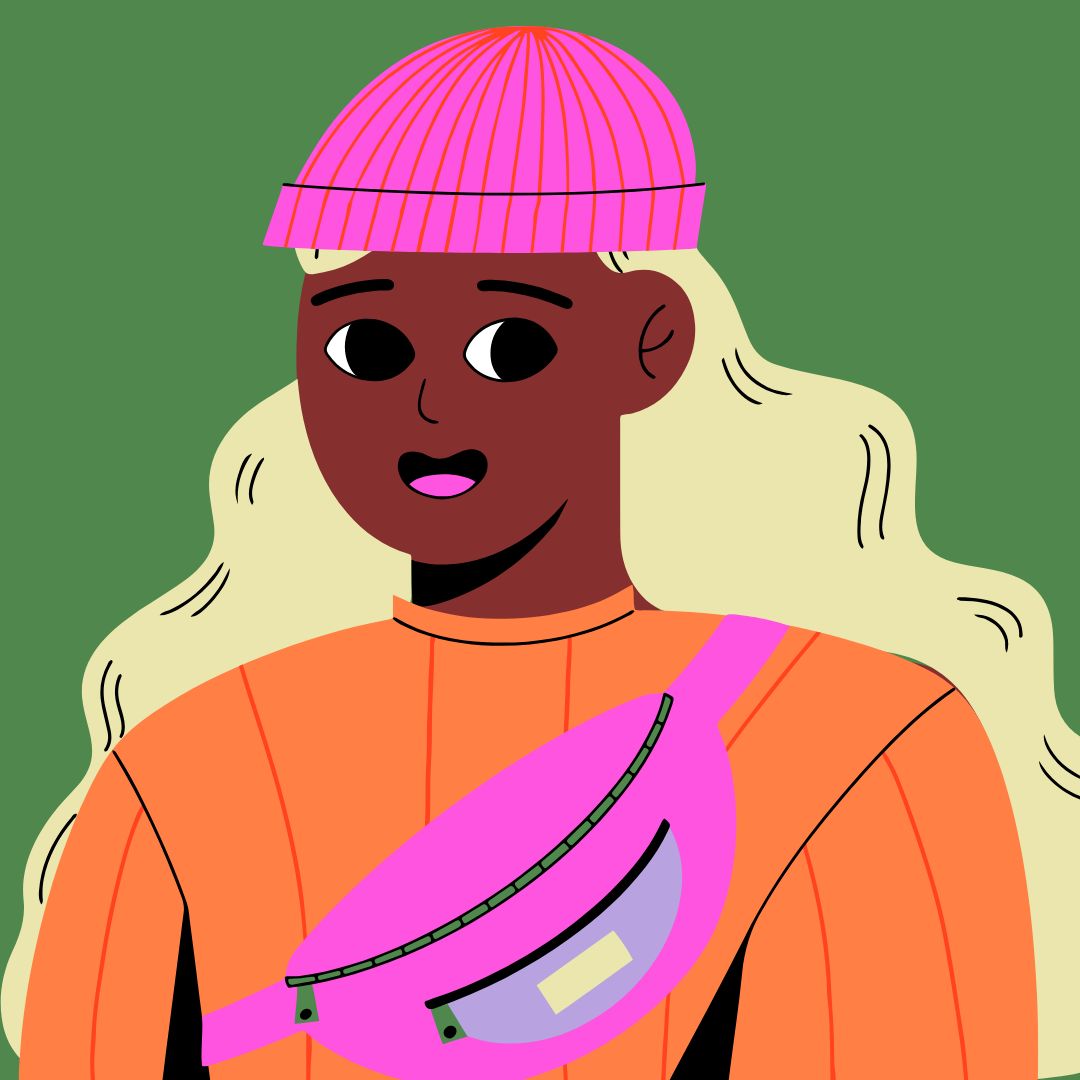
Sourav Pan
Transcript
What is a heterotroph? Let’s explore this fundamental concept in biology.
Heterotrophs are organisms that cannot produce their own food. This is their defining characteristic.
To understand heterotrophs better, let’s compare them with autotrophs.
Heterotrophs cannot produce their own food, must consume organic matter, and obtain energy from other organisms.
In contrast, autotrophs can produce their own food using sunlight or chemicals, making them self-sufficient for energy.
Examples of heterotrophs include animals, fungi, and many bacteria, while autotrophs include plants, algae, and some bacteria like cyanobacteria.
Heterotrophs play crucial roles in food chains and energy flow throughout ecosystems.
Energy flows from producers, which are autotrophs like plants, to primary consumers, which are heterotrophs like herbivores.
Then to secondary consumers like carnivores, which are also heterotrophs.
Finally, decomposers, another type of heterotroph, break down dead organisms and return nutrients to the soil.
Let’s summarize the key points about how heterotrophs acquire energy and nutrients.
Unlike autotrophs, heterotrophs cannot convert sunlight or inorganic sources into energy. They must rely on breaking down complex organic compounds obtained from other organisms.
They obtain carbon from organic sources and serve as essential links in the flow of energy through ecosystems.
Holozoic nutrition is a form of heterotrophic nutrition where organisms ingest solid food particles and digest them internally.
Unlike autotrophs, heterotrophs that use holozoic nutrition cannot synthesize their own food and must consume other organisms.
