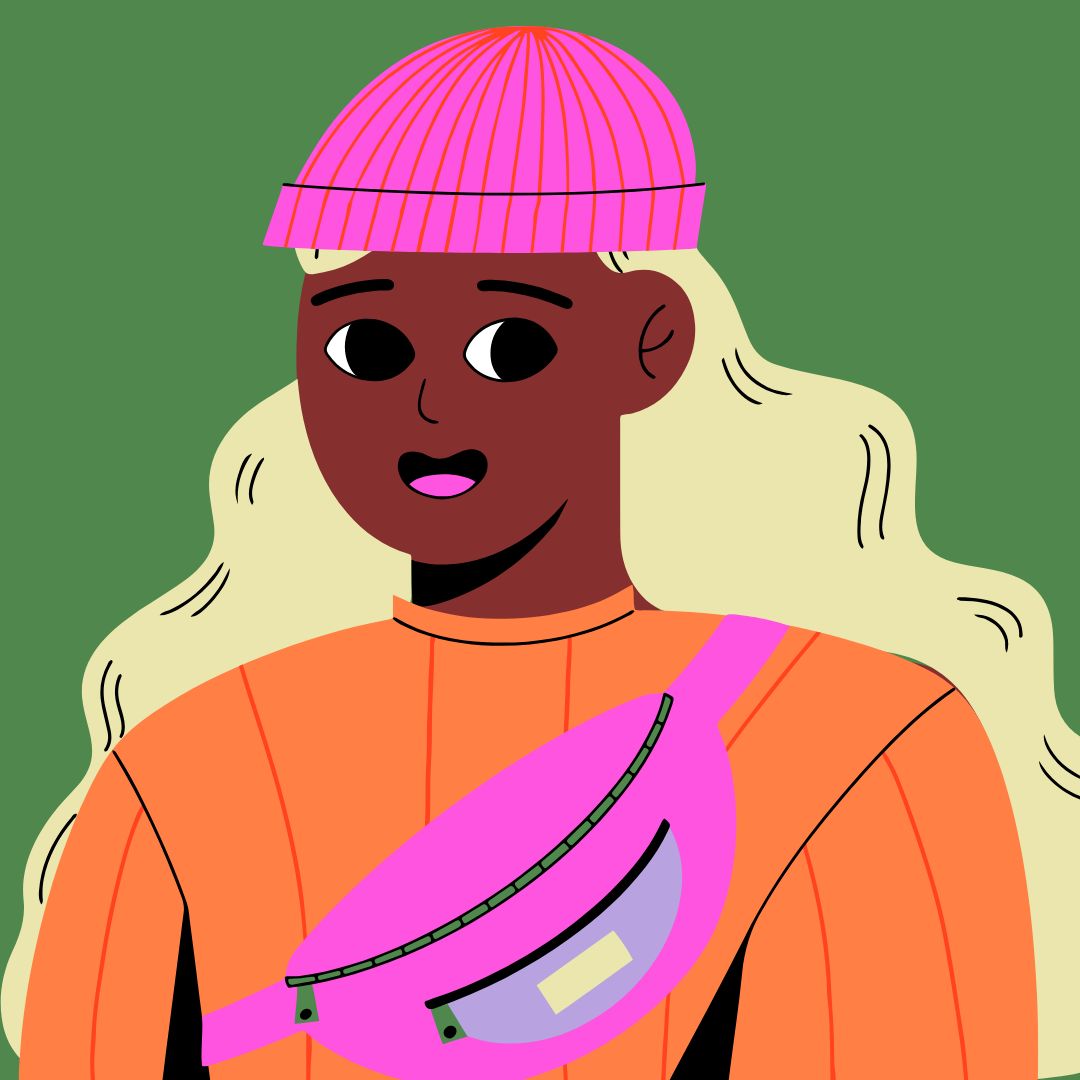
Sourav Pan
Transcript
Simple diffusion is a type of passive transport where molecules move across permeable barriers based on electrochemical potential differences.
It involves the movement of solutes from areas of high concentration to areas of low concentration.
This process requires no energy input, unlike active transport mechanisms that consume ATP.
The diffusion process continues until the concentration equalizes throughout the available space, reaching an equilibrium state.
Brownian motion is the random, erratic movement of particles suspended in a fluid.
This phenomenon, first observed by botanist Robert Brown in 1827, results from particles being bombarded by the surrounding medium’s molecules.
Let’s visualize this process. Here, the orange particles represent suspended particles, while the tiny blue dots represent the fluid molecules.
The suspended particles move erratically as they’re constantly bombarded by the fluid molecules from all directions.
These random movements have a powerful cumulative effect. Over time, they cause particles to spread evenly throughout the available space.
This is why particles naturally move from areas of high concentration to areas of low concentration, even though individual particle movements are random.
Although each particle moves randomly due to molecular collisions, the overall statistical tendency is for them to distribute evenly.
The key takeaway is that the randomness of molecular collisions is the driving force behind simple diffusion – a fundamental process in chemistry and biology.
Diffusion is a continuous process that proceeds until it reaches a state called equilibrium.
Equilibrium is reached when the concentration of molecules becomes uniform throughout the system.
When allowed to diffuse freely, molecules move from areas of high concentration to areas of low concentration.
As diffusion continues, we eventually reach equilibrium where molecules are evenly distributed.
At equilibrium, although molecules continue to move randomly due to Brownian motion, there is no net movement in any particular direction.
This state of equilibrium represents the maximum entropy, or disorder, of the system. It is the most energetically stable state.
While molecules continue to move in all directions, the rates of movement between any two areas are equal, resulting in zero net flux.
Remember that equilibrium doesn’t mean molecules stop moving; it simply means there is no net directional movement. The system has reached its most stable state.
The concentration gradient is the difference in concentration between two regions.
Here we can see a container with a high concentration of molecules on the left and a low concentration on the right.
The difference in concentration between these two regions creates a concentration gradient that drives diffusion.
The steeper the concentration gradient, the faster diffusion occurs.
Notice how molecules move much faster from high to low concentration when the gradient is steeper.
