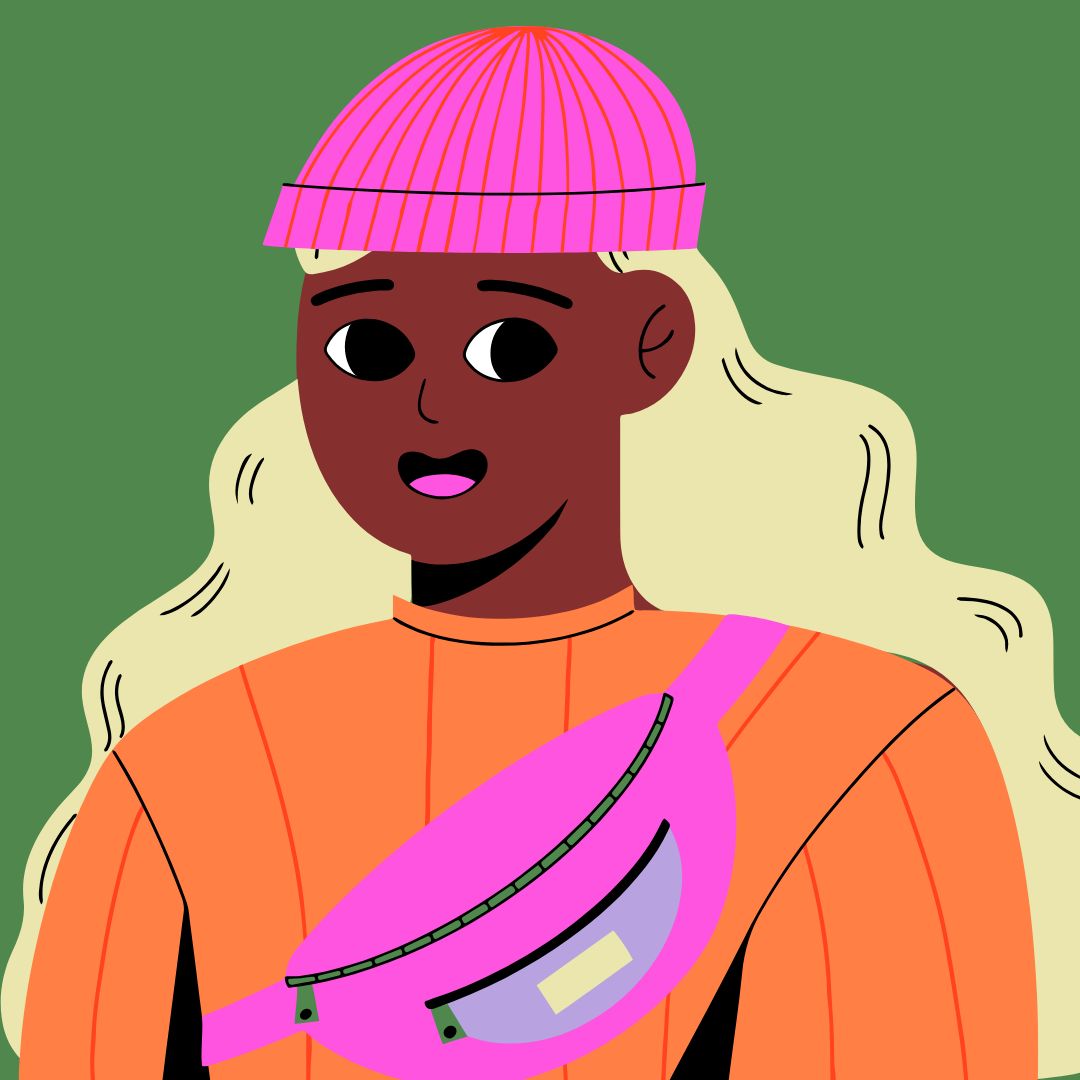
Sourav Pan
Transcript
Kingdom Monera was first proposed by Ernst Haeckel in 1866 as part of his three-kingdom classification system.
Initially, Haeckel considered Monera a subkingdom within his kingdom Protista, which included all single-celled organisms.
Over a century later, in 1969, R.H. Whittaker elevated Monera to kingdom status in his five-kingdom classification system.
This significant change recognized the fundamental biological differences between prokaryotes and eukaryotes.
Prokaryotes, which include all Monerans, lack a true nucleus. Their genetic material floats freely within the cell.
In contrast, eukaryotes have their DNA contained within a membrane-bound nucleus, a fundamental structural difference that justified separating these organisms into different kingdoms.
In the 1990s, Carl Woese made a groundbreaking discovery that transformed our classification of life.
He analyzed the genetic sequences of ribosomal RNA, a component found in all living cells.
Woese’s research challenged the traditional five-kingdom classification system that had been used for decades.
Based on his findings, Woese proposed a new three-domain system that replaced the traditional five kingdoms.
Under this new system, Kingdom Monera was divided into two separate domains: Bacteria and Archaea.
This reclassification was based on significant genetic differences between these two groups of prokaryotes.
Bacteria and Archaea show fundamental differences in their cell membrane composition, with Archaea having distinctive ether-linked lipids.
They also differ in their RNA polymerase structure, with Archaea having a more complex structure similar to eukaryotes.
Additionally, unique genetic markers distinguish Archaea from Bacteria, despite their similar cellular structures.
This reclassification was revolutionary because it recognized that despite their structural similarities as prokaryotes, Bacteria and Archaea represent distinct evolutionary lineages.
Today, Woese’s three-domain system has become the standard classification framework in modern biology, replacing the traditional five-kingdom system.
Monerans are prokaryotes, which means they have a simple cellular structure that differs significantly from eukaryotic cells.
Unlike eukaryotic cells, prokaryotes lack a membrane-bound nucleus.
Instead, their DNA floats freely in the cytoplasm in a region called the nucleoid.
Prokaryotic cells don’t have membrane-bound organelles such as mitochondria or chloroplasts.
This simple cellular organization was the first to evolve on Earth, making monerans the most ancient form of life.
Appearing approximately three point five billion years ago, these simple cells were able to reproduce through binary fission, a form of asexual reproduction.
This simple structure allows prokaryotes to be incredibly adaptable and successful, thriving in virtually every environment on Earth.
Most bacteria in Kingdom Monera have a distinctive cell wall structure.
The cell wall is a rigid structure surrounding the bacterial cell.
Let’s examine the composition of this cell wall in more detail.
The cell wall of most bacteria is composed of peptidoglycan, a polymer consisting of sugars and amino acids.
Peptidoglycan has a net-like structure with sugar chains connected by amino acid bridges.
This mesh-like structure provides rigidity and protection to the bacterial cell while maintaining its shape.
The cell wall composition is a key characteristic used in bacterial identification and classification through techniques like Gram staining.