Holozoic nutrition involves five distinct stages that work together to process food.
Stage one is ingestion, where food is taken in through the mouth or specialized feeding structures.
Stage two is digestion, where complex food molecules are broken down into simpler, absorbable nutrients through mechanical and chemical processes.
Stage three is absorption, where digested nutrients move from the digestive tract into the bloodstream or body fluids.
Stage four is assimilation, where the absorbed nutrients are used for energy production, growth, and cell repair.
Stage five is egestion, where undigested and waste materials are eliminated from the body.
Let’s look at some examples of animals that use holozoic nutrition.
Humans have an omnivorous digestive system with a long digestive tract that can process both plant and animal material.
Dogs have a primarily carnivorous digestive system with a shorter intestinal tract optimized for digesting meat.
Birds have specialized digestive adaptations including a crop for food storage and a muscular gizzard for grinding food items.
Let’s review some key points about holozoic nutrition.
Digestive systems vary greatly among organisms based on diet and evolutionary adaptations.
All five stages—ingestion, digestion, absorption, assimilation, and egestion—are essential for complete nutrient extraction.
Different animals have specialized digestive structures based on whether they’re herbivores, carnivores, or omnivores.
Holozoic nutrition is found in most animals, many protozoans, and some fungi.
Herbivores are heterotrophs that obtain energy and nutrients exclusively from plant materials.
They occupy a critical position in food chains as primary consumers, directly connecting plant producers to higher-level consumers.
Herbivores have evolved specialized adaptations to efficiently process plant material, which is often difficult to digest.
Most herbivores have specialized flat teeth with wide surfaces for grinding tough plant material. These teeth help break down cell walls and increase the surface area for digestive enzymes.
Herbivores typically have longer and more complex digestive systems than carnivores. Ruminants like cows have multi-chambered stomachs that allow for fermentation and extended digestion of plant matter.
Many herbivores rely on symbiotic relationships with microorganisms in their digestive tracts. These microbes produce enzymes that break down cellulose, a major component of plant cell walls that most animals cannot digest on their own.
Common examples of herbivores include cows, which are ruminants with four-chambered stomachs, rabbits, which use hindgut fermentation, and elephants, which process massive quantities of plant material daily.
Ecologically, herbivores play a crucial role as primary consumers. They convert plant energy into animal biomass, making it available to higher trophic levels in the ecosystem.
Herbivores also help control plant populations through grazing, maintain ecosystem balance, and provide a critical food source for carnivores and omnivores.
Detritivores are essential heterotrophs that play a crucial role in recycling nutrients in ecosystems.
Detritivores are organisms that feed on dead organic matter, also known as detritus. This includes fallen leaves, dead animals, and other organic waste materials.
Unlike decomposers, which break down dead matter externally using enzymes, detritivores physically consume and digest detritus. Both groups are vital components in nutrient recycling.
Common examples of detritivores include earthworms, millipedes, and various crustaceans like crabs.
Detritivores play a critical role in nutrient cycling by converting dead organic matter back into usable nutrients that can be absorbed by plants.
They form a vital link in the nutrient cycle. Plants are consumed by animals, which eventually die and become detritus. Detritivores then process this material, returning nutrients to the soil for plants to use again.
Detritivores provide numerous benefits to ecosystems. They accelerate decomposition, improve soil quality through aeration, support plant growth by releasing nutrients, and prevent the accumulation of waste materials.
As nature’s recyclers, detritivores ensure the continuous flow of nutrients in ecosystems, demonstrating yet another fascinating adaptation among heterotrophic organisms.
Photoheterotrophic bacteria represent a fascinating intersection between heterotrophs and autotrophs.
These unique microorganisms use light for energy, similar to plants, but cannot fix carbon dioxide, requiring organic carbon sources like other heterotrophs.
They exhibit several key characteristics that distinguish them from other bacteria. They harness light energy through phototrophy, but unlike autotrophs, they cannot fix carbon dioxide. Instead, they require organic carbon compounds for growth, demonstrating their heterotrophic nature. This dual metabolic approach is made possible by specialized pigments called bacteriochlorophylls.
Bacteriochlorophylls are specialized pigment molecules that enable these bacteria to capture light energy. Unlike plant chlorophylls, bacteriochlorophylls can absorb light in the near-infrared spectrum and function in anaerobic conditions.
The photosystem of these bacteria consists of a light-harvesting complex that captures photons and transfers the energy to a reaction center. This generates a flow of electrons and establishes a proton gradient that drives ATP synthesis.
Two major groups of photoheterotrophic bacteria are the purple non-sulfur bacteria and green non-sulfur bacteria. Purple non-sulfur bacteria, like Rhodobacter and Rhodopseudomonas, can use various organic compounds such as acetate. They can grow in anaerobic conditions with light, or aerobically in darkness. Green non-sulfur bacteria, including Chloroflexus and Oscillochloris, metabolize organic acids and sugars, and are commonly found in hot springs and microbial mats.
To understand where photoheterotrophs fit in the microbial world, let’s compare them with photoautotrophs. Both use light as their energy source, but they differ in their carbon source. While photoautotrophs can fix carbon dioxide from the atmosphere, photoheterotrophs must obtain organic carbon compounds from their environment.
