- The transfer of genes across plant species has significantly contributed to crop enhancement for many years. Traditional plant improvement has relied on both natural selection and the deliberate efforts of plant breeders. This process typically involves evolving, evaluating, and selecting favorable combinations of alleles that provide beneficial traits, such as resistance to diseases, insects, and environmental stresses. Over time, useful genes have been successfully transferred from wild relatives to cultivated crops, expanding the genetic diversity and adaptability of agricultural varieties.
- Since the 1970s, there has been a marked acceleration in the development of techniques for manipulating plant genetic information, primarily through recombinant DNA (rDNA) technology. This transformative process encompasses several key steps: the introduction of foreign genes into a plant, their integration into the host genome, and the subsequent expression of these genes. Plants that incorporate and stably express these foreign genes are known as transgenic plants. The creation of transgenic varieties is achieved through the integration of rDNA technology, gene transfer methods, and tissue culture techniques, all of which have broadened the potential for enhancing food, fiber, vegetable, fruit, and forest crops.
- Recent advancements in plant biotechnology have emerged as powerful tools for addressing longstanding agricultural challenges. This innovation enables the cloning of plant genes, the deciphering of genetic regulatory signals, and the transfer of genes from entirely unrelated organisms, including bacteria and viruses. These methods allow for the introduction of specifically desirable traits into crop plants without the accompanying transfer of undesirable genes that often occurs with traditional breeding methods. Thus, genetic transformation serves as a precise tool for improving agricultural outcomes.
- The pioneering work in plant transformation was demonstrated in tobacco through two main approaches: Agrobacterium-mediated transformation and vectorless methods. These methods have expanded the repertoire of plant species that can be transformed, with over 120 species from at least 35 families now capable of undergoing transformation. The success of these techniques spans major economic crops, vegetables, ornamental plants, medicinal species, fruits, trees, and pasture plants. As a result, the ability to transfer genes and regenerate transgenic plants is no longer a limiting factor in the development of effective transformation systems for many species.
- In particular, the early assumption that monocotyledonous plants could not be transformed using Agrobacterium tumefaciens has led to the development of direct DNA introduction techniques or vectorless methods. However, recent advancements have demonstrated successful Agrobacterium-mediated transformation in important monocot species such as rice, maize, and wheat. These breakthroughs have opened new avenues for enhancing crop traits and increasing yields.
- The initial applications of genetic engineering in agriculture focused on developing transgenic plants that express foreign genes conferring resistance to viruses, insects, herbicides, and reducing post-harvest deterioration. Furthermore, some modifications have aimed at enhancing the nutritional quality of storage products. Such innovations underscore the potential of transgenic technologies to revolutionize crop production and food security.
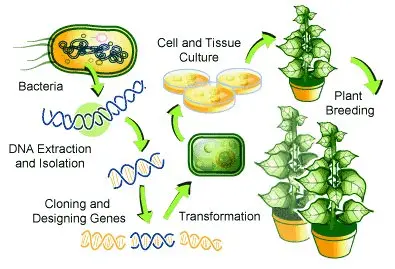
Resistance of transgenic crops to biotic stresses
Resistance to biotic stresses has been discussed under the following headings.
- Insect resistance
- Viral resistance
- Fungal and bacterial disease resistance
Insect resistance of transgenics crops
The development of transgenic crops with insect resistance represents a significant advancement in agricultural biotechnology. By incorporating specific insect control genes, particularly those derived from Bacillus thuringiensis (Bt), researchers have successfully engineered plants that exhibit enhanced resistance to various insect pests. The following points provide an in-depth understanding of the mechanisms, genes involved, and the progress achieved in this area.
- Introduction to Insect Resistance in Transgenic Crops
Insect resistance in transgenic crops has been notably advanced through the use of genes from Bacillus thuringiensis. Initial reports of insect resistance were seen in tobacco and tomato plants in the late 1980s. Since then, approximately 40 different insect resistance genes have been successfully incorporated into various crop species. These genes originate from diverse sources, including microorganisms and higher plants. - Sources of Resistance Genes
- Microorganisms:
- Bt Gene: The most well-known insect resistance gene is derived from Bacillus thuringiensis, an entomocidal bacterium that produces proteins toxic to certain insects.
