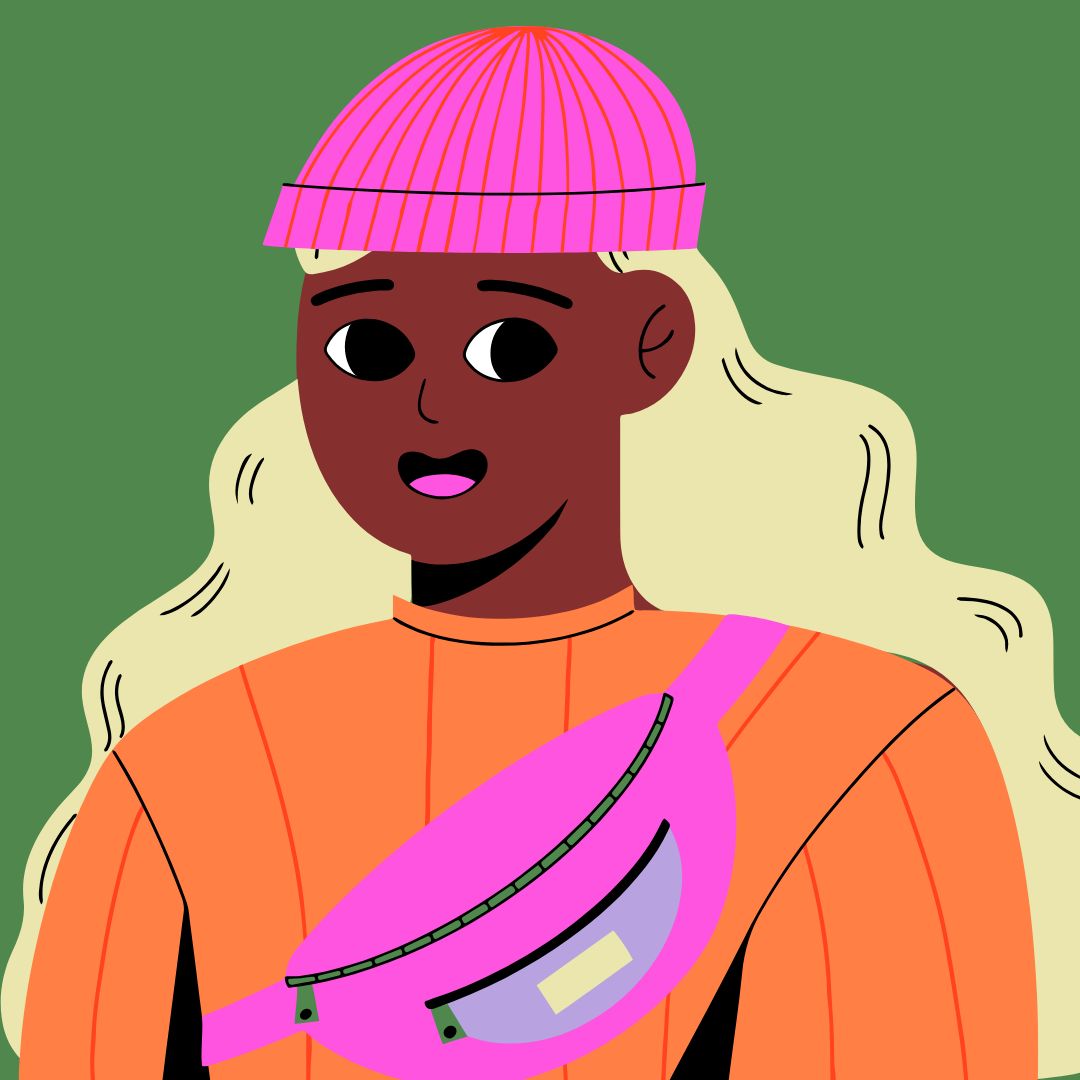
Sourav Pan
Transcript
The foundation of the cell membrane is the phospholipid bilayer.
Each phospholipid molecule has a hydrophilic head and two hydrophobic fatty acid tails.
The hydrophilic head is water-loving, while the hydrophobic tails are water-repelling.
In water, these molecules naturally arrange themselves into a double layer called a bilayer.
The hydrophilic heads face outward toward the watery environments both inside and outside the cell.
The hydrophobic tails face inward toward each other, away from water.
This arrangement creates a stable barrier that separates the cell’s interior from its exterior environment.
The phospholipid bilayer is flexible and semi-fluid, allowing for some movement while maintaining its barrier properties.
Proteins are crucial components of the cell membrane, making up about fifty percent of its mass.
There are two main categories of membrane proteins: integral proteins, which extend through the entire bilayer, and peripheral proteins, which associate with the membrane surface.
Integral proteins often have hydrophobic regions that anchor them within the membrane’s interior.
These integral proteins also have hydrophilic regions that extend into the aqueous environments on either side of the membrane.
Peripheral proteins attach temporarily to either the membrane surface or to integral proteins through various interactions.
Together, these different types of membrane proteins perform a wide range of vital cellular functions.
Integral membrane proteins are permanently embedded within the phospholipid bilayer of cell membranes.
These proteins contain hydrophobic amino acid regions that interact with the fatty acid tails of phospholipids in the membrane interior.
They also have hydrophilic domains that extend into the aqueous environments on either side of the membrane.
Many integral proteins are transmembrane proteins, spanning the entire phospholipid bilayer.
Other integral proteins may be partially embedded, with portions extending into only one side of the membrane.