The Gram stain technique differentiates bacteria based on their cell wall properties.
Gram-positive bacteria have a thick peptidoglycan layer, which retains the crystal violet stain, appearing purple under the microscope.
Gram-negative bacteria have a thinner peptidoglycan layer and an additional outer membrane. They appear pink under the microscope as they don’t retain the primary stain.
The cell wall serves several important functions for bacterial cells.
The rigid peptidoglycan structure provides critical protection and helps maintain the cell shape.
The cell wall provides protection against external pressures and maintains cell shape and integrity.
It prevents the cell from bursting in hypotonic environments and serves as an attachment site for other cell components.
Beyond its structural role, cell wall composition is crucial for bacterial classification, determining antibiotic sensitivity, and influencing pathogenicity.
Monerans reproduce primarily through asexual methods, with binary fission being the most common.
Binary fission is the primary method of reproduction in bacteria.
In binary fission, a single bacterial cell divides into two identical daughter cells.
The binary fission process occurs in three main steps.
First, the bacterial DNA replicates, creating two identical copies of the genetic material.
Next, the cell elongates, stretching to almost twice its original length.
Finally, the cell divides in the middle, forming two identical daughter cells, each with its own copy of DNA.
Some bacteria also reproduce through a process called budding.
In budding, a small outgrowth or bud forms on the parent cell.
The bud grows in size while remaining attached to the parent cell.
DNA is transferred to the bud, and eventually it develops into a complete cell.
Finally, the bud detaches from the parent cell, becoming a new independent cell.
These simple reproductive methods allow for rapid population growth under favorable conditions.
Starting with a single bacterial cell, let’s observe how quickly the population can grow through binary fission.
Under optimal conditions, some bacteria can divide every twenty minutes, allowing for exponential growth of bacterial populations.
Monerans exhibit various forms of locomotion using specialized structures.
Flagella are whip-like structures that propel bacteria through liquid environments.
The flagellum rotates like a propeller, pushing the bacterium forward through its environment.
Some bacteria use pili, which are hair-like appendages, for a type of movement called twitching motility.
Twitching motility occurs when bacteria extend pili, attach to a surface, and then retract the pili to pull themselves forward.
Other bacteria glide along surfaces without visible locomotory structures.
This smooth movement allows bacteria to travel across moist surfaces using mechanisms that are still being studied.
Bacterial mobility allows for taxis, which is directed movement toward nutrients or away from harmful substances.
Chemotaxis is the movement of bacteria toward higher concentrations of attractants, such as food sources.
As bacteria detect chemical gradients, they adjust their movement, preferentially moving toward beneficial substances.
Bacteria exhibit various forms of taxis, including chemotaxis in response to chemicals, phototaxis in response to light, and aerotaxis in response to oxygen.
To summarize, bacteria utilize diverse locomotion mechanisms including flagella, pili, gliding motility, and directed movement through taxis.
Archaebacteria, now classified under the domain Archaea, are single-celled organisms that can survive in extreme environments where other life forms cannot.
Archaebacteria are classified into different types of extremophiles based on the extreme environments they inhabit.
Thermophiles thrive in extremely hot environments like hydrothermal vents, with optimal growth temperatures between 80 and 110 degrees Celsius.
Halophiles live in highly saline environments such as salt lakes and salt flats, where salt concentrations can be up to ten times higher than seawater.
Acidophiles survive in extremely acidic conditions with pH levels between 0 and 3, such as acidic hot springs and mine drainage.
Methanogens produce methane as a metabolic byproduct and live in oxygen-free environments like deep sea sediments and the digestive tracts of animals.
Archaebacteria have distinct cell structures that set them apart from bacteria.
Archaebacteria possess unique cell membrane lipids with ether bonds instead of ester bonds found in bacteria. They also lack the peptidoglycan cell wall that is characteristic of bacteria.
The environments where archaebacteria thrive today are remarkably similar to the conditions on early Earth four billion years ago.
This similarity suggests that archaebacteria may resemble some of Earth’s earliest life forms, providing scientists with insights into how life evolved on our planet and potentially how life might exist on other worlds.
Archaebacteria represent one of the three domains of life, separate from both bacteria and eukaryotes. Genetic studies show that archaebacteria are more closely related to eukaryotes than to bacteria.
Their study provides crucial insights into the nature of the Last Universal Common Ancestor and the early evolution of life on Earth, helping scientists piece together how all modern life forms came to be.
Eubacteria, or true bacteria, are the most common and diverse group of monerans.
Eubacteria come in various shapes and forms, including spherical cocci, rod-shaped bacilli, and spiral-shaped spirilla.
Eubacteria have cell walls made of peptidoglycan, a complex structure of sugars and amino acids that gives them structural integrity. This feature is unique to true bacteria.
Eubacteria are found in virtually every habitat on Earth. They thrive in soil where they contribute to nutrient cycling, in water bodies as part of aquatic ecosystems, and within the human body as part of our microbiome.
Eubacteria include both beneficial species that are essential for ecosystems and human health, as well as pathogenic species that cause diseases. Beneficial bacteria assist in digestion, nitrogen fixation, and food production. Pathogenic bacteria cause illnesses such as strep throat, food poisoning, and tuberculosis.
Eubacteria reproduce through binary fission, a simple form of asexual reproduction. First, the DNA replicates. Then, the cell elongates. Finally, the cell divides into two identical daughter cells.
Many Eubacteria are motile, using structures like flagella to move through their environment. The flagellum rotates like a propeller, pushing the bacterium forward.
To summarize, Eubacteria are the most common and diverse group of prokaryotes. Their cell walls contain peptidoglycan, they exist in virtually every habitat on Earth, include both beneficial and harmful species, and reproduce through binary fission.
Cyanobacteria are a group of photosynthetic prokaryotes, formerly classified as blue-green algae.
They have a simple cellular structure with characteristic internal thylakoid membranes where photosynthesis occurs.
What gives cyanobacteria their distinctive blue-green color is their unique combination of pigments: chlorophyll a for green, phycocyanin for blue, and phycoerythrin for red.
Cyanobacteria have played a crucial role in Earth’s history through their oxygen-producing photosynthesis.
Like plants, cyanobacteria perform photosynthesis, converting carbon dioxide and water into sugar and oxygen using sunlight energy.
About 2.4 billion years ago, cyanobacteria triggered the Great Oxygenation Event, transforming Earth’s atmosphere from oxygen-poor to oxygen-rich.
This fundamental change in atmospheric composition made complex aerobic life forms possible, paving the way for the evolution of animals, plants, and eventually humans.
Monerans exhibit remarkable metabolic diversity unmatched by any other group of organisms.
They can be classified into four main metabolic types based on how they obtain energy and carbon.
Photoautotrophs, like cyanobacteria, use sunlight as their energy source and carbon dioxide for building their cells.