Photoheterotrophic bacteria demonstrate nature’s remarkable metabolic diversity. Their ability to use light for energy while requiring organic carbon illustrates the fascinating evolutionary adaptations of microorganisms to various ecological niches.
Parasitic plants are unusual heterotrophs in the plant kingdom that have evolved to extract nutrients from other plants.
Unlike typical plants that photosynthesize, these heterotrophs extract water, minerals, and organic compounds directly from their host plants.
Through evolution, parasitic plants have reduced or completely lost their photosynthetic capabilities. They’ve developed specialized feeding structures while some species maintain partial photosynthesis.
The key adaptation in parasitic plants is the haustorium, a specialized feeding structure that penetrates the host’s tissues to extract nutrients.
Common examples include dodder, which appears as yellow or orange thread-like stems wrapping around host plants, mistletoe growing on tree branches, and broomrape emerging from host roots.
Looking closely at how parasitic plants connect to their hosts, the haustorium penetrates through the host tissue to reach the vascular system, tapping into both the xylem and phloem to extract water, minerals, and carbohydrates.
Parasitic plants can be classified based on their dependence on hosts. Holoparasites are completely dependent, while hemiparasites maintain some photosynthetic activity. They can also be obligate or facultative parasites, depending on whether they can survive without a host.
Saprophytic plants are a fascinating group of heterotrophs in the plant kingdom.
Unlike most plants, saprophytic plants have lost their chlorophyll and cannot photosynthesize. Instead, they obtain nutrients from decaying organic matter in the forest floor.
These unusual plants evolved from autotrophic ancestors, adapting to life in low-light forest environments by developing specialized relationships with fungi.
Their nutritional mechanism is particularly interesting.
Saprophytic plants form mycoheterotrophic relationships with fungi. These fungi connect to tree roots, forming mycorrhizal networks, allowing the plants to effectively steal nutrients that the fungi obtain from trees.
The Indian Pipe, scientifically known as Monotropa uniflora, is a classic example. It has a distinctive pure white, waxy appearance due to its complete lack of chlorophyll, and is typically found in dense, shady forests.
Another example is the Ghost Plant, or Monotropa hypopitys, which exhibits a yellowish or pinkish coloration. Unlike the Indian Pipe, it produces multiple flowers on a single stem and is often found in coniferous forests.
These specialized plants play unique roles in forest ecosystems.
Saprophytic plants inhabit specific forest niches and can serve as indicators of mycorrhizal fungal health. They form part of the complex nutrient networks that exist beneath the forest floor.
In the forest ecosystem, trees provide carbon to mycorrhizal fungi through their roots. The saprophytic plants then tap into this fungal network to extract the nutrients they need, without directly contributing to the photosynthetic process.
Through this specialized nutritional strategy, saprophytic plants have carved out a unique niche in forest ecosystems, demonstrating the remarkable diversity of heterotrophic adaptations in the plant kingdom.
Energy transfer in heterotrophs follows specific processes that determine how organisms obtain and use energy.
Heterotrophs transfer energy through four main processes: ingestion, where food is taken in; digestion, where it’s broken down; absorption, where nutrients enter the cells; and cellular respiration, where energy is extracted.
Energy transfer between trophic levels is remarkably inefficient, with only about 10 percent of energy successfully passing from one level to the next.
This inefficiency occurs for several reasons: much energy is lost as heat during metabolism, used for movement, directed to bodily functions, and some biomass is never consumed.
At the cellular level, energy is extracted through cellular respiration. When glucose is broken down, only about 40 percent of its energy is captured as ATP, the cell’s energy currency.
The remaining energy is lost as heat and used to form waste products like carbon dioxide, illustrating why energy transfer can never be 100 percent efficient.
Heterotrophs play critical roles in maintaining ecological balance within ecosystems.
Predator-prey relationships are fundamental to ecological balance. When prey populations increase, predator populations follow, creating a natural balancing effect.
Trophic cascades occur when changes at one level of a food web ripple down through other levels. For example, when wolves are removed from an ecosystem, deer populations can explode, leading to overgrazing of plants.
Keystone species are heterotrophs that have a disproportionately large impact on their ecosystem. Sea otters are a classic example – they control sea urchin populations, which in turn prevents the destruction of kelp forests.
When non-native heterotrophs are introduced to ecosystems, they can severely disrupt ecological balance. In Australia, cane toads poison native predators, leading to population explosions of the prey species these predators would normally control.
Heterotrophs play a crucial role in nutrient cycling. As they consume organic matter and excrete waste, they make essential elements available for producers. Decomposers like fungi and bacteria complete this cycle by breaking down dead organisms.
Study Materials
No study materials available for this video.
