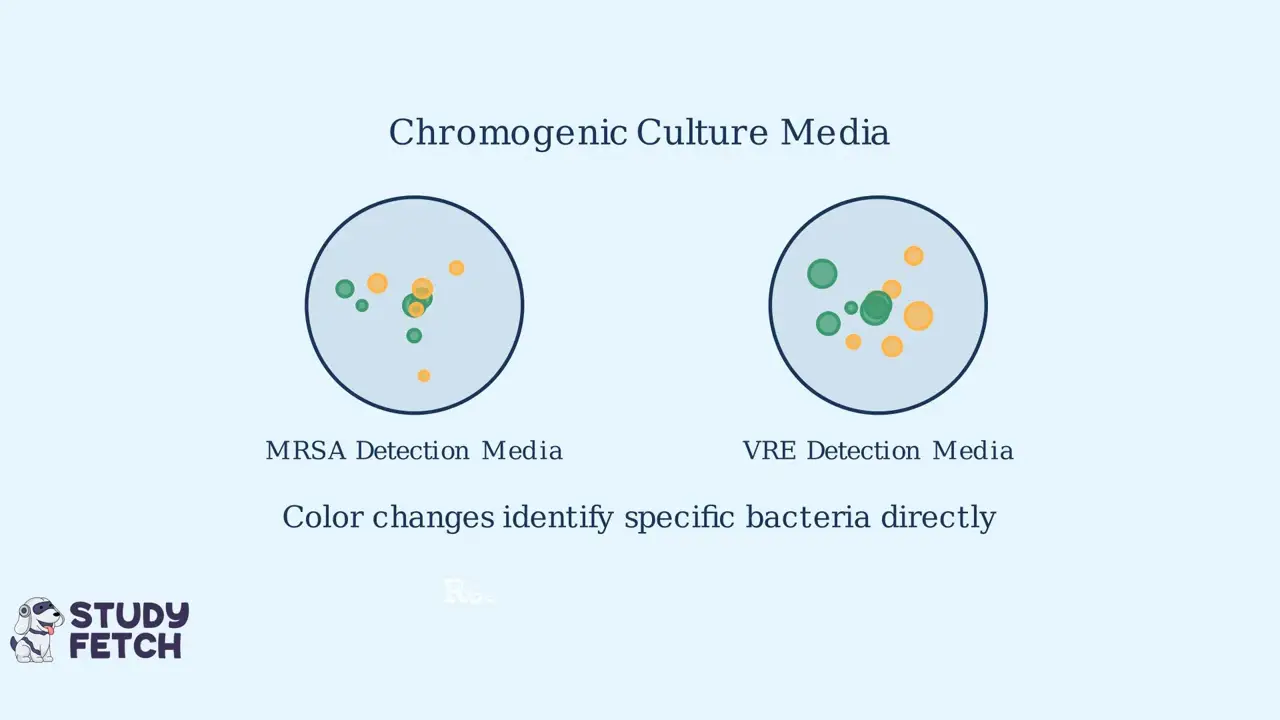
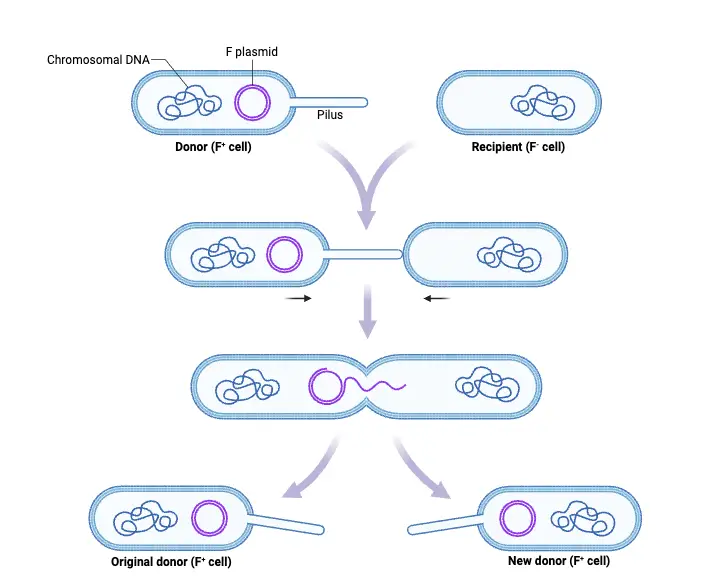
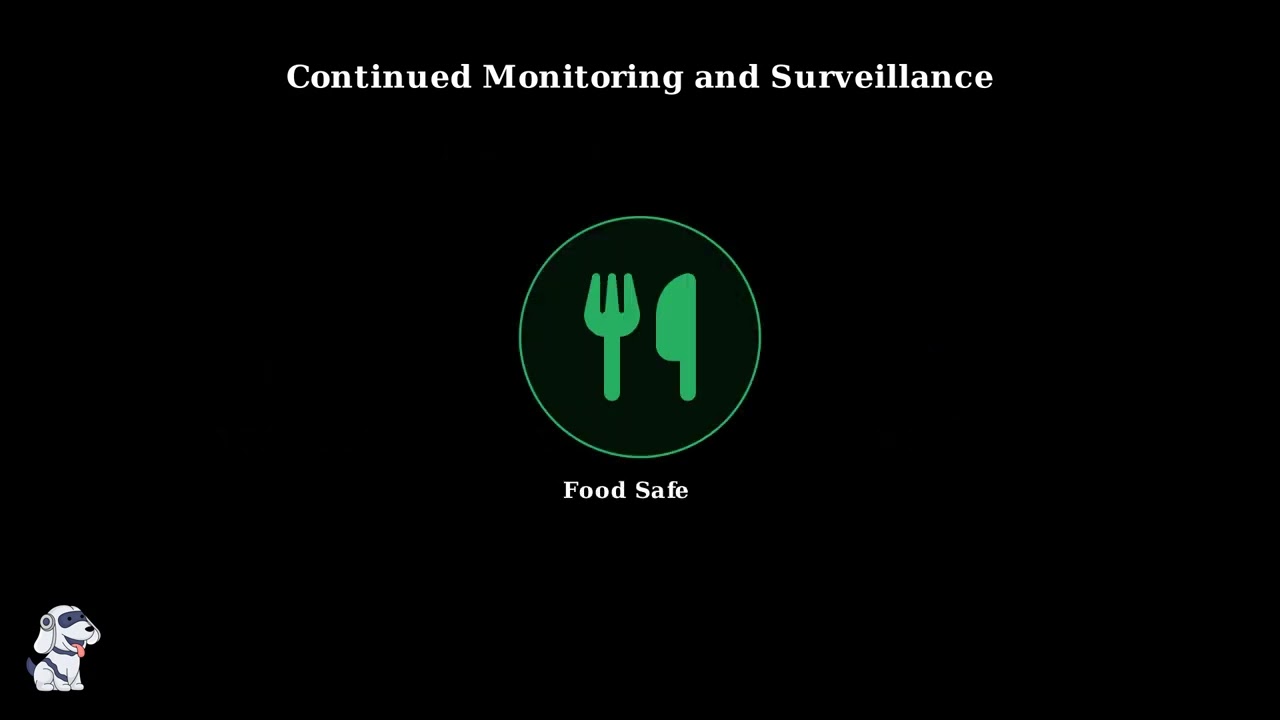