Chemoautotrophs obtain energy by oxidizing inorganic compounds such as hydrogen sulfide or ammonia, while still using carbon dioxide as their carbon source.
Photoheterotrophs use light for energy, but unlike photoautotrophs, they require organic carbon compounds.
Finally, chemoheterotrophs, the most common type, use organic compounds as both their energy and carbon sources.
This metabolic versatility allows monerans to inhabit virtually every ecological niche on Earth.
From sun-drenched ocean surfaces where photoautotrophs thrive, to deep-sea hydrothermal vents where chemoautotrophs convert sulfur compounds to energy.
From nutrient-rich soils where photoheterotrophs capture both light and organic matter, to animal intestines where chemoheterotrophs break down complex molecules.
This metabolic diversity is fundamental to global ecosystem function.
Monerans drive essential processes like decomposition, nitrogen fixation, and carbon cycling. Their metabolic capabilities allow them to thrive in environments from deep-sea trenches to hot springs, and even within other organisms.
Monerans play a crucial role as primary decomposers in ecosystems.
Among these, bacteria like Bacillus and Pseudomonas are particularly important for decomposition processes.
The decomposition process begins when bacteria secrete enzymes that break down complex organic matter.
These enzymes digest complex organic molecules externally, breaking them down into simpler compounds.
The bacteria then absorb these simpler compounds for their own metabolism and growth.
Through decomposition, monerans recycle nutrients back into the ecosystem.
These recycled nutrients become available for plants and other producers to use.
When plants and other organisms die, they become organic matter that decomposers break down, completing the nutrient cycle.
The decomposition activities of monerans are essential for ecosystem functioning in several ways.
Without bacterial decomposers, dead organic matter would accumulate, and nutrients would remain locked in complex forms, unavailable to other organisms.
The human digestive system hosts trillions of beneficial bacteria that play crucial roles in our health.
The human gut microbiome contains over one hundred trillion bacterial cells, outnumbering our own body cells. These bacteria predominantly reside in the small and large intestines.
These beneficial bacteria help break down complex carbohydrates that human enzymes cannot digest. Bacterial enzymes split large polysaccharides into simple sugars that can be absorbed by our intestinal cells.
Gut bacteria produce essential vitamins that our bodies cannot synthesize. These include vitamin K, which is crucial for blood clotting and bone metabolism, and several B vitamins needed for energy production and nerve function.
Beneficial gut bacteria form a protective barrier against harmful pathogens. They occupy ecological niches, preventing colonization by disease-causing bacteria. They also produce antimicrobial compounds and stimulate our immune system to fight infections.
The gut microbiome is increasingly recognized as crucial for overall health. About seventy percent of our immune cells reside in the gut, where bacteria help train immune responses. Through the gut-brain axis, these microbes influence mood and cognitive function. They also play key roles in metabolism, energy extraction, and blood sugar regulation.
Understanding the critical roles of beneficial bacteria in our digestive system highlights the importance of maintaining a healthy gut microbiome through diet and lifestyle choices.
Disease-causing monerans can have devastating effects on human health.
Pathogenic bacteria have shaped human history through devastating epidemics. Three notable examples include Mycobacterium tuberculosis, Vibrio cholerae, and Yersinia pestis.
These diseases have had profound impacts throughout history. The Black Death killed an estimated one-third of Europe’s population in the 14th century. Cholera caused seven major pandemics from the 19th to early 20th centuries. Tuberculosis was known as ‘the white plague’ and was a leading cause of death in the industrial era.
Bacteria cause disease through several mechanisms. Some produce toxins that damage host cells. Others directly invade tissues, multiplying and spreading throughout the body. Many trigger harmful immune responses that cause inflammation and tissue damage.
Understanding these pathogens is crucial for disease prevention and treatment. Vaccines train the immune system to recognize and fight specific bacteria. Antibiotics disrupt bacterial processes like cell wall synthesis. Simple measures like proper sanitation can prevent transmission.
Monerans, especially bacteria, have become essential tools in modern biotechnology.
Bacteria like Escherichia coli serve as cellular factories for producing valuable pharmaceuticals.
Through genetic engineering, scientists can program these bacteria to produce human insulin, growth hormones, and various vaccines.
The process involves inserting human genes into bacterial DNA, effectively turning these simple organisms into specialized molecular factories.
Bacterial enzymes are powerful biological catalysts with numerous industrial applications.
In the detergent industry, bacterial proteases and lipases help remove protein and fat stains from clothing.
Food processing uses bacterial amylases to convert starch to sugar in brewing and baking.
The textile industry employs bacterial cellulases to soften fabrics and reduce pilling.
The CRISPR-Cas9 gene editing system has revolutionized biotechnology and genetic engineering.
This powerful tool was derived from bacterial immune systems that protect against viral invaders.
CRISPR technology has numerous applications, including treating genetic diseases, improving crops, and developing new antimicrobials.
To summarize, monerans have transformed biotechnology through their use as cellular factories, sources of industrial enzymes, and the revolutionary CRISPR-Cas9 system.
Bacteria have become invaluable tools in scientific research.
Model organisms in microbiology provide scientists with essential insights into fundamental biological processes.
Two key bacterial model organisms are Escherichia coli and Bacillus subtilis.
These bacteria offer several advantages for research: they have simple prokaryotic structures, reproduce rapidly in just twenty to thirty minutes, have well-characterized genetics, and can be easily manipulated in the lab.
One of the most valuable features of bacterial models is their rapid cell division cycle.
A bacterial cell elongates, replicates its DNA, and then divides into two identical daughter cells.
Bacterial models have been instrumental in studying fundamental processes including DNA replication, gene regulation, and protein synthesis.
Research on bacterial DNA replication has been crucial for understanding this fundamental process.
Bacterial models revealed how DNA unwinds and new complementary strands are synthesized, creating two identical copies of the genetic material.
Discoveries in bacterial models have revealed fundamental principles that apply across all forms of life.
These include the central dogma of molecular biology, CRISPR gene editing technology, understanding of antibiotic resistance, and advances in genomics and biotechnology.
Bacterial model organisms continue to drive scientific breakthroughs and expand our fundamental understanding of life.
Monerans are key players in maintaining ecological balance through their essential roles in biogeochemical cycles.
These microscopic organisms participate in several major biogeochemical cycles that regulate our planet’s chemistry.
In the carbon cycle, methanogenic archaea play a crucial role by producing methane in anaerobic environments such as wetlands, landfills, and animal digestive tracts.
In the sulfur cycle, specialized bacteria convert sulfur compounds between different forms. Sulfur-oxidizing bacteria convert sulfide to sulfate, while sulfur-reducing bacteria do the reverse.
In the phosphorus cycle, phosphate-solubilizing bacteria play a critical role by breaking down insoluble phosphate compounds and making phosphorus available to plants.