- Cholesterol Oxidase Gene: This gene, sourced from Streptomyces cultures, has demonstrated toxicity against the boll weevil larvae and has been engineered into tobacco plants.
- Isopentenyl Transferase (ipt) Gene: Derived from Agrobacterium tumefaciens, this gene has been shown to reduce leaf consumption by tobacco hornworm larvae and decrease the survival rates of aphids.
- Higher Plants:
- Proteinase Inhibitors: These include genes such as the cowpea trypsin inhibitor (CpTI), which interferes with insect digestion by inhibiting digestive enzymes.
- Lectins: Plant-derived glycoproteins that can deter insect feeding. The snowdrop lectin (GNA) has shown effectiveness against aphids and has been integrated into various crops.
- α-Amylase Inhibitors: These inhibit the enzyme responsible for starch digestion in insects, effectively starving them.
- Microorganisms:
- Mechanism of Action of Bt Toxins
The insecticidal properties of Bacillus thuringiensis stem from the production of crystal proteins, known as δ-endotoxins, during sporulation. Upon ingestion by susceptible larvae, these proteins are activated and disrupt ion transport across the insect gut membranes, leading to the death of the insect. Different strains of Bt produce various toxins with specific activity against different insect groups, such as Lepidoptera, Diptera, and Coleoptera. - Progress in Transgenic Development
The cloning and sequencing of the first insecticidal protein genes in 1981 marked the beginning of the biotechnological approach to pest resistance. Over 100 crystal protein gene sequences have been identified since then. The expression of both full-length and truncated forms of these proteins has been achieved in several plant species, leading to the development of transgenic plants capable of synthesizing effective levels of insecticidal proteins. Initial generations of these transgenic plants have already been commercialized. - Resistance Mechanisms from Higher Plants
In addition to microbial genes, plant-derived resistance mechanisms have been explored extensively:- Proteinase Inhibitors: These disrupt the digestive processes of insects by blocking protein digestion. For example, CpTI from cowpeas has shown efficacy against multiple insect species, reducing their survival and the damage they cause.
- Lectins: While lectins like GNA have been expressed in several crops, their effectiveness as insecticides has been limited due to the requirement for high doses for efficacy.
- α-Amylase Inhibitors: These proteins specifically target the enzymes necessary for starch digestion, thereby preventing larval development in targeted pests.
- Resistance Genes from Animal Sources
Research has also explored the potential of animal-derived serine proteinase inhibitors. For instance, bovine pancreatic trypsin inhibitor (BPTI) and other inhibitors have been evaluated for their effectiveness in conferring insect resistance. Although some promising results were observed, challenges remain in achieving consistent resistance across different plant species. - Adoption and Impact
By 2007, insect-resistant transgenic crops covered approximately 18% of the global area under transgenic cultivation, with Bt cotton and Bt maize leading the way. This significant adoption underscores the effectiveness of transgenic approaches in managing pest populations and highlights their potential benefits for agricultural sustainability.
Viral resistance of transgenic crops
Viral resistance in transgenic crops is a crucial area of plant biotechnology aimed at combating the negative impacts of viral infections on agricultural productivity. By leveraging molecular strategies, scientists can introduce resistance traits into plants, thereby enhancing their ability to withstand viral pathogens. The following points outline the different strategies employed in creating transgenic crops resistant to viruses, as well as their mechanisms of action.
- Molecular Strategies for Viral Resistance:
The small genomic size of plant viruses facilitates the development of molecular strategies to control viral diseases. These strategies primarily focus on identifying viral genes or gene products that, when expressed improperly, disrupt the viral infection process. - Coat Protein Mediated Cross Protection:
- This strategy employs the concept of cross protection, where a mild virus strain inoculates a susceptible crop, providing protection against a more virulent strain.