Helpful: 0%
Related Videos
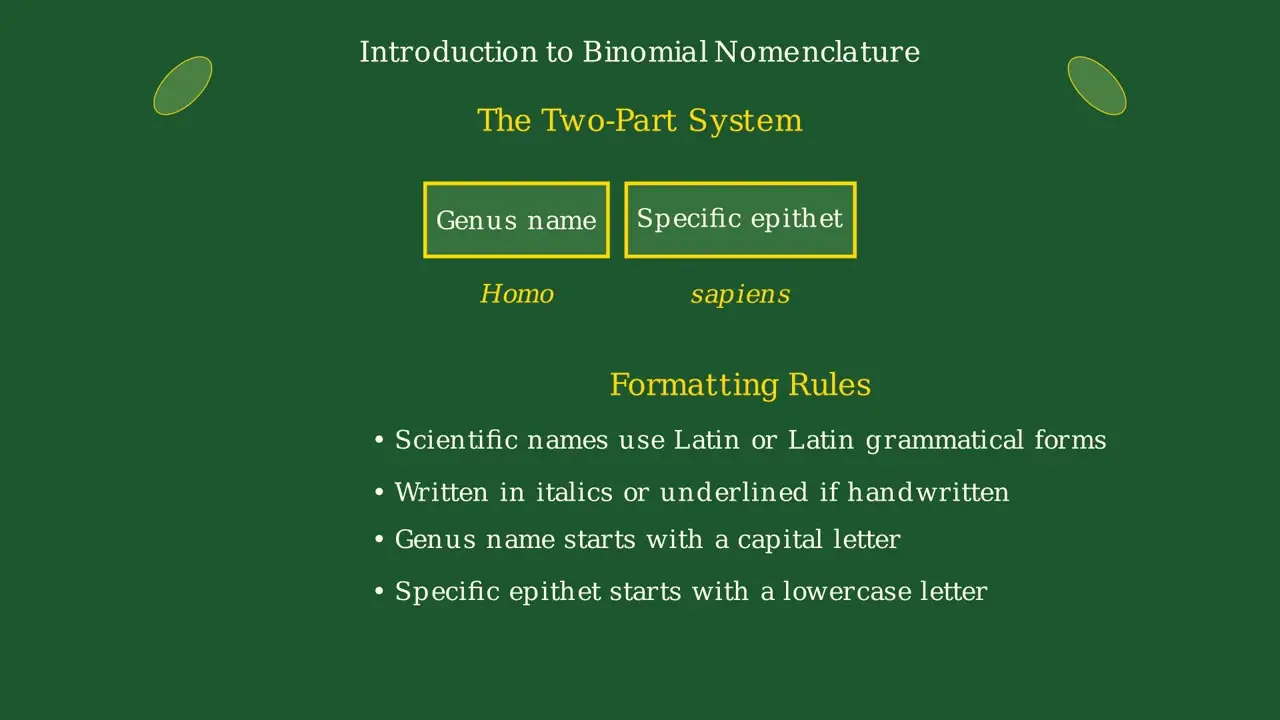