These biogeochemical processes facilitated by monerans are essential for ecosystem functioning, nutrient recycling, and global environmental sustainability.
Escherichia coli, commonly known as E. coli, is one of the most well-studied bacteria. It naturally inhabits the gut of humans and animals.
E. coli has become an invaluable model organism in molecular biology research, contributing to our understanding of bacterial genetics, metabolism, and protein synthesis.
Staphylococcus aureus is a spherical bacterium that forms grape-like clusters. It commonly causes skin infections, abscesses, and food poisoning.
Some strains have developed resistance to antibiotics, known as MRSA or Methicillin-Resistant Staphylococcus Aureus, which presents significant challenges in medical settings.
Bacillus anthracis is the rod-shaped bacterium that causes anthrax, a serious infectious disease. It forms endospores that can remain viable in soil for decades.
These endospores are highly resistant to environmental stresses and disinfectants, making Bacillus anthracis a potential bioterrorism agent.
Cyanobacteria, also known as blue-green algae, are photosynthetic bacteria with significant ecological importance.
Nostoc forms distinct gelatinous colonies in soil and freshwater environments. These colonies can survive extreme drying and play important roles in soil fertility.
Anabaena is a filamentous cyanobacterium known for its nitrogen-fixing abilities. It contains specialized cells called heterocysts that convert atmospheric nitrogen into forms plants can use.
This nitrogen fixation capability makes Anabaena important in aquatic ecosystems and in symbiotic relationships with certain plants like water ferns.
Spirulina is a spiral-shaped cyanobacterium that has gained popularity as a nutritional supplement. It’s exceptionally rich in protein, vitamins, and antioxidants.
Spirulina has been used as a food source for centuries by various cultures and is now commercially cultivated worldwide. It’s also being researched as a potential source for sustainable biofuels.