Some integral proteins function specifically as transport channels, creating passages through which molecules can cross the membrane.
Integral membrane proteins serve various essential functions in cells.
Transport proteins create channels or carriers that move substances like ions, sugars, and amino acids across the membrane.
Some integral proteins have enzymatic properties, catalyzing important biochemical reactions at the membrane surface.
Receptor proteins receive chemical signals from outside the cell and initiate appropriate responses within the cell.
Adhesion proteins help cells bind to neighboring cells or to the extracellular matrix.
Some integral proteins provide structural support, helping to maintain cell shape and stability.
The specific three-dimensional structure of integral membrane proteins is critical for their functions.
Their complex folding creates specific binding sites, allows for conformational changes during function, and determines how they interact with other molecules.
Section 7: Peripheral Membrane Proteins
To understand peripheral membrane proteins, let’s first look at the cell membrane structure.
We previously learned about integral membrane proteins, which penetrate through the entire membrane.
In contrast, peripheral membrane proteins do not penetrate the hydrophobic core of the bilayer.
Instead, they attach temporarily to the membrane surface through specific interactions.
They may interact with integral proteins embedded in the membrane.
Or they may bind to the polar heads of phospholipids on the membrane surface.
A key characteristic of peripheral proteins is that they can be easily removed without disrupting the membrane structure.
Peripheral membrane proteins serve several important functions.
They participate in cell signaling pathways, transmitting information into and out of the cell.
They can function as enzymes, catalyzing chemical reactions at the membrane surface.
They can also connect the membrane to the cytoskeleton, providing structural support.
Their reversible attachment allows for dynamic regulation of cellular processes.
In summary, peripheral membrane proteins are characterized by their temporary attachment to the membrane surface without penetrating the hydrophobic core, allowing for dynamic regulation of many cellular processes.
The Fluid Mosaic Model, proposed by Singer and Nicolson in 1972, represents our current understanding of membrane structure.
It describes the membrane as a fluid phospholipid bilayer in which protein molecules are embedded, creating a mosaic-like pattern.
The ‘fluid’ aspect refers to the lateral movement of membrane components within the bilayer.
The ‘mosaic’ describes the varied arrangement of proteins throughout the membrane, creating a mosaic-like pattern.
This model emphasizes the dynamic nature of the membrane, with components able to move, interact, and reorganize in response to cellular needs.
Membrane fluidity refers to the ability of membrane components to move laterally within the bilayer.
This property is crucial for numerous cellular functions including nutrient absorption, waste elimination, and cell growth.
Fluidity is influenced by several factors. First, temperature: higher temperatures increase membrane fluidity by providing energy for phospholipid movement.
Second, fatty acid composition affects fluidity. Unsaturated fatty acids have kinks in their tails that prevent tight packing, increasing membrane fluidity.
Third, cholesterol content plays a dual role in membrane fluidity. At high temperatures, it reduces fluidity by restricting phospholipid movement. At low temperatures, it prevents membranes from becoming too rigid.
Proper membrane fluidity ensures that the membrane can perform its functions efficiently while maintaining structural integrity. The cell must maintain a delicate balance between fluidity for function and stability for structure.
One of the most important properties of the cell membrane is selective permeability.
This is the ability to allow some substances to pass through while blocking others.
Small, nonpolar molecules like oxygen and carbon dioxide can diffuse directly through the phospholipid bilayer.
Water molecules can pass through specialized protein channels called aquaporins.
Larger or charged molecules require specific transport proteins to cross the membrane.
This selective barrier function allows the cell to maintain its internal environment distinct from its surroundings.
Cell membranes play a vital role in how cells identify and interact with each other.
On the cell surface, specialized molecules serve as identification markers.
Glycoproteins and glycolipids on the cell surface act as cellular ‘ID cards’.
Cell adhesion molecules, or CAMs, help cells bind to each other and to the extracellular matrix.
These recognition and adhesion functions are essential for tissue formation, immune response, and maintaining multicellular organization.
Through these mechanisms, cells can recognize their own kind and distinguish foreign entities, allowing complex tissues and organs to form and function properly.
The cell membrane serves as a physical barrier that protects the cell’s internal components from the external environment.
It prevents the leakage of vital cellular contents while blocking harmful substances from entering the cell.
When harmful particles attempt to enter the cell, the membrane acts as a selective barrier, preventing their passage.
Similarly, essential cellular contents are contained within the cell, maintaining the internal environment.
The integrity of the cell membrane is maintained through continuous repair mechanisms.
When damage occurs to the cell membrane, the cell has mechanisms to quickly repair the breach.
Repair vesicles inside the cell rapidly move to the damaged area and fuse with the membrane.
This fusion seals the breach and restores the membrane’s barrier function, protecting the cell from the external environment.
In multicellular organisms, specialized membrane structures like tight junctions provide additional barrier protection.
These junctions form seals between adjacent cells, preventing the unregulated passage of materials between them.
This barrier function is essential for maintaining the integrity of tissues and organs, allowing them to function as specialized units.
Let’s examine the historical models of cell membranes proposed by Davson, Danielli, and Robertson.
In 1935, Hugh Davson and James Danielli proposed a model for the cell membrane structure.
Their model featured a phospholipid bilayer sandwiched between two continuous layers of proteins.
In the 1950s, J. David Robertson refined this concept into what he called the ‘unit membrane’ model.
Robertson proposed that all cellular membranes, regardless of their location or function, shared the same basic structure.
While these early models correctly identified the phospholipid bilayer nature of the membrane, they had a fundamental flaw.