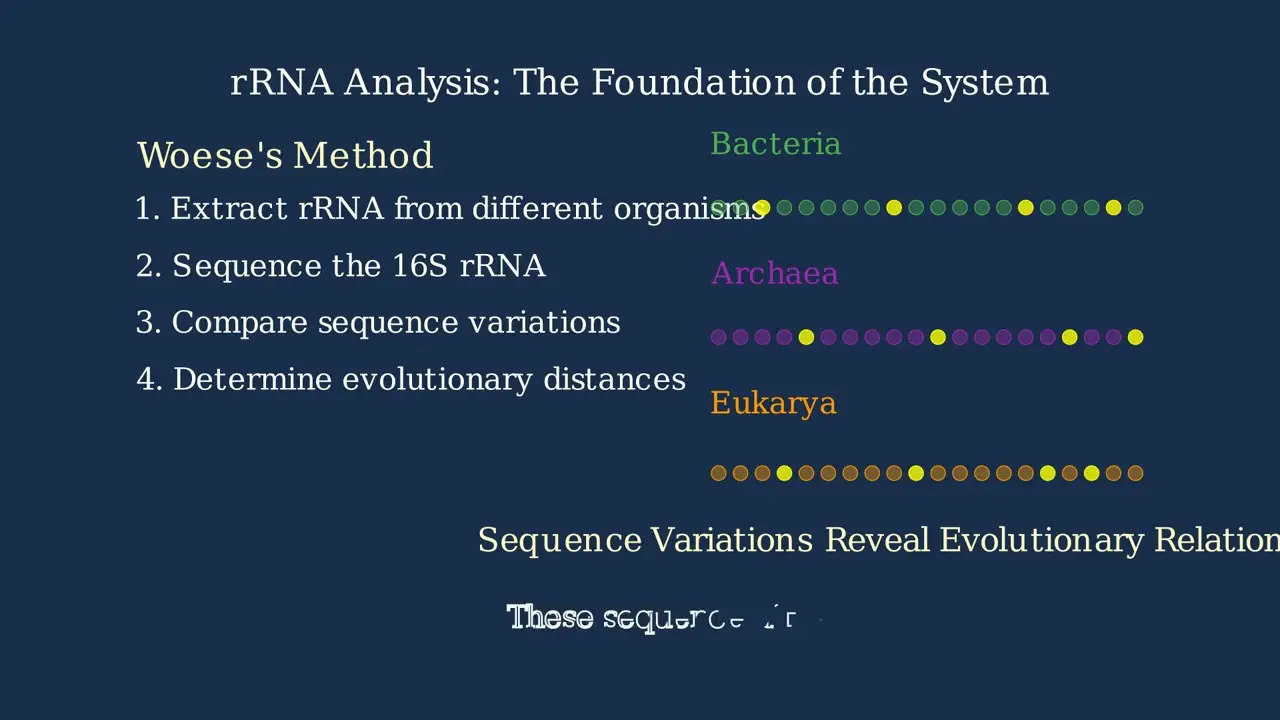
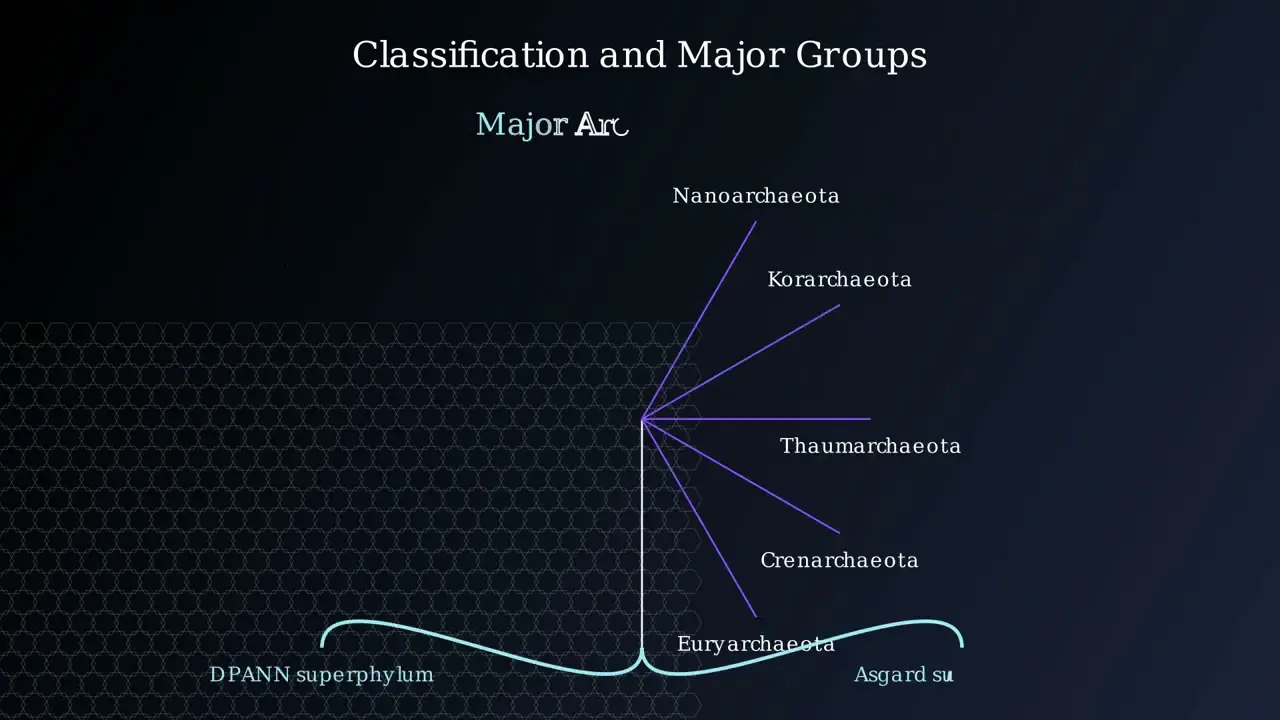