As molecules move from high to low concentration areas, the gradient gradually decreases until it reaches zero at equilibrium.
This concentration gradient is the primary driving force behind simple diffusion. Without a gradient, diffusion stops.
Molecular size significantly impacts how quickly substances diffuse.
Smaller molecules diffuse faster than larger ones because they encounter less resistance when moving through a medium.
This is because smaller molecules have less surface area, and therefore experience less drag when moving through the medium.
When molecules move through a medium, they encounter resistance from surrounding particles and structures.
Smaller molecules can navigate more easily between these obstacles, facing less resistance.
Larger molecules, however, have more difficulty navigating through the same space and encounter more resistance.
This relationship between molecular size and diffusion rate is described by Graham’s Law of Diffusion.
According to Graham’s Law, the rate of diffusion of a gas is inversely proportional to the square root of its molecular mass.
This is why gases like oxygen diffuse much more rapidly than larger molecules like proteins.
For example, oxygen with a molecular mass of 32 Daltons diffuses about 45 times faster than hemoglobin with a mass of 64,500 Daltons.
This relationship between molecular size and diffusion rate is crucial in biological systems, affecting everything from cellular respiration to drug delivery.
Understanding how molecular size affects diffusion helps us explain many natural processes and design more effective technologies.
Solubility and its impact on diffusion
Solubility refers to how readily a substance dissolves in a particular solvent.
Molecules that are more soluble in a membrane can diffuse through it more easily.
Cell membranes consist of a phospholipid bilayer, which has both hydrophobic and hydrophilic regions.
Let’s examine how different types of molecules interact with the cell membrane.
Lipid-soluble molecules can easily pass through the cell membrane because they can dissolve in the hydrophobic lipid bilayer.
In contrast, water-soluble molecules struggle to pass through the hydrophobic regions of the membrane.
This principle of solubility has important applications in drug design.
Many medications are designed to be lipid-soluble to improve their ability to cross cell membranes and reach their targets inside cells.
Examples of lipid-soluble drugs include aspirin, steroids, and various anesthetics.
Let’s summarize how solubility impacts diffusion across cell membranes.
Substances with high membrane solubility diffuse quickly and easily through membranes. This includes oxygen, carbon dioxide, and many hormones.
In contrast, substances with low membrane solubility, like ions and glucose, diffuse slowly and require specialized transport proteins to cross membranes efficiently.
Understanding solubility is crucial for predicting how molecules will move across biological membranes, which has significant implications in physiology, medicine, and biotechnology.
The density of the solvent medium significantly affects how molecules move through it during diffusion.
Diffusion occurs at different rates depending on the state of matter. Let’s examine how molecules move in gas, liquid, and solid environments.
In gases with low density, molecules move rapidly with minimal resistance, resulting in fast diffusion.
In liquids with medium density, molecules encounter more resistance, slowing their movement and reducing diffusion speed.
In solids with high density, molecules face significant resistance, greatly limiting their movement and resulting in very slow diffusion.
The key concept is that denser solvents create more resistance, which leads to slower diffusion rates.
This principle is critical in many industrial applications. For example, pharmaceutical companies carefully select solvent densities to control how quickly medications release their active ingredients.
Understanding solvent density effects allows scientists and engineers to control diffusion rates in various applications, from drug delivery systems to industrial manufacturing processes.
Surface area and membrane thickness are critical factors that directly influence diffusion rates.
Larger surface areas allow more molecules to diffuse simultaneously, significantly increasing the overall rate of diffusion.
Consider these two containers – one narrow with a small surface area, and one wide with a large surface area.
With three times the surface area, the wider container allows more molecules to diffuse simultaneously, resulting in a much higher overall diffusion rate.
Conversely, membrane thickness has an opposite effect on diffusion.
Thicker membranes slow diffusion by increasing the distance molecules must travel to get from one side to the other.
Notice how particles move through the thin membrane more quickly than through the thick membrane, where they encounter greater resistance.
This is why many biological structures have evolved to maximize surface area through folding or branching, while minimizing membrane thickness.
In the lungs, tiny air sacs called alveoli create an enormous surface area for gas exchange. The human lungs contain about 500 million alveoli, providing a surface area of around 70 square meters.
Similarly, the small intestine uses finger-like projections called villi to dramatically increase the surface area available for nutrient absorption. This adaptation increases the functional surface area by up to 600 times.
Remember that surface area and membrane thickness work together to determine how efficiently diffusion occurs. In both natural and engineered systems, these principles guide the design of structures where diffusion is critical.
Pharmaceutical companies carefully design medications with diffusion principles in mind.
After administration, drugs enter the bloodstream and must diffuse across various barriers to reach their target tissues.
The rate of diffusion is critical – it determines how quickly a medication takes effect and how long it remains active in the body.
Several key factors affect how drugs diffuse through the body: molecular size, solubility, concentration gradient, and tissue barriers.
Understanding diffusion principles helps pharmaceutical companies develop different drug delivery systems.
Immediate release formulations deliver the full dose of medication at once, causing a rapid increase in drug concentration.
Controlled-release formulations, on the other hand, use special matrices or coatings to release medication gradually over time.
This graph compares how drug concentration changes over time with different formulations.
Immediate release formulations quickly reach peak concentration but may fall below therapeutic levels rapidly.
Controlled-release formulations maintain drug levels within the therapeutic range for a longer period, improving effectiveness and reducing side effects.
By understanding and applying diffusion principles, pharmaceutical scientists can design medications that maintain therapeutic drug levels over extended periods, improving patient outcomes.
Environmental scientists study air pollution dispersal to understand how pollutants spread through the atmosphere.
When pollutants are released from sources like factories, they spread through diffusion from areas of high concentration to areas of lower concentration.
This natural process is driven by random molecular motion, causing particles to gradually spread away from the source.
As pollutants disperse, they create a concentration gradient. The concentration is highest near the source and decreases with distance.
Several factors influence how pollutants disperse through the air.
Temperature affects the rate of dispersal. Higher temperatures increase the kinetic energy of particles, causing faster and more extensive diffusion.
Wind plays a crucial role by accelerating the dispersal process and determining the direction of pollution spread.