They incorrectly positioned proteins as continuous sheets covering the membrane surfaces, rather than as discrete molecules embedded within and across the bilayer.
Despite their limitations, the Davson-Danielli and Robertson models were crucial steps in our understanding of cell membrane structure. Their correct identification of the phospholipid bilayer laid the groundwork for the later Fluid Mosaic Model.
In 1972, Singer and Nicolson revolutionized our understanding of cell membranes.
They proposed the Fluid Mosaic Model, which fundamentally changed how we view cellular membranes.
This model correctly described proteins as discrete molecules partially or wholly embedded within the fluid phospholipid bilayer.
Unlike previous models that suggested proteins formed continuous sheets on the membrane surface, the fluid mosaic model recognized the diverse ways proteins integrate with the lipid bilayer.
It emphasized the dynamic nature of the membrane, with components able to move laterally within their respective leaflets.
The Singer-Nicolson model recognized the diversity of protein arrangements within the membrane, accommodating various types of membrane proteins.
This model has become the foundation of our modern understanding of cell membranes.
Let’s explore the diverse protein arrangements described by this model.
Despite being proposed nearly fifty years ago, the fluid mosaic model remains our accepted view of membrane structure today.
While the model has been refined over decades with new discoveries, its fundamental principles have not changed.
Modern refinements have added concepts like lipid rafts, protein clustering, and a more detailed understanding of membrane domains.
The Singer-Nicolson Fluid Mosaic Model represents one of the most important paradigm shifts in our understanding of cell biology and continues to guide research today.
An important aspect of cell membranes is their asymmetry – the inner and outer leaflets of the bilayer differ in composition.