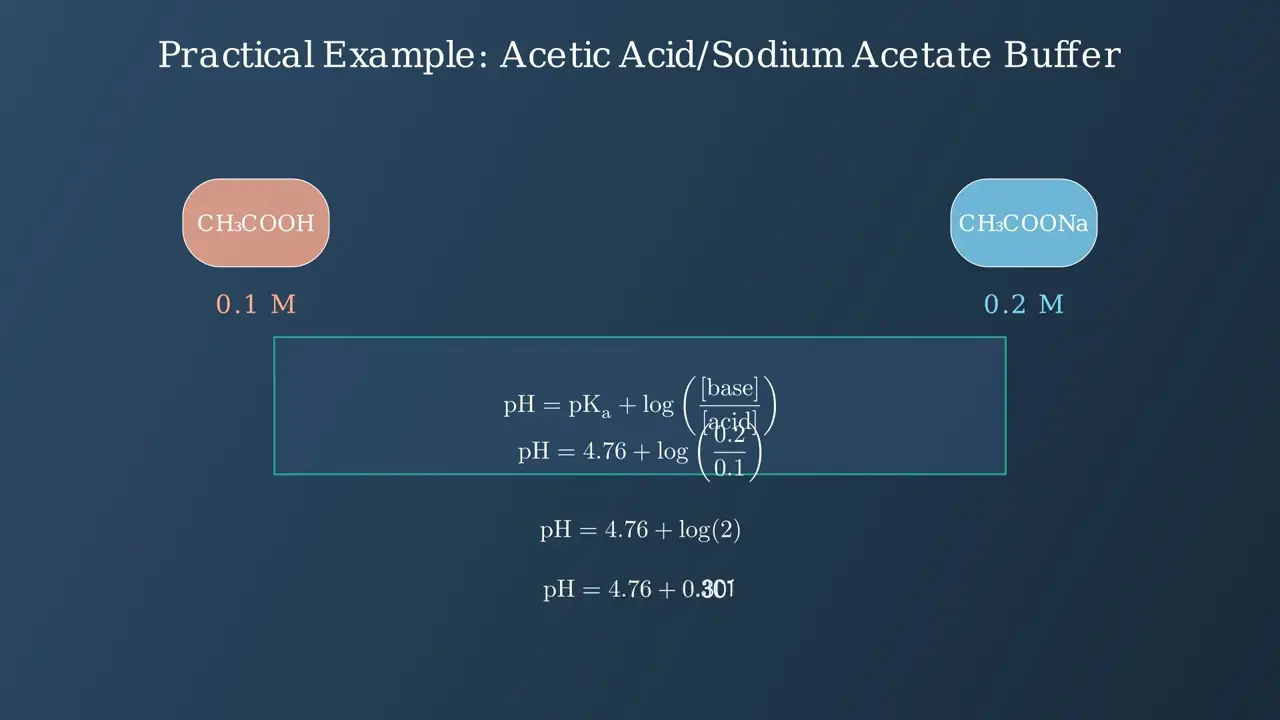
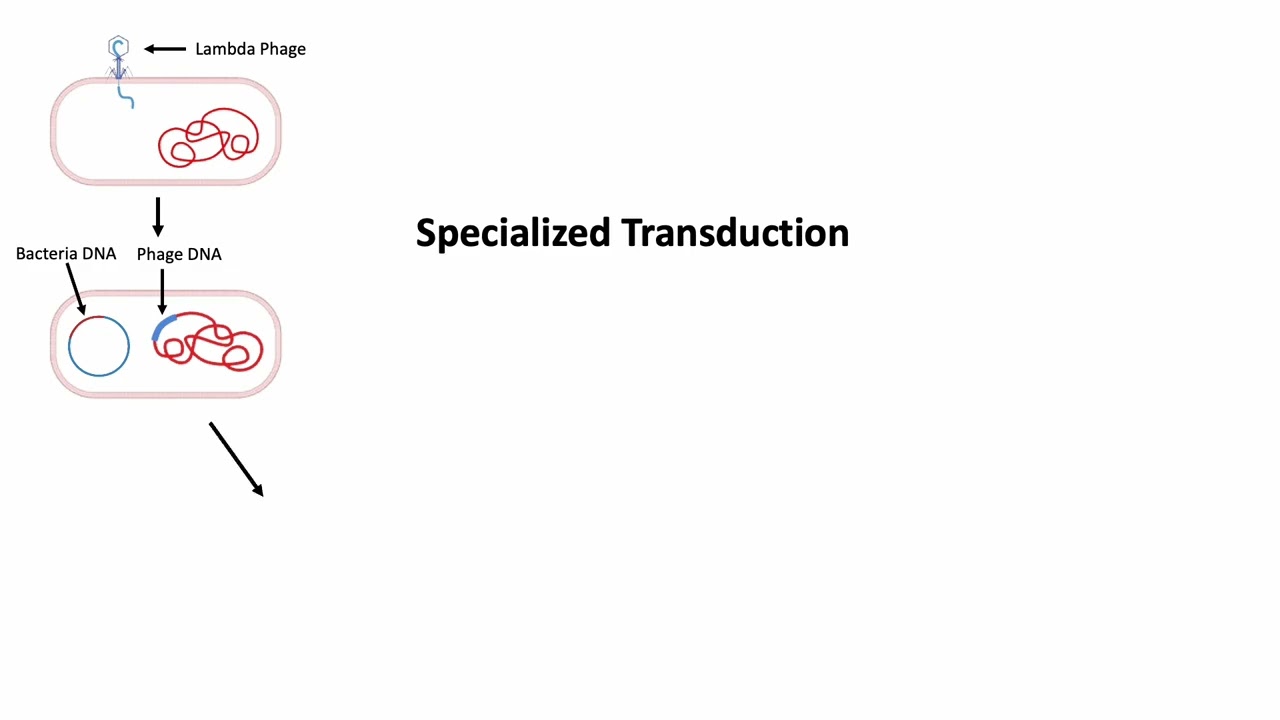