The pattern and extent of pollution dispersal determines its environmental impact.
This includes effects on air quality in surrounding areas, deposition of pollutants on land and water bodies, and exposure risks for ecosystems and human populations.
Understanding how pollutants disperse through diffusion is essential for developing effective environmental protection strategies and public health policies.
Let’s compare passive transport, like simple diffusion, with active transport.
In passive transport, molecules move across a membrane with no energy input.
This relies solely on the kinetic energy of the molecules and follows the concentration gradient – from high to low concentration.
Active transport, however, requires energy input in the form of ATP.
This energy allows molecules to be transported against their concentration gradient – from low to high concentration.
Let’s examine the key differences between these transport mechanisms.
While passive transport like simple diffusion is energy-efficient, it is limited to moving molecules from areas of high concentration to low concentration.
Active transport can work against concentration gradients, but requires ATP energy, making it less energy-efficient.
Both transport mechanisms play essential roles in cellular function, with simple diffusion providing energy-efficient transport along concentration gradients.
Simple diffusion is unique because it doesn’t require the help of membrane proteins.
At the heart of this concept is the phospholipid bilayer of cell membranes.
Simple diffusion involves the direct movement of molecules through the phospholipid bilayer without any assistance.
Small, uncharged molecules can pass directly through the lipid portion of the membrane, moving from areas of high concentration to areas of low concentration.
Let’s compare simple diffusion with facilitated diffusion to understand the key differences.
Simple diffusion occurs directly through the phospholipid bilayer, while facilitated diffusion requires transport proteins embedded in the membrane.
In simple diffusion, small molecules like oxygen and carbon dioxide move directly through the phospholipid bilayer.
In contrast, facilitated diffusion requires specific transport proteins to help larger or charged molecules cross the membrane.
Let’s highlight the key distinctions that make simple diffusion unique.
Simple diffusion doesn’t require any transport proteins. It works best for small, uncharged molecules like oxygen and carbon dioxide.
Like all diffusion processes, it follows concentration gradients from high to low. Its rate is limited by factors like molecular size and membrane properties.
Let’s look at some common examples of molecules that use simple diffusion to cross cell membranes.
Oxygen and carbon dioxide are classic examples of molecules that use simple diffusion to cross cell membranes.
Water also uses simple diffusion, though it’s primarily regulated by osmosis. Other small lipid-soluble molecules can diffuse directly through the membrane as well.
To summarize, simple diffusion is a passive transport process that allows molecules to move directly through the phospholipid bilayer without the assistance of membrane proteins.
This independence from membrane proteins distinguishes simple diffusion from facilitated diffusion, though both follow concentration gradients.
Scientists use various techniques to measure diffusion rates in different systems.
Three primary techniques include fluorescent markers, which allow tracking of labeled molecules through fluorescence microscopy.
Radioactive tracers, which use isotopes to monitor molecule movement across membranes and tissues.
And specialized imaging methods such as FRAP, FRET, and other advanced techniques that capture diffusion in real-time.
Fick’s laws of diffusion provide the mathematical framework for understanding and measuring diffusion rates.
Fick’s first law states that the diffusion flux is proportional to the concentration gradient. This helps calculate the amount of substance flowing through a unit area per unit time.
Fick’s second law describes how concentration changes over time, which is proportional to the second derivative of concentration with respect to position.
These equations include variables for diffusion flux, the diffusion coefficient, concentration, position, and time.
These measurement techniques and mathematical models help scientists understand and quantify diffusion in various practical applications.
In biology, diffusion rate measurements help understand nutrient transport across cell membranes and tissues.
Industrial engineers use diffusion measurements to optimize equipment design for gas exchange, mixing processes, and material development.
In pharmaceutical research, diffusion rate measurements are crucial for determining drug release rates from delivery systems and predicting drug distribution in the body.
Let’s examine a common technique called Fluorescence Recovery After Photobleaching, or FRAP.
In this technique, a small region containing fluorescent molecules is exposed to intense light, causing photobleaching.
As time passes, unbleached fluorescent molecules from surrounding areas diffuse into the bleached region.
The rate of fluorescence recovery directly correlates with the diffusion rate, allowing scientists to calculate precise diffusion coefficients.
To summarize, scientists use various techniques to measure diffusion rates, with Fick’s laws providing the mathematical foundation for these measurements.
These measurements help researchers understand biological processes, design industrial equipment, and develop pharmaceutical products with greater precision.
Plants rely on diffusion for nutrient uptake from the soil. Let’s examine how this critical process works.
The root system of plants, especially the tiny root hairs, provides an extensive surface area for nutrient absorption.
Essential nutrients like nitrogen, phosphorus, potassium, and water are present in the soil at various concentrations.
These nutrients diffuse from areas of high concentration in the soil to areas of lower concentration inside the root hairs, following concentration gradients.
Plants use two main mechanisms for nutrient uptake. Passive diffusion occurs naturally, requiring no energy from the plant.
However, for some essential nutrients, especially when soil concentrations are low, plants must use active transport, which requires energy.
Understanding diffusion in plant nutrition has led to several important agricultural practices that enhance crop yields.
By optimizing conditions for diffusion, farmers can significantly improve nutrient uptake efficiency and ultimately increase crop yields.
Diffusion principles have applications far beyond natural processes, forming the basis for numerous technological innovations.
Dialysis machines rely on diffusion to filter waste products from blood. As blood flows through the dialyzer, waste molecules diffuse across a semipermeable membrane due to concentration differences.
Controlled-release fertilizers utilize diffusion principles to gradually deliver nutrients to plants. A polymer coating controls the rate at which nutrients diffuse into the soil, providing sustained nutrition.
Gas sensors detect chemicals based on their diffusion properties. Target gas molecules diffuse through a selective membrane at specific rates, enabling precise detection of compounds like carbon monoxide or methane.
Understanding diffusion principles has led to numerous practical innovations across multiple industries. From pharmaceutical delivery systems to water purification membranes, diffusion science continues to drive technological advancement.
Simple diffusion is a fundamental process that occurs throughout nature.