The outer leaflet typically contains more phosphatidylcholine and sphingolipids.
While the inner leaflet has more phosphatidylserine and phosphatidylethanolamine.
Glycolipids and most glycoproteins are found exclusively on the outer surface of the membrane.
This asymmetry is actively maintained by specialized enzymes called flippases, floppases, and scramblases.
Flippases actively move specific phospholipids from the outer leaflet to the inner leaflet.
Scramblases, when activated, can disrupt this asymmetry, which is an important signal for cellular processes like apoptosis – programmed cell death.
This membrane asymmetry is crucial for proper cell function, including cell recognition, membrane stability, signaling processes, and apoptosis initiation.
In conclusion, the cell membrane is far more than a simple barrier.
It’s a dynamic, complex structure that is central to cellular life.
Its phospholipid bilayer foundation, embedded with diverse proteins and modified with carbohydrates, creates a multifunctional interface between the cell and its environment.
From controlling molecular traffic to receiving signals, from identifying cellular neighbors to protecting internal contents, the membrane performs countless essential functions.
Our understanding of this remarkable structure continues to evolve, revealing ever more sophisticated aspects of its organization and function.
Study Materials
No study materials available for this video.
Helpful: 0%
Related Videos
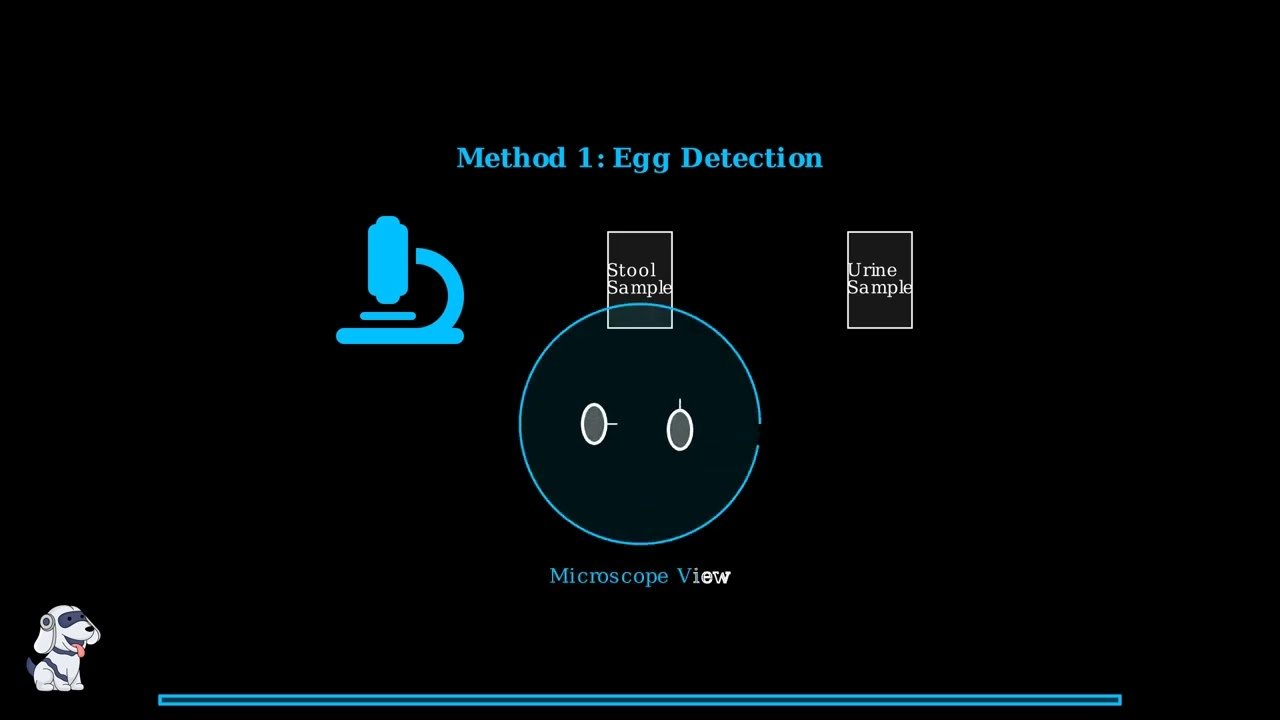
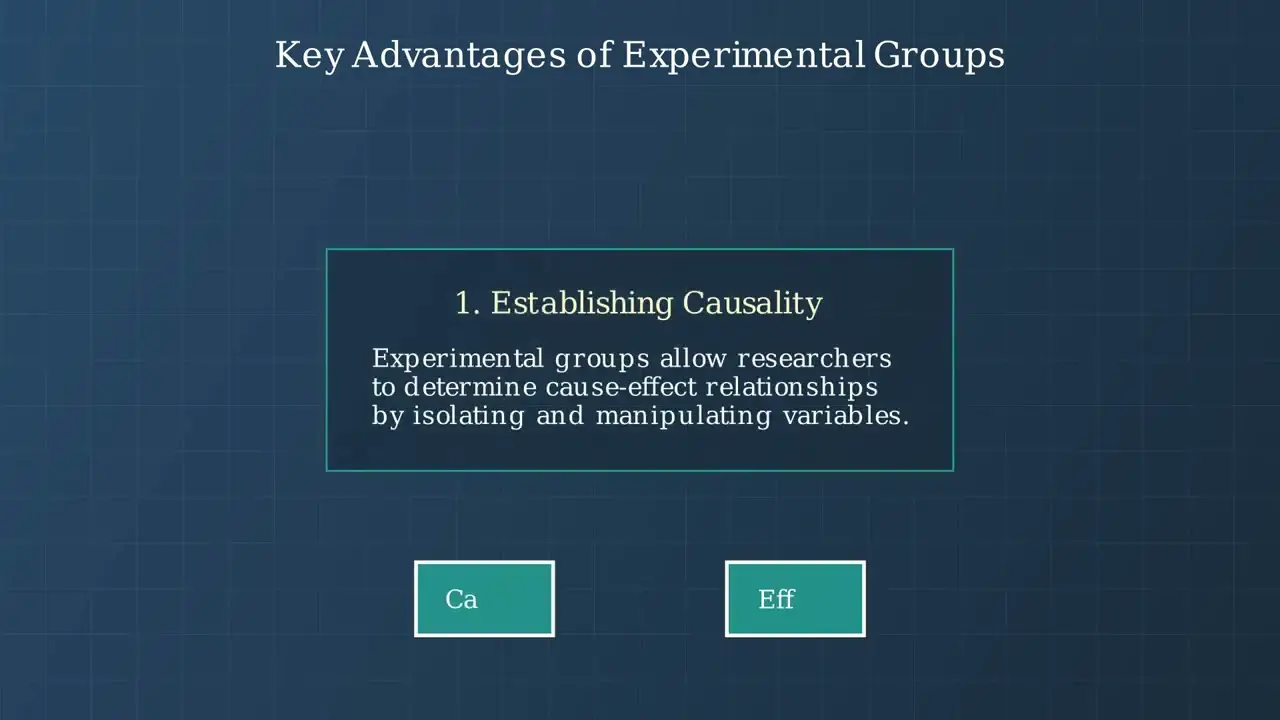
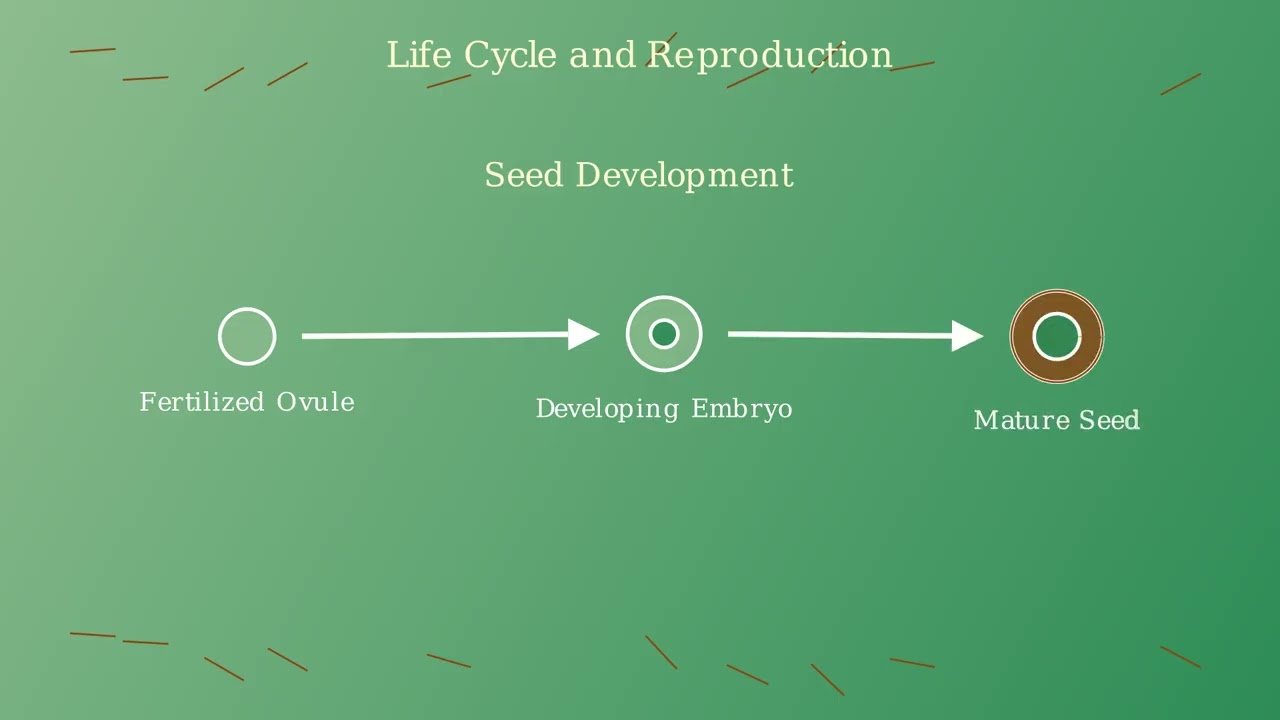
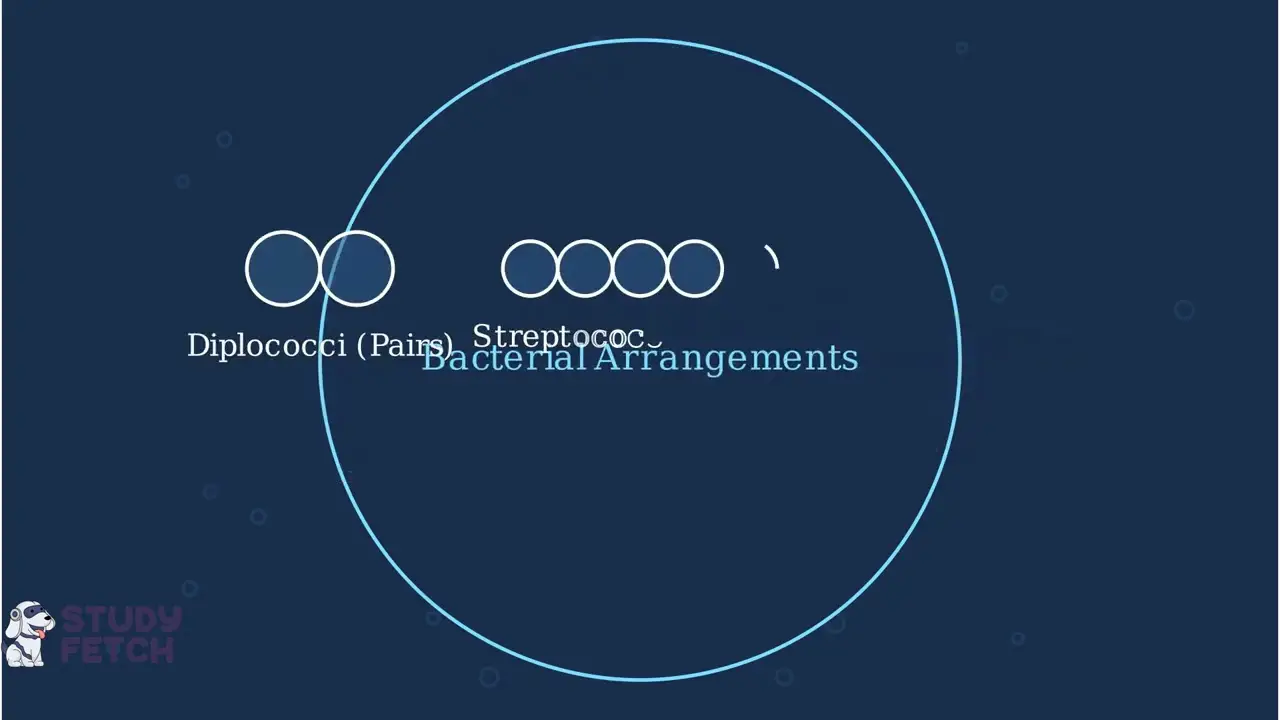
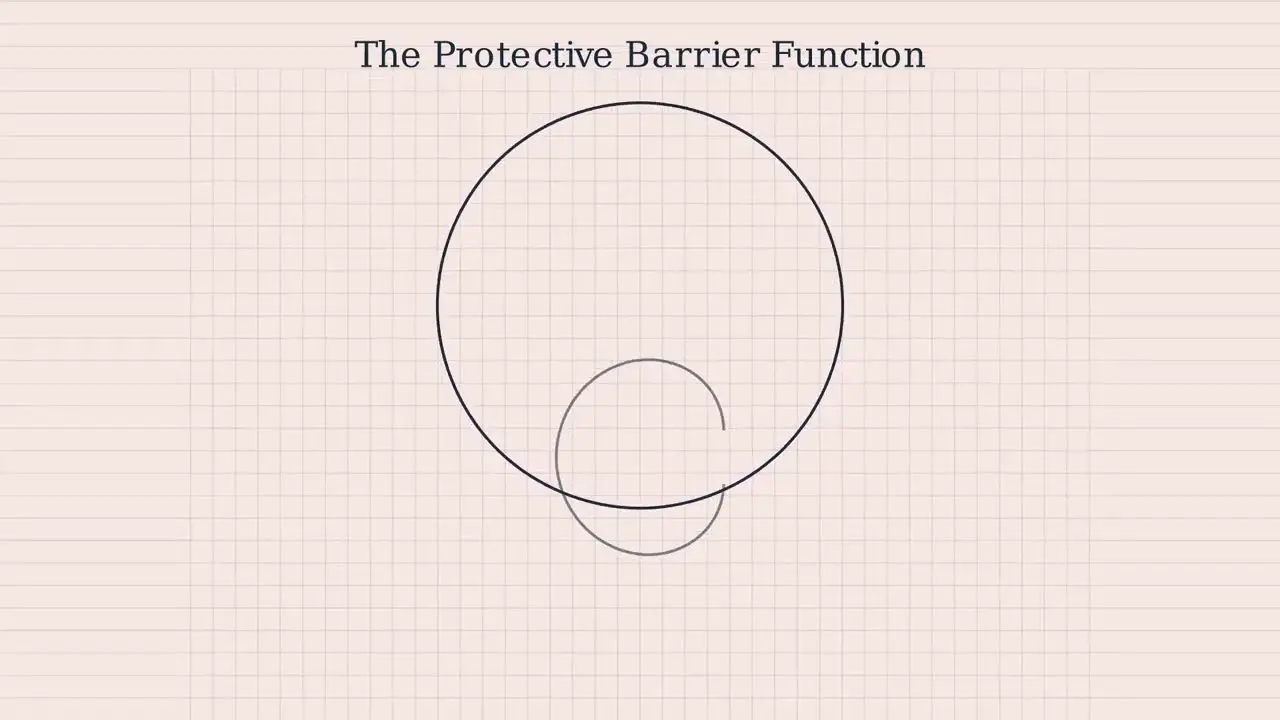
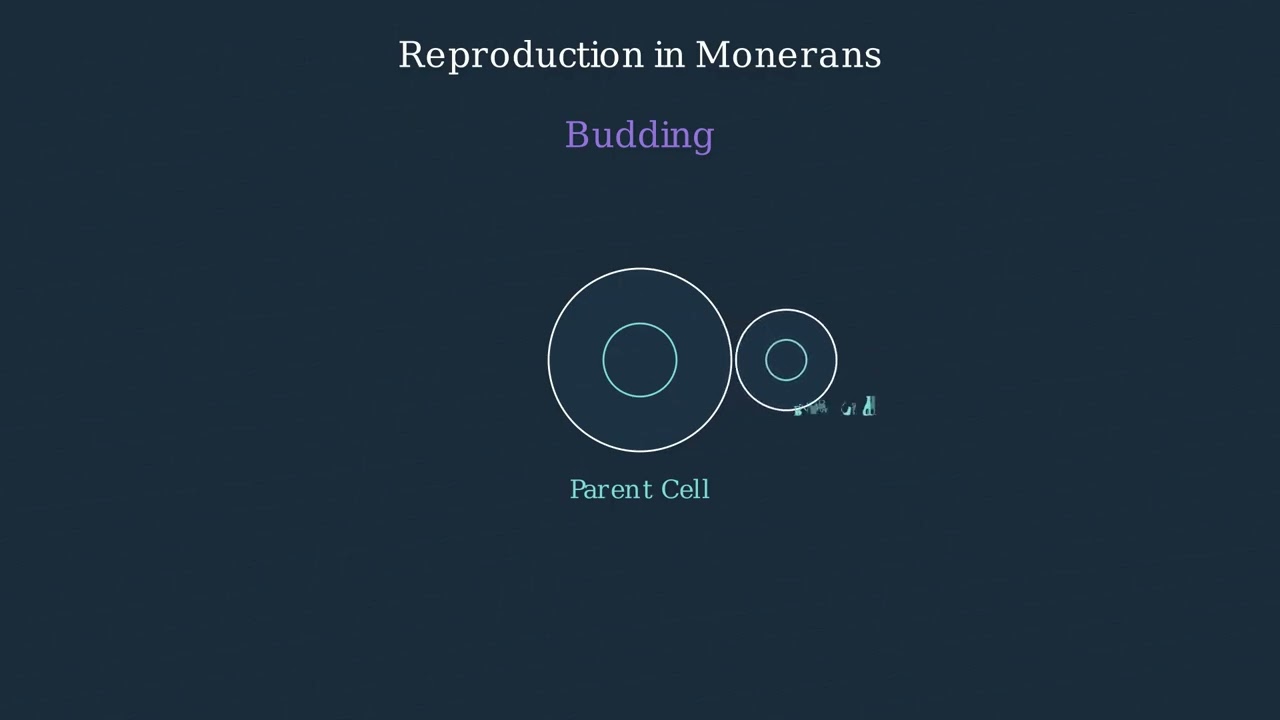
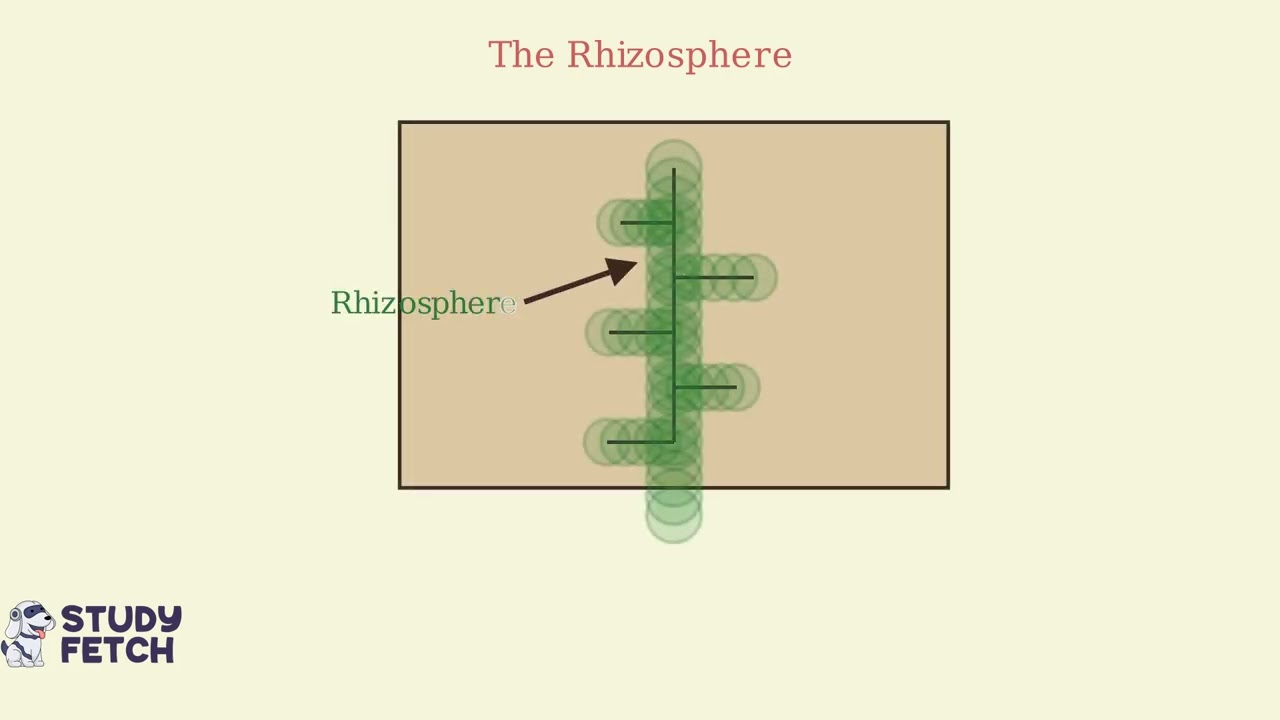
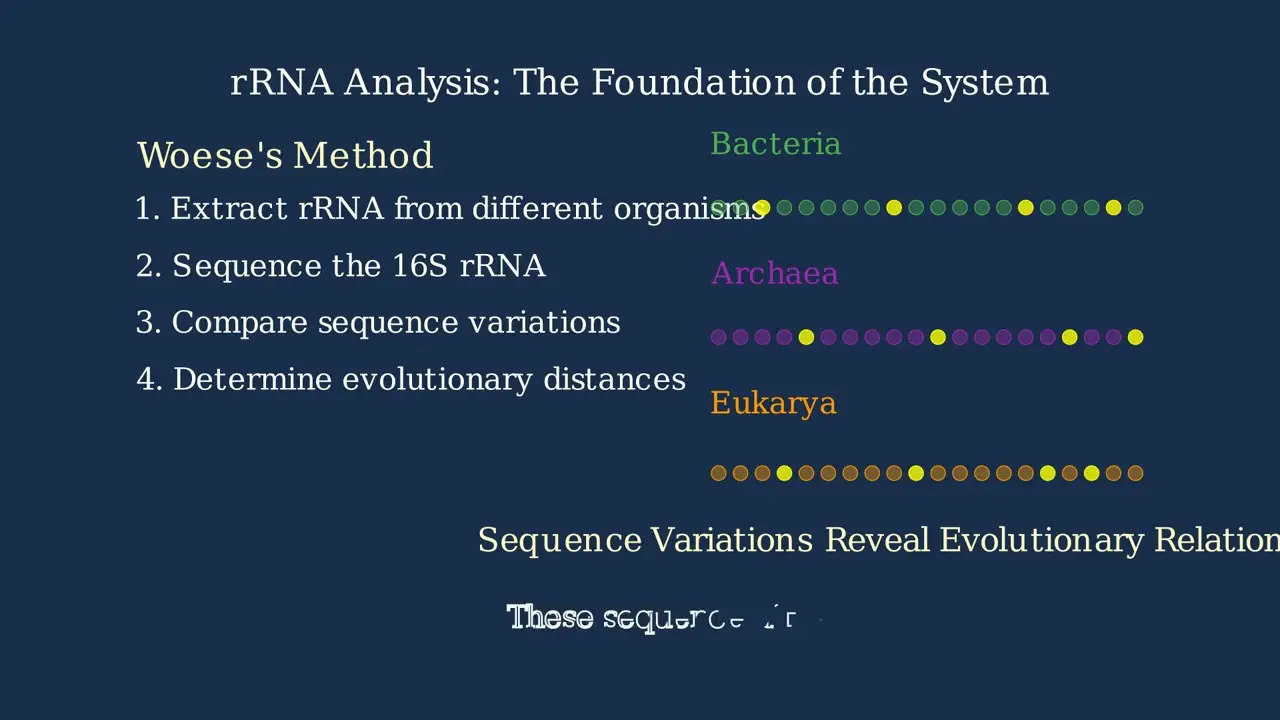
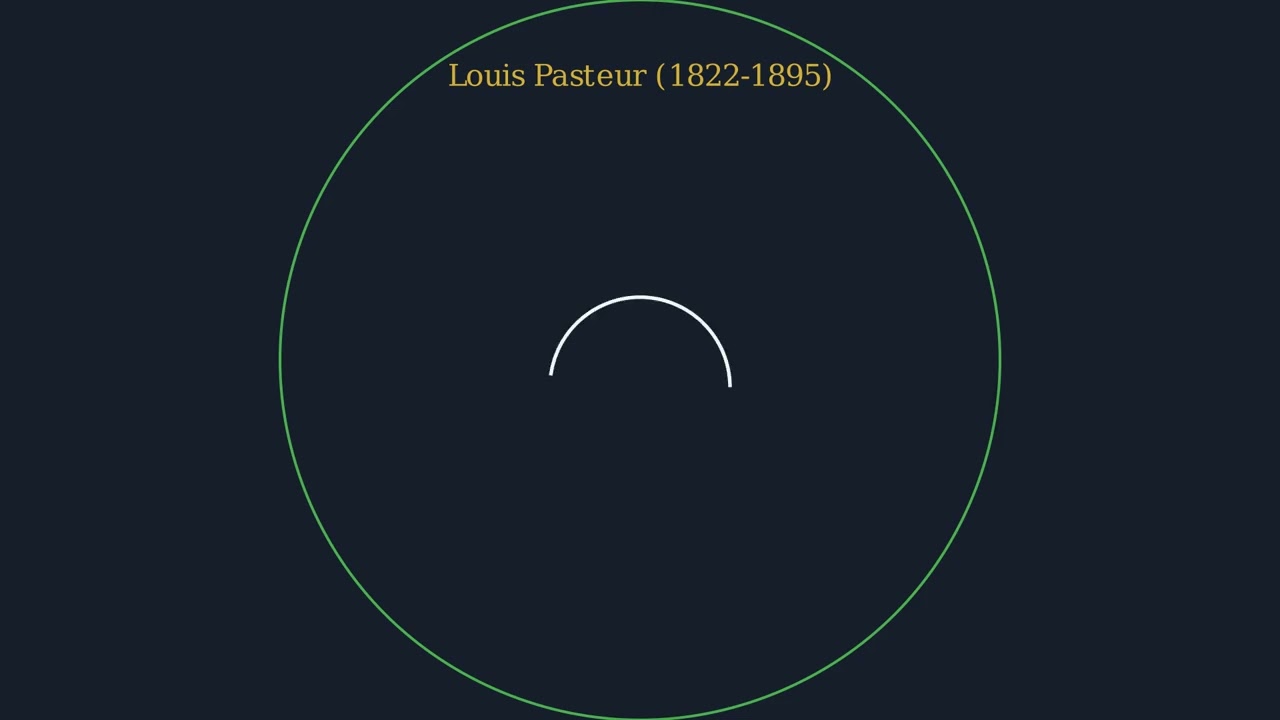
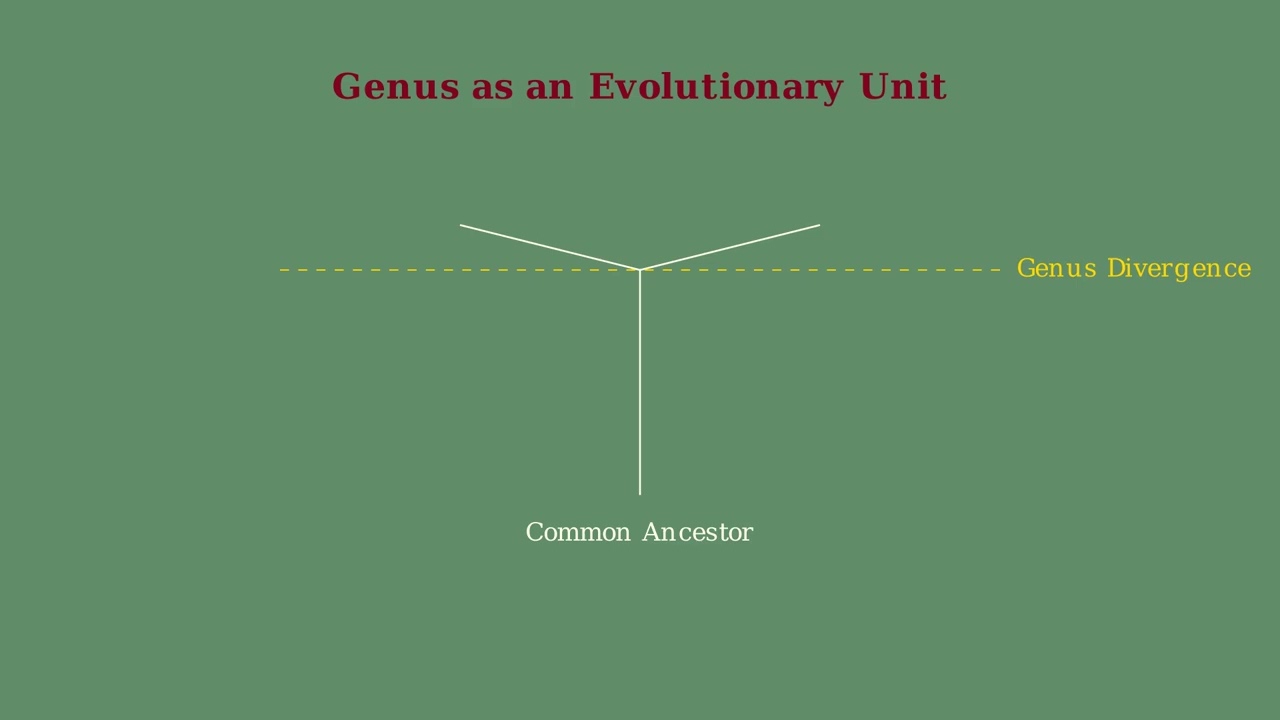