Archaea represent the third domain of life, previously classified within Monera.
Despite their visual similarities to bacteria, archaea are now recognized as a distinct domain of life, alongside Bacteria and Eukarya.
Archaea include three major groups that thrive in extreme environments.
Methanogens produce methane gas as a metabolic byproduct and are found in anoxic environments like marshes and the digestive tracts of animals.
Halophiles thrive in extremely salty environments such as the Dead Sea, salt lakes, and salt flats, where most other organisms cannot survive.
Thermoacidophiles inhabit some of the most extreme environments on Earth, such as volcanic hot springs, where temperatures exceed 80 degrees Celsius and pH levels can be highly acidic.
Despite superficial similarities to bacteria, archaea possess unique genetic and biochemical characteristics that place them closer to eukaryotes in some respects.
Their membrane lipids are ether-linked rather than ester-linked, they possess histone proteins similar to eukaryotes, and they share more translational and transcriptional machinery with eukaryotes than with bacteria.
The unique position of Archaea in the tree of life has profound evolutionary significance. They may represent a crucial link between bacteria and the more complex eukaryotes.
Although Kingdom Monera is now taxonomically outdated, its study remains fundamental to various scientific fields.
The study of prokaryotes—both bacteria and archaea—is essential in biology, ecology, medicine, and biotechnology.
Understanding prokaryotes provides crucial insights into the origin of life and evolutionary processes.
These microorganisms represent some of the earliest forms of life, providing valuable clues about how life evolved over billions of years.
Prokaryotes are vital for the functioning of ecosystems, driving key processes like nutrient cycling, decomposition, and even photosynthesis in cyanobacteria.