It has countless applications across many disciplines, from science and medicine to industry and technology.
What makes diffusion so important is that it occurs naturally without energy expenditure, driven by concentration gradients, and influenced by factors like temperature and molecular size.
Understanding this basic principle helps us develop more effective medications, innovative technologies, and sustainable solutions to various challenges.
In conclusion, simple diffusion shapes our world in ways both visible and invisible, underlying countless processes essential to life and technology.
Study Materials
No study materials available for this video.
Helpful: 0%
Related Videos
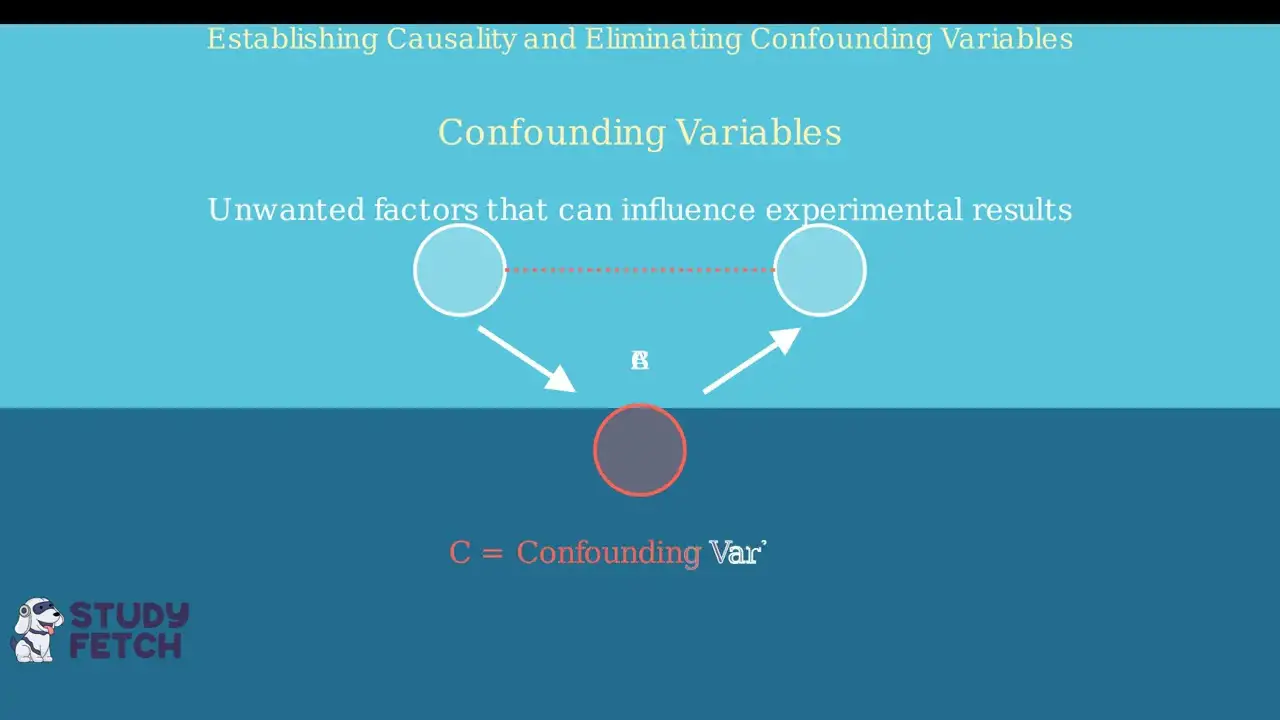
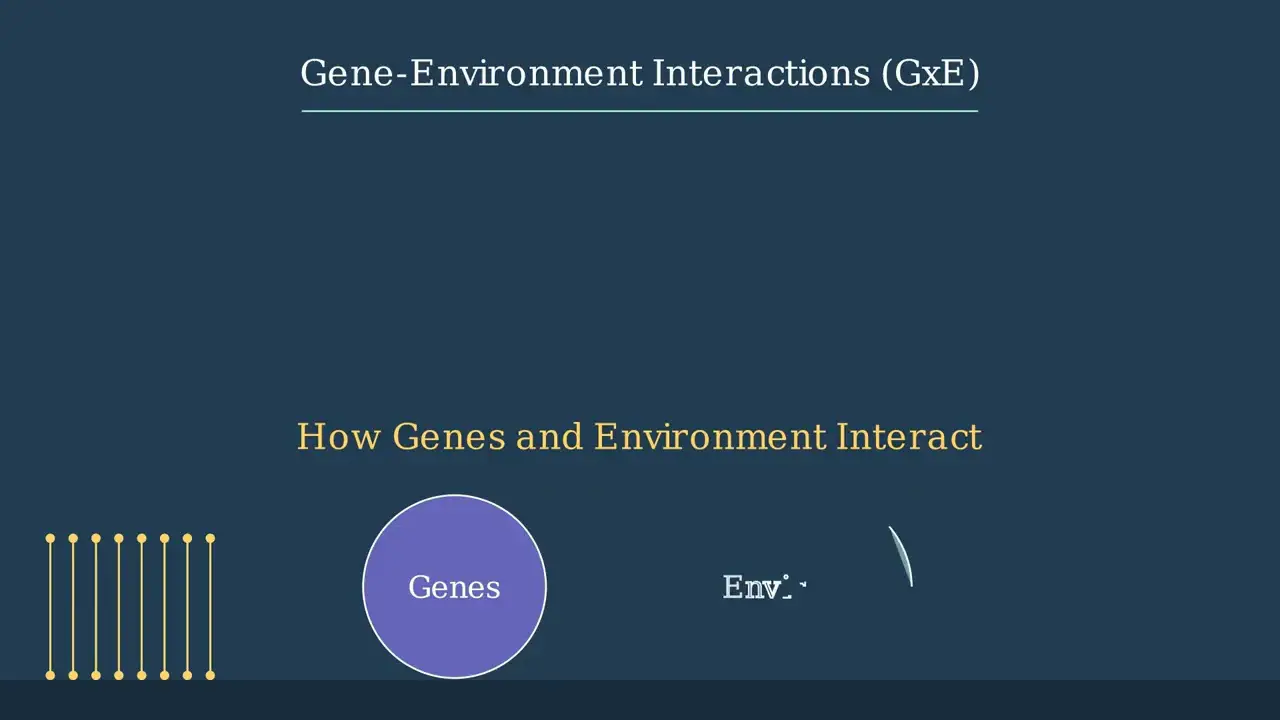
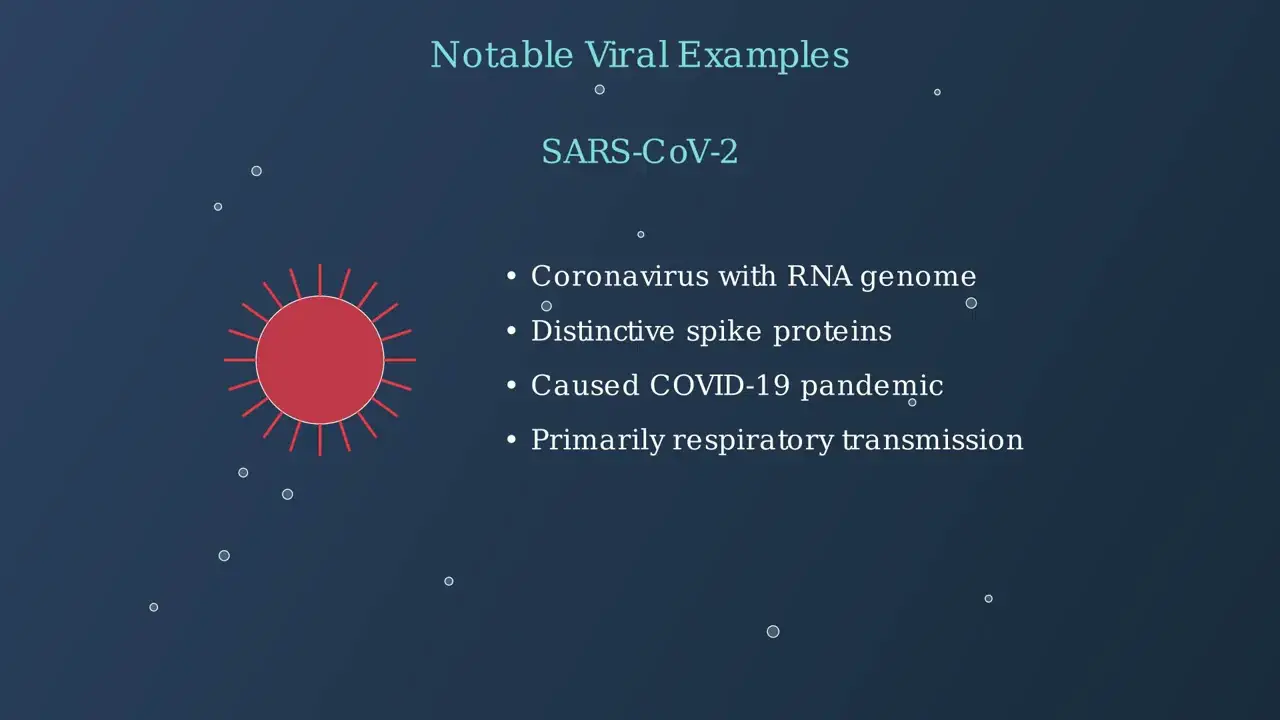
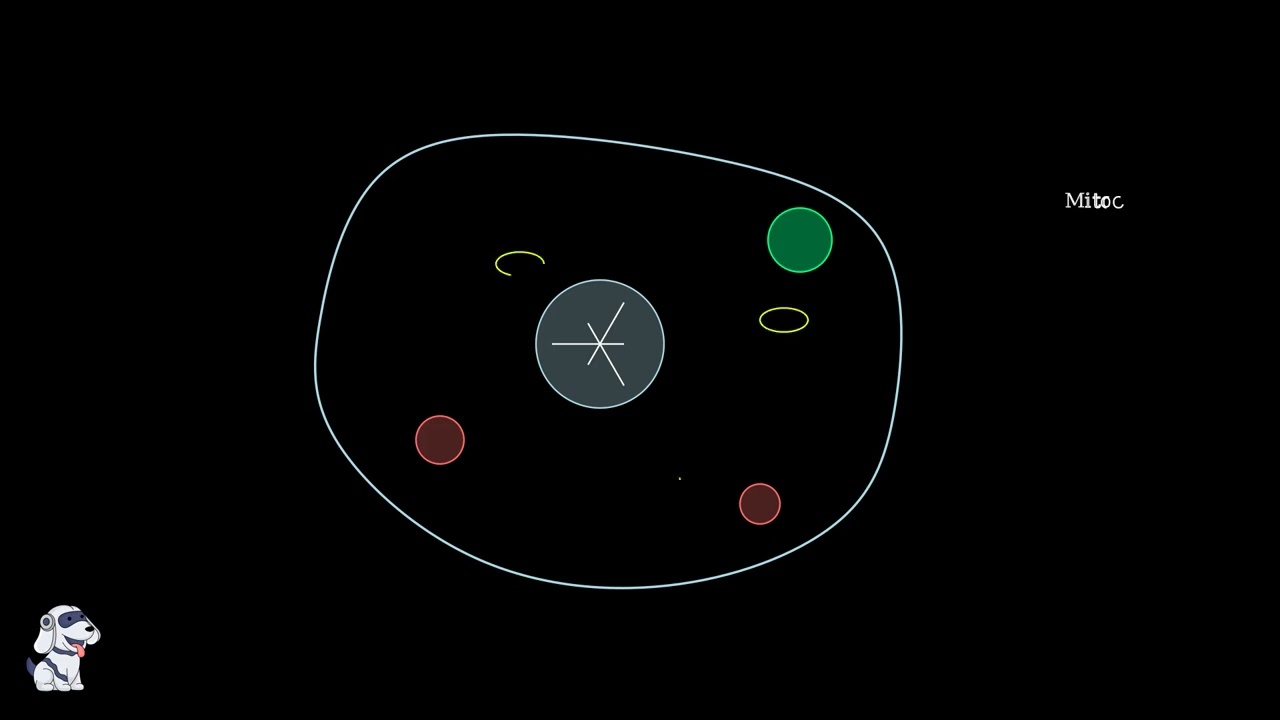
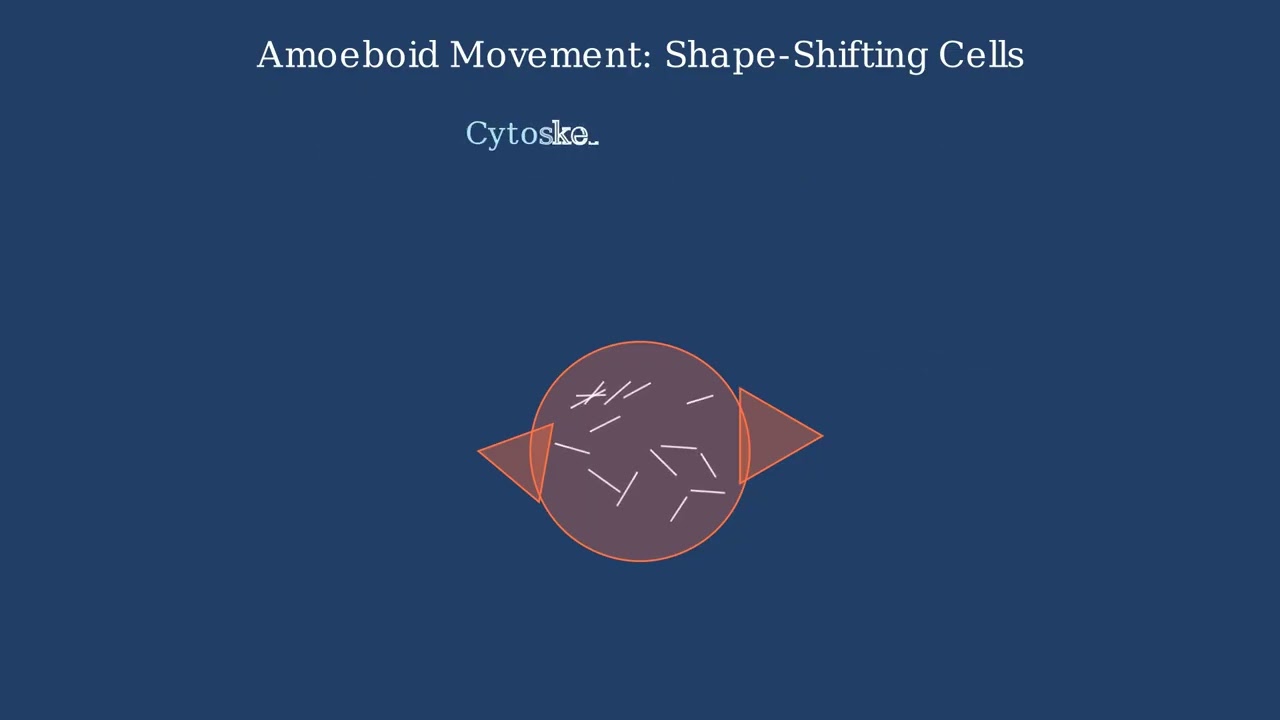