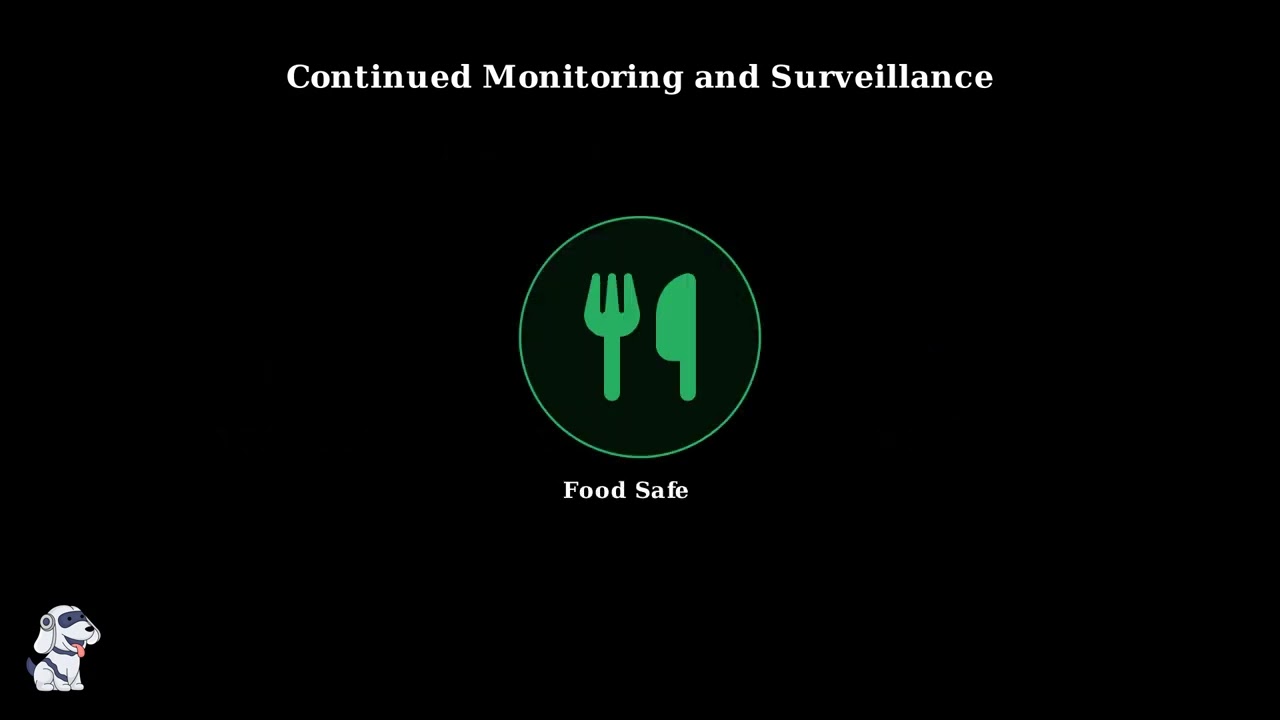
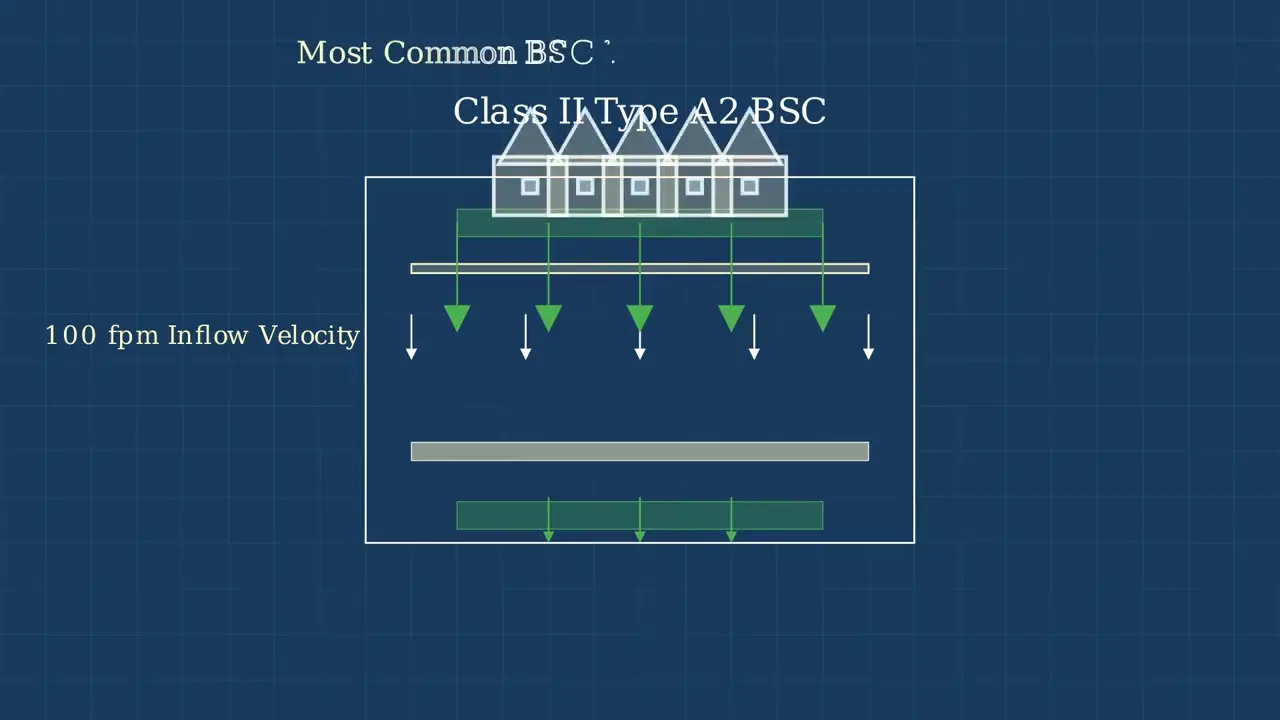