As we face challenges like antibiotic resistance and climate change, our knowledge of these ancient and adaptable organisms becomes increasingly valuable.
In conclusion, though Kingdom Monera is now taxonomically outdated, the study of prokaryotes remains foundational to science and essential for addressing global challenges.
Study Materials
Kingdom Monera - Classification, Characteristics, Importance, Examples
Top 14 Difference Between Monera and Protista
Top 10 Difference Between Monera, Protista, Fungi, Animalia, Plantae
Helpful: 0%
Related Videos
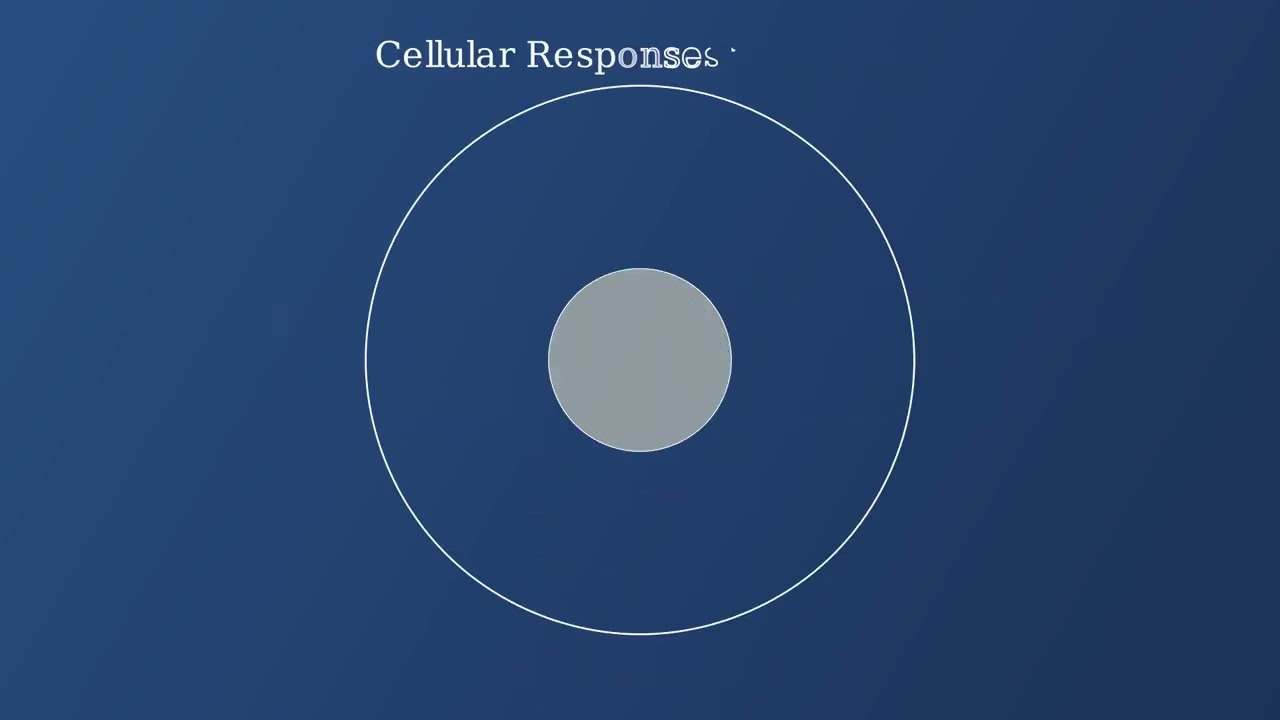
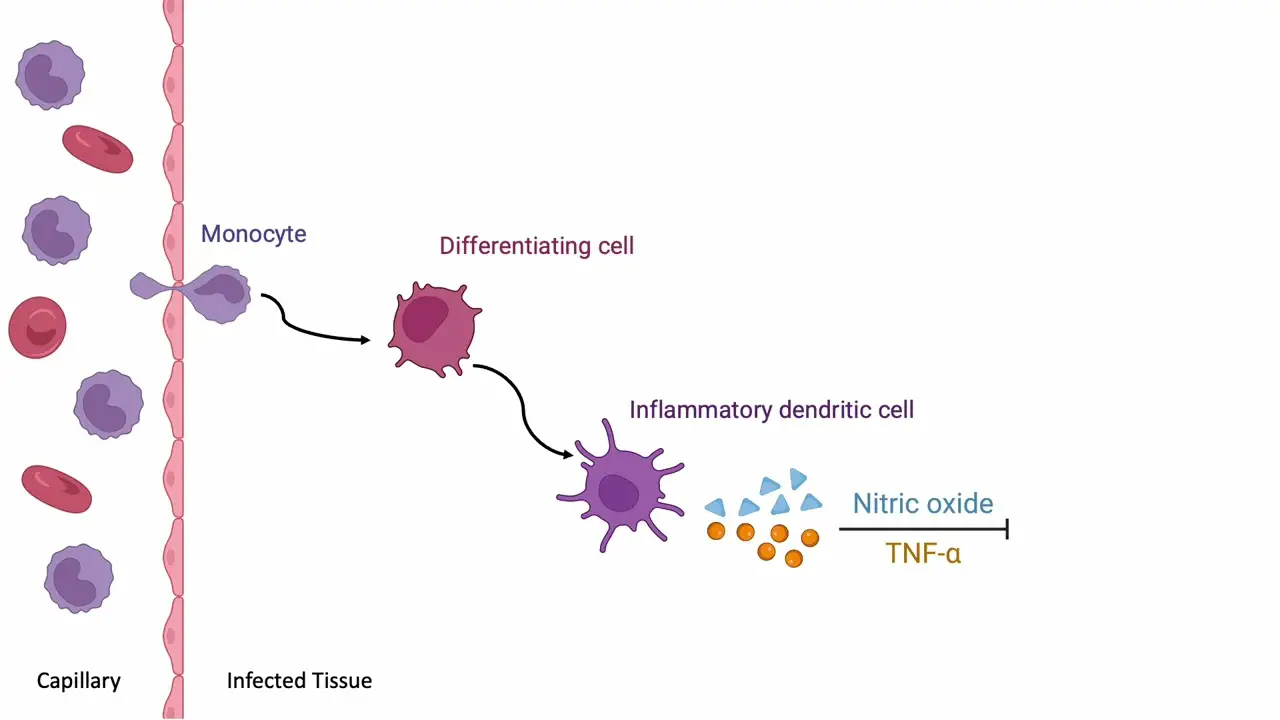
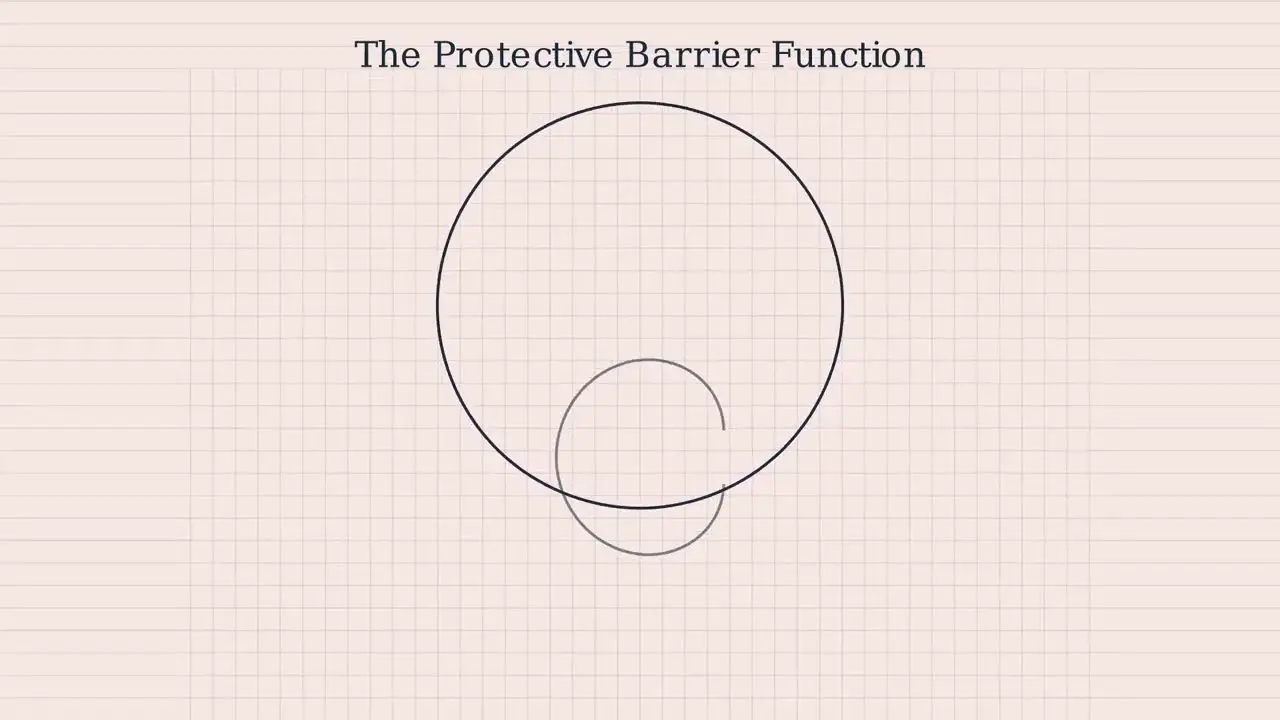
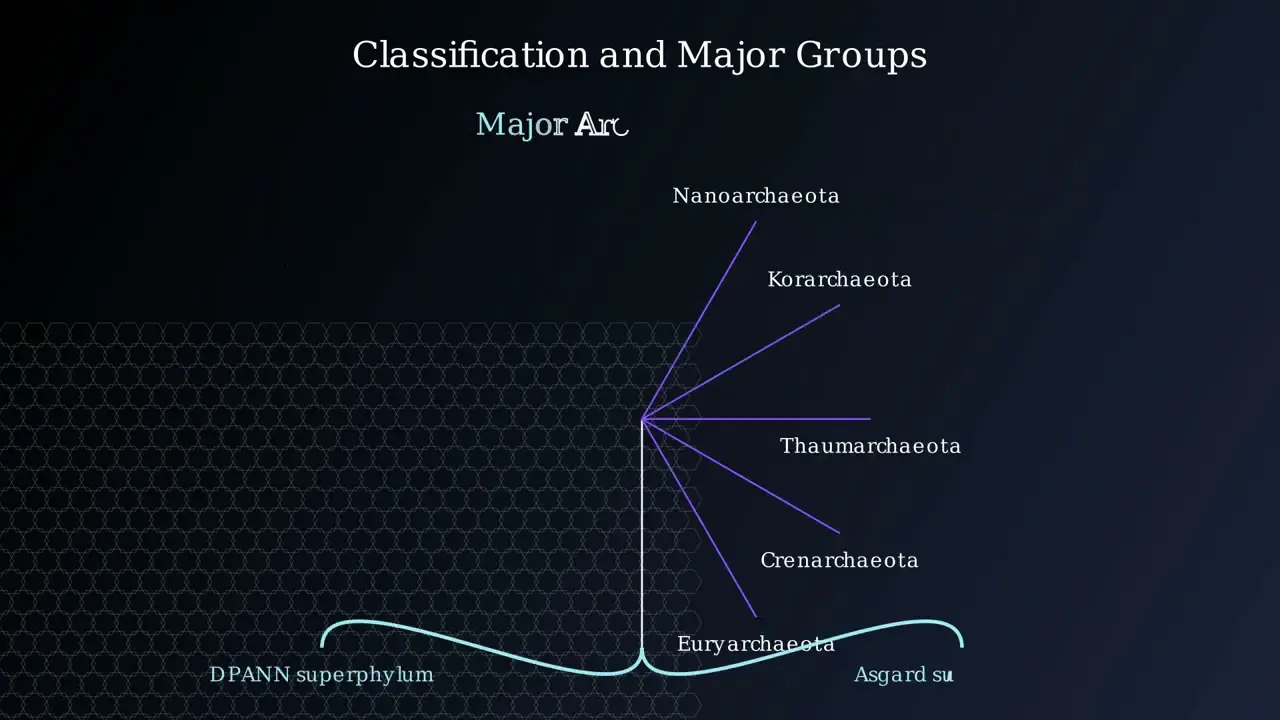
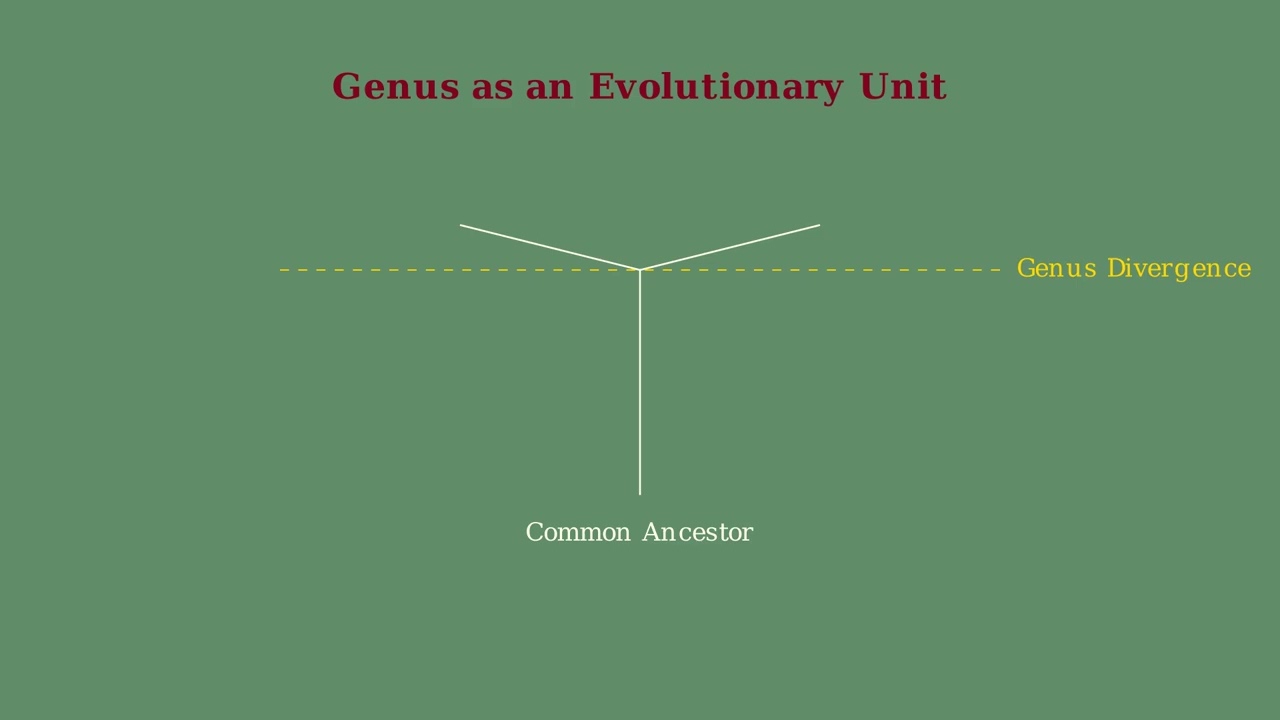
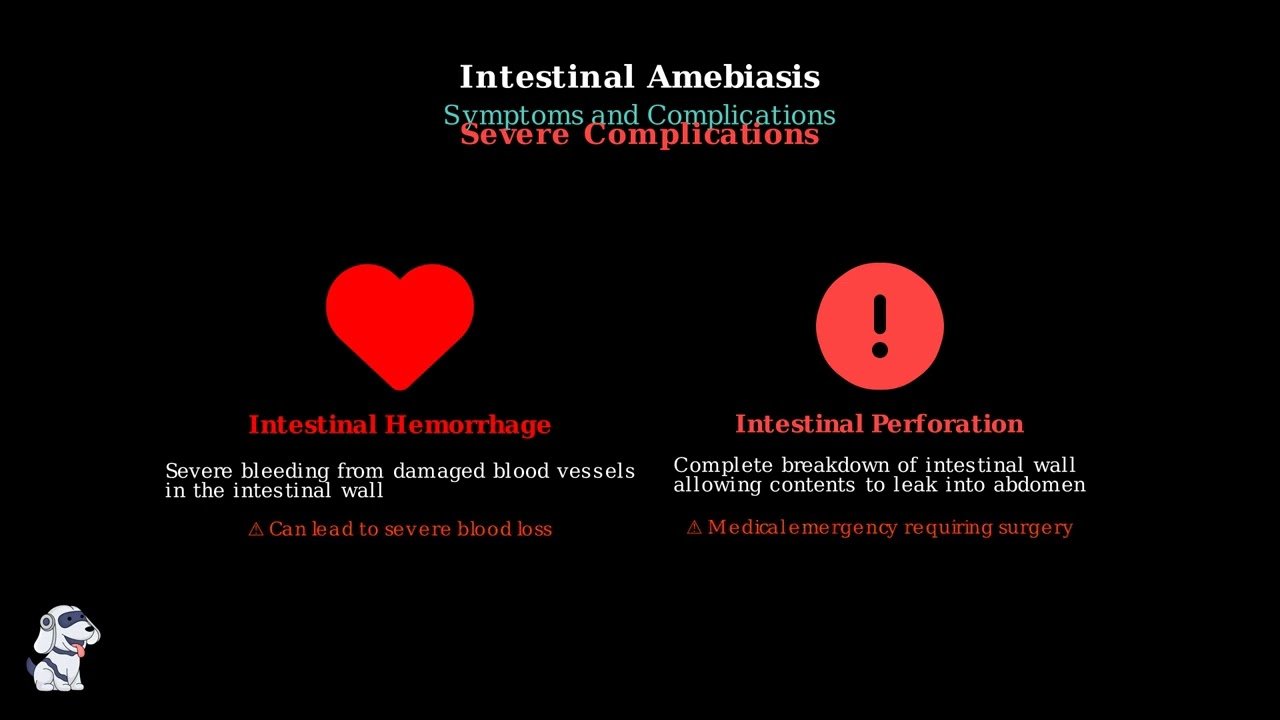
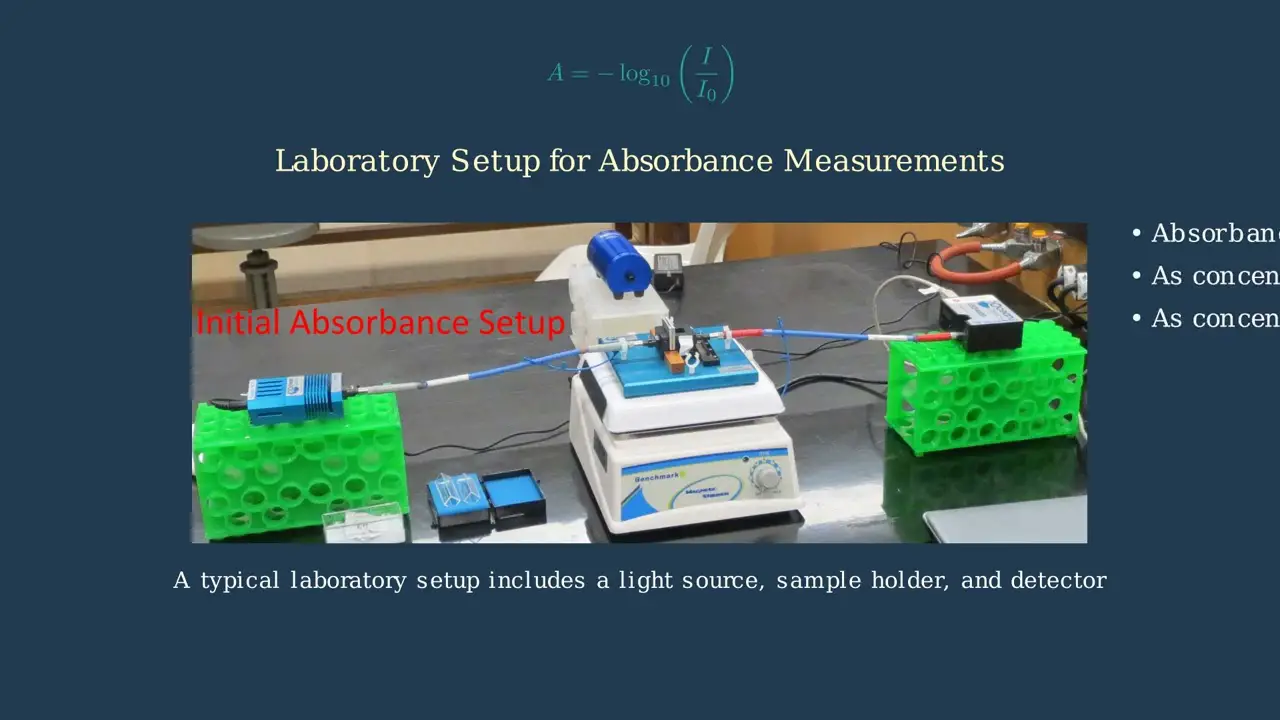
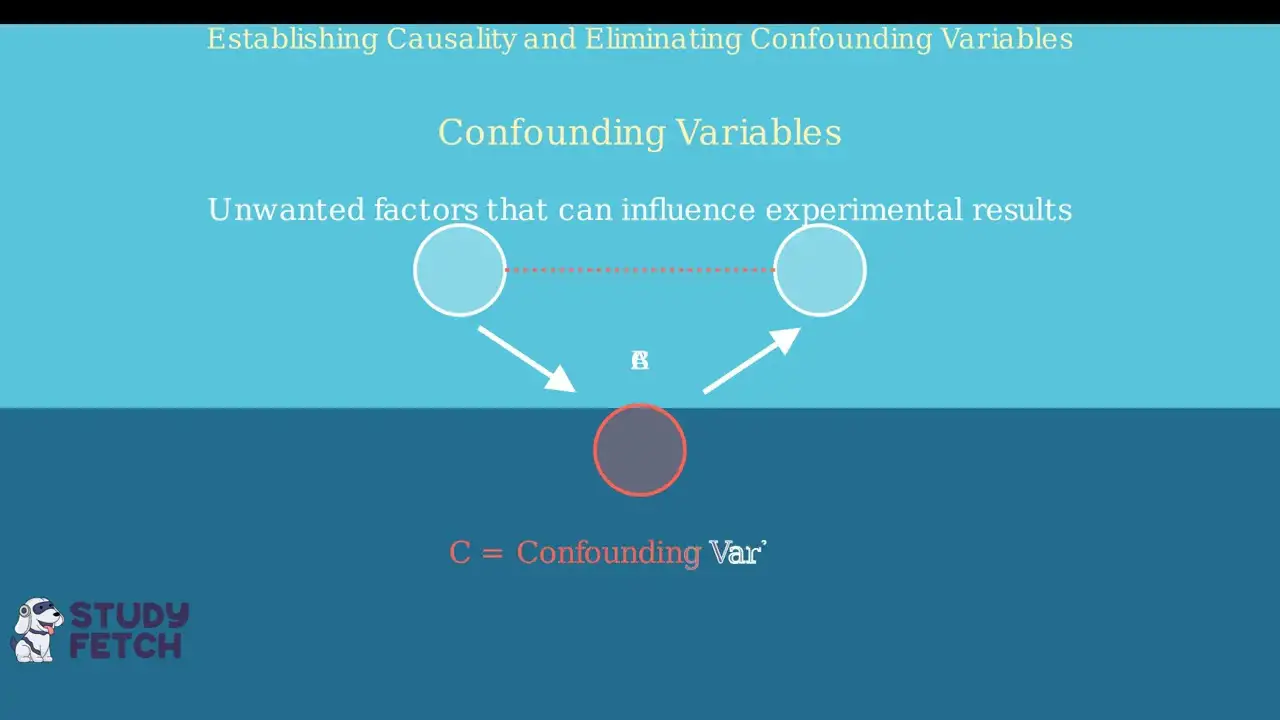
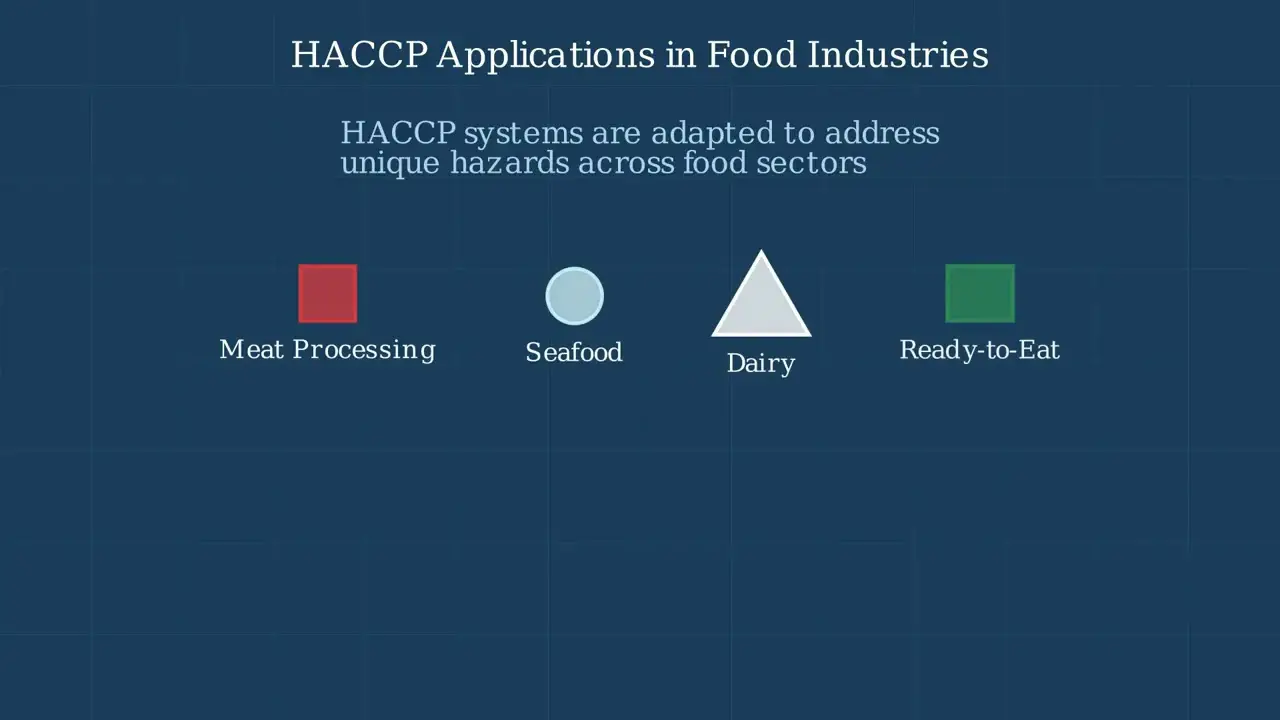
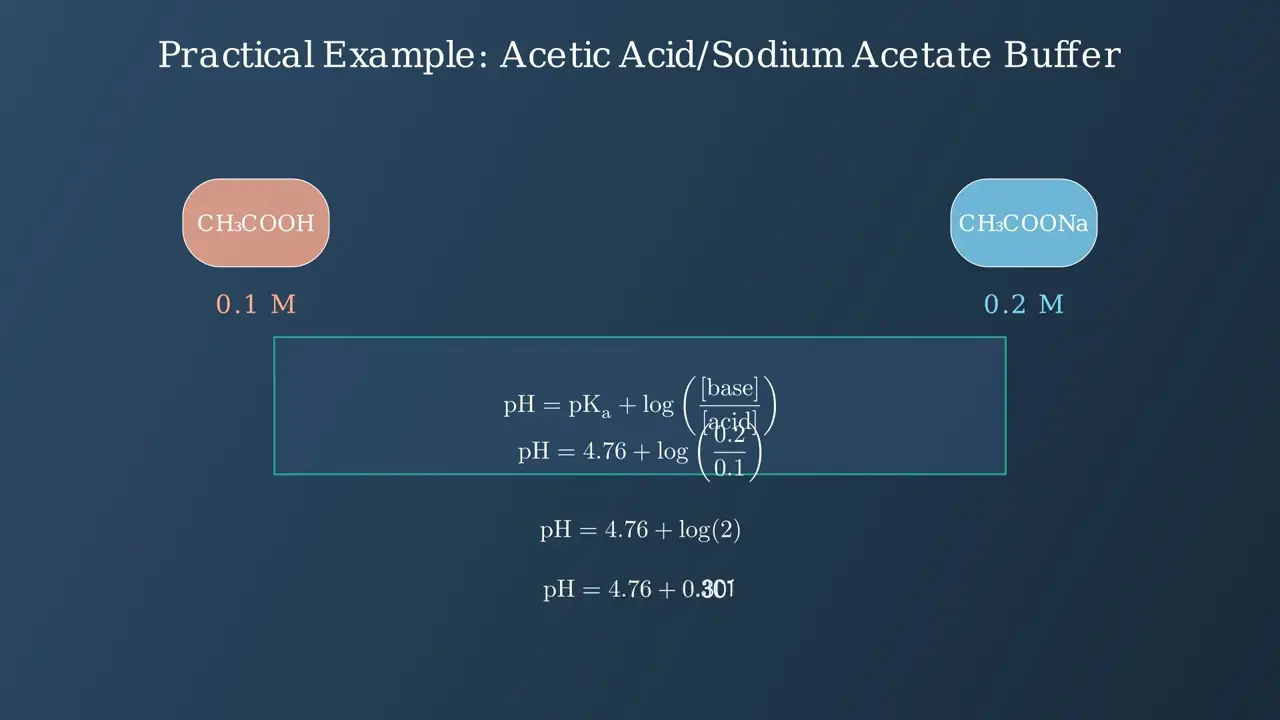