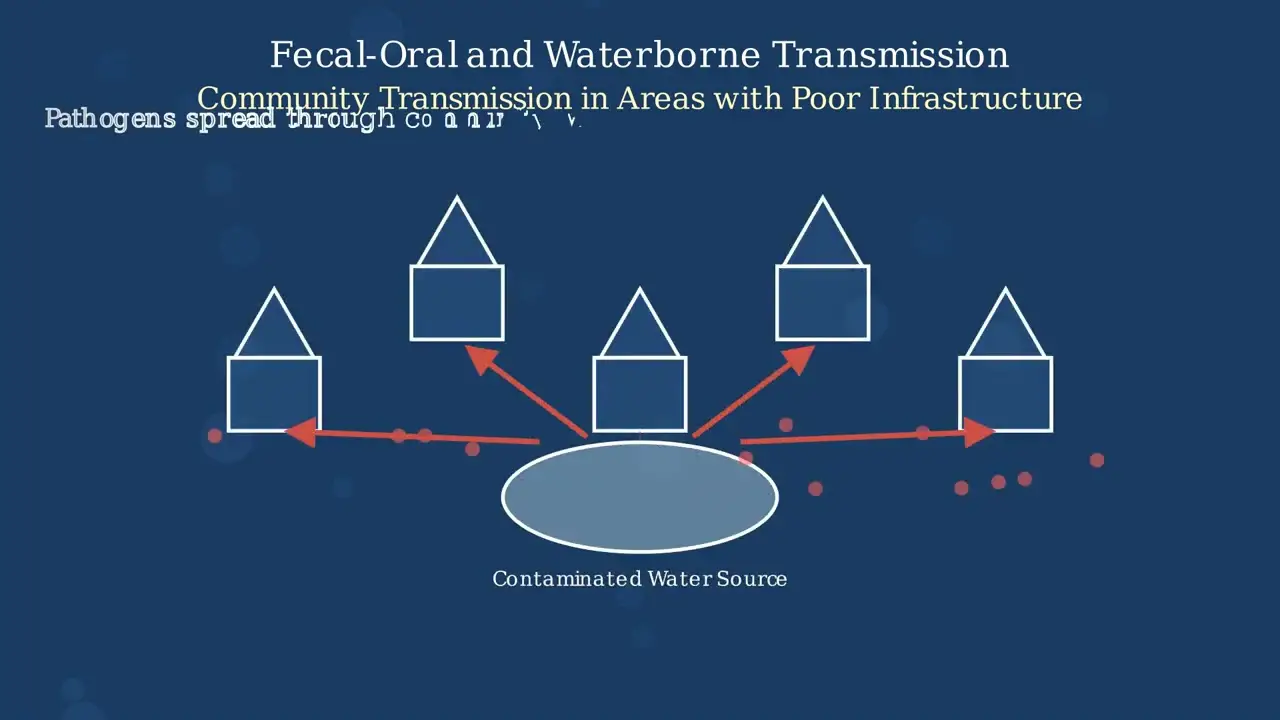
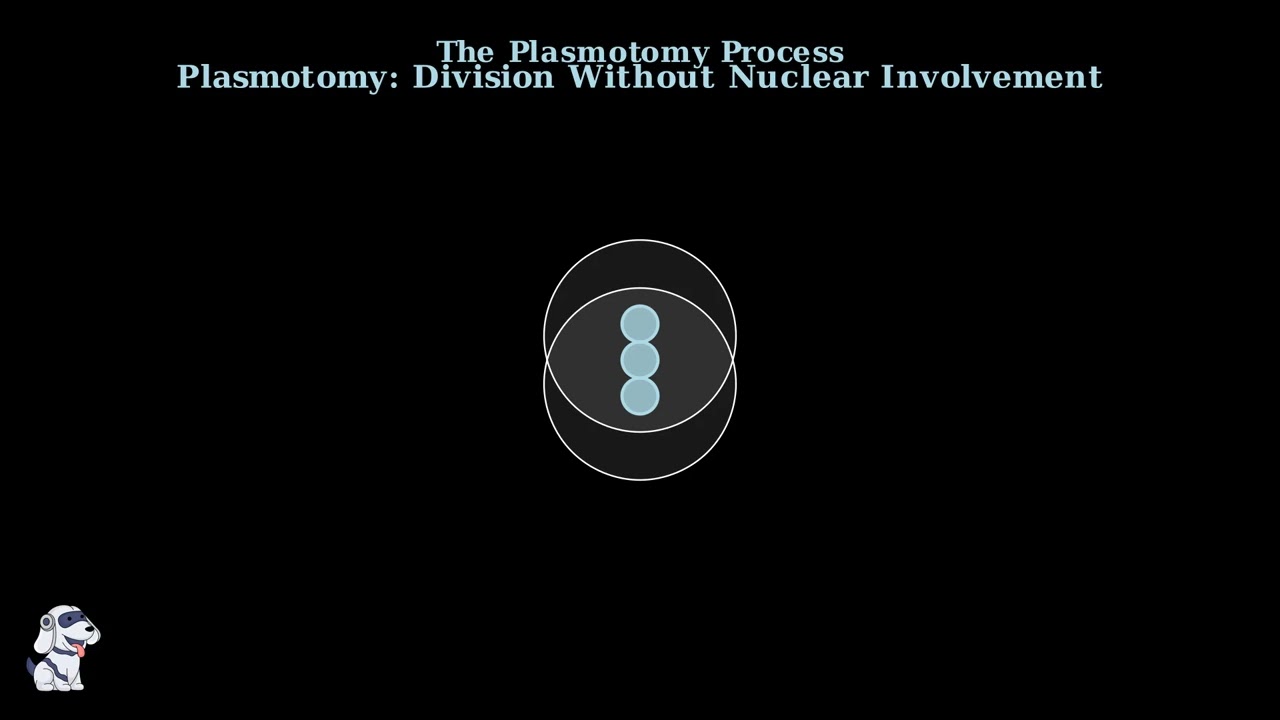
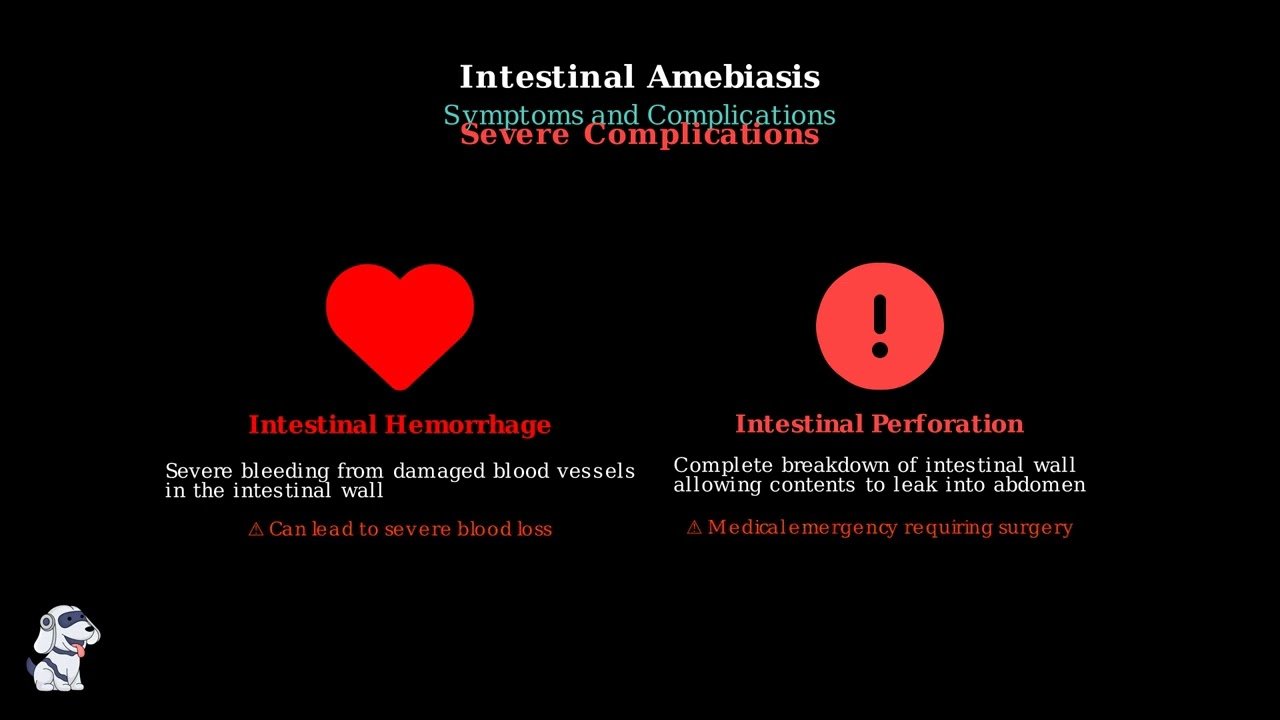
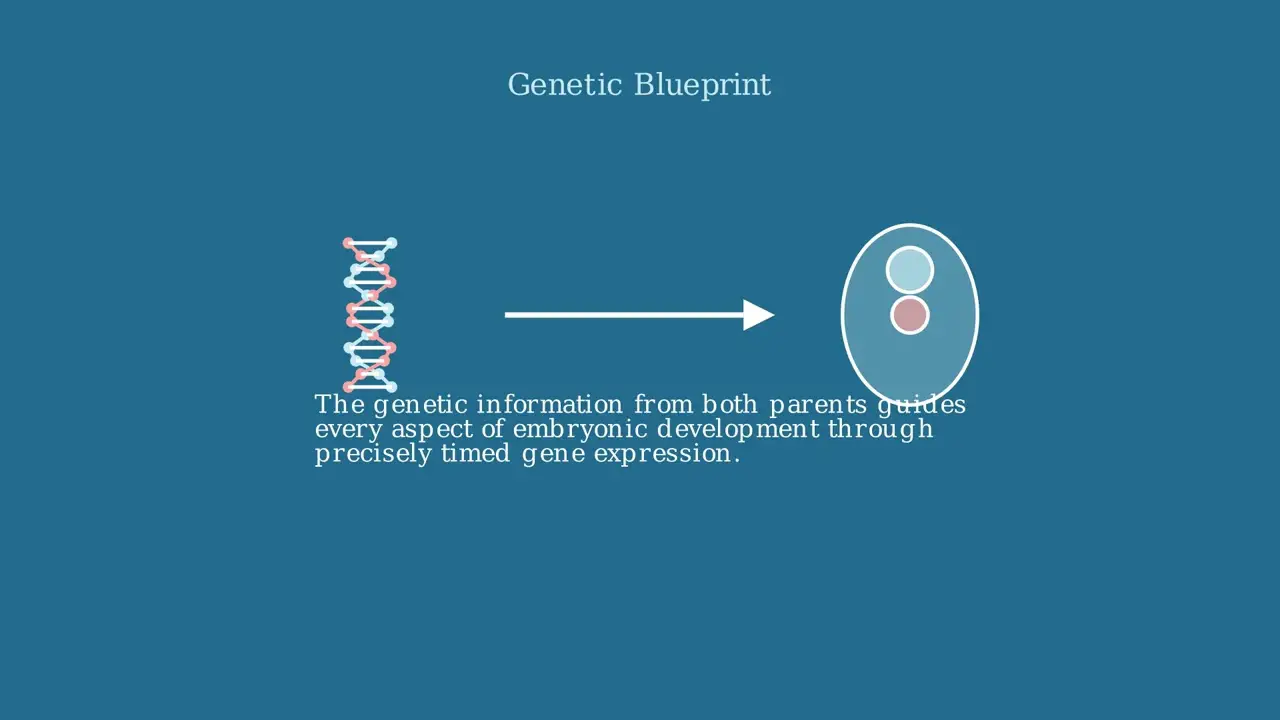
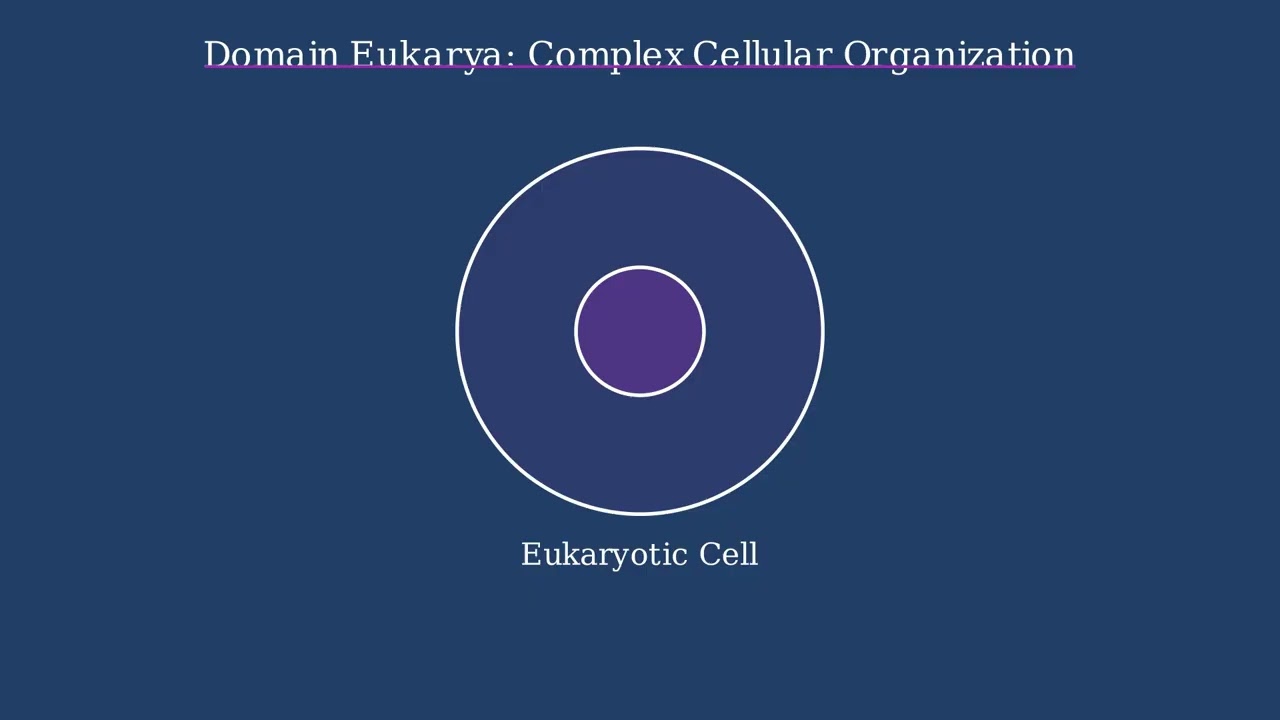