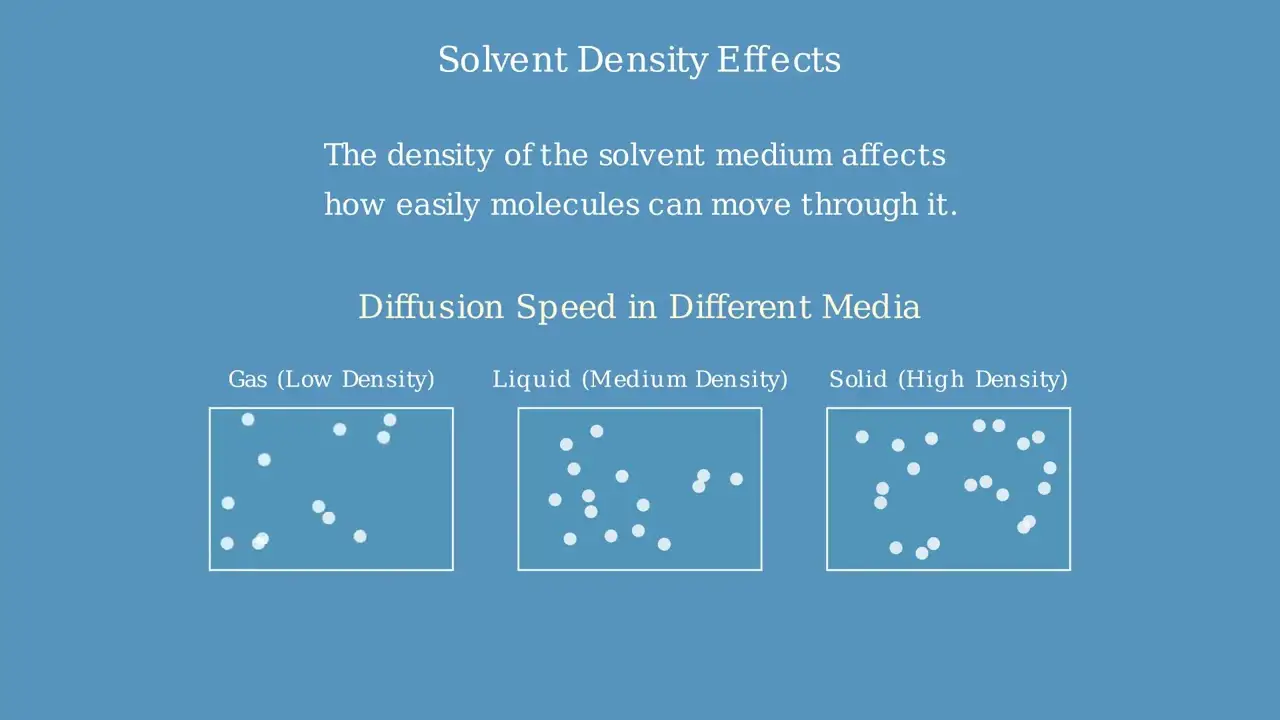
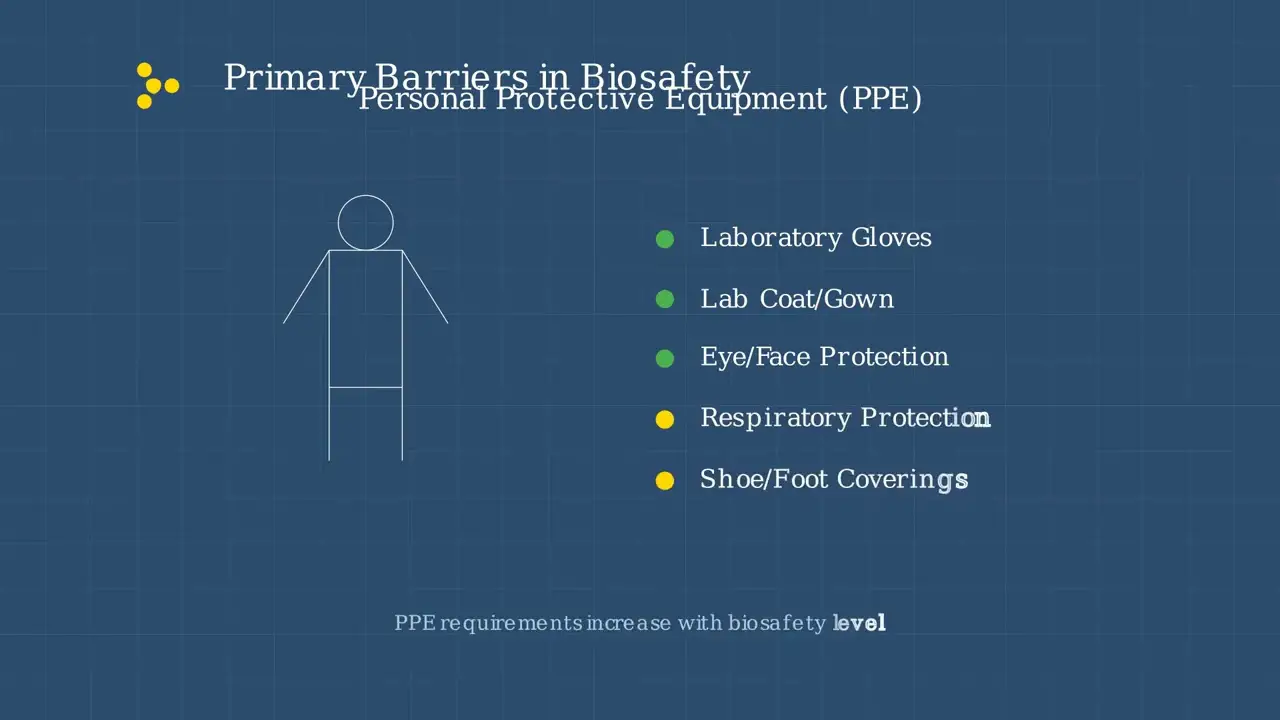