- The seminal work by Powell-Abel et al. (1986) demonstrated that transgenic tobacco expressing the coat protein of Tobacco Mosaic Virus (TMV) exhibited resistance similar to natural cross protection.
- Various crops, including tobacco, tomato, potato, alfalfa, melons, and rice, have benefited from this approach. For example, Kouassi et al. (2006) showed that rice expressing the coat protein gene for Rice Yellow Mottle Virus exhibited significant resistance.
- The mechanism involves the coat protein inhibiting the uncoating of the challenge virus RNA, reducing infection sites and consequently preventing disease progression.
- Non-Structural Protein Mediated Resistance:
- This approach utilizes viral non-structural proteins necessary for replication.
- Golemboski et al. (1990) illustrated this by expressing the 54 kDa open reading frame of TMV in transgenic tobacco, resulting in enhanced resistance.
- Additional examples include the development of transgenic tobacco resistant to Pea Early Browning Virus (PEBV) and Potato Virus X (PVX).
- Antisense and Sense Mediated Resistance:
- The antisense strategy involves the expression of RNA sequences complementary to viral RNA, hindering its replication.
- Transgenic tobacco expressing complementary RNA to the coat protein has demonstrated effective viral inhibition.
- Sense constructs can also disrupt viral infection by altering the start codon of essential viral genes, thus preventing translation.
- Satellite RNA Protection:
- Satellite RNAs, which are small, single-stranded RNA molecules dependent on a helper virus, have shown potential in conferring resistance.
- Research by Tien et al. (1987) indicated that inoculating tobacco and other plants with a mild strain of Cucumber Mosaic Virus (CMV) alongside symptom-attenuating satellite RNA resulted in improved resistance and yield.
- Kim et al. (1997) further demonstrated this with hot pepper plants expressing CMV satellite RNA, confirming symptom attenuation upon viral infection.
- Defective Interfering RNAs (DI RNAs):
- DI RNAs are derived from viral genomes and can attenuate symptoms by competing with the full virus for replication resources.
- Studies have shown that transgenic plants expressing DI RNAs exhibit reduced symptoms following viral infection, particularly in cases like African Cassava Mosaic Virus (ACMV).
- Pathogen Targeted Resistance:
- Anti-viral proteins, particularly ribosome-inactivating proteins (RIPs) from plants like pokeweed, have been successfully expressed in transgenic plants, providing resistance against various viruses such as Potato Virus X (PVX) and Cucumber Mosaic Virus (CMV).
- The mammalian 2′→5′ oligoadenylate synthase gene has been utilized in plants to enhance resistance through the degradation of viral RNA, thus curtailing replication.
- Ribozyme technology, which uses RNA molecules to cleave viral RNA at specific sites, has also shown promise. Edington and Nelson (1992) demonstrated significant reductions in viral accumulation using ribozymes directed against TMV in tobacco.
- Examples of Transgenic Plants and Their Resistance Mechanisms:
- Various crops have been genetically engineered to exhibit resistance against specific viruses using different transgenes:
- Potato: Replicase protein provides competition for the replication enzyme against Potato Virus Y (PVY).
- Tobacco: Movement protein impedes transport, providing resistance against TMV.
- Tomato: Ribozyme technology has been utilized to cleave the RNA of CEVd, enhancing resistance.
- Various crops have been genetically engineered to exhibit resistance against specific viruses using different transgenes:
Fungal and bacterial disease resistance of transgenic crops
Transgenic crops are genetically modified plants designed to exhibit resistance to various diseases, enhancing agricultural productivity and sustainability. The integration of plant defense response genes into crops represents a significant advancement in biotechnology. These transgenic plants can effectively combat pathogens through a range of mechanisms, which include the expression of antimicrobial proteins, pathogenesis-related proteins, and phytoalexins, as well as the manipulation of specific disease resistance genes.
- Plant Defense Response Genes: A substantial number of plant defense genes that encode antimicrobial proteins have been cloned and studied. These genes are typically activated in response to infection or microbial elicitors. The resulting products from these defense genes include:
- Hydrolytic enzymes, such as chitinases and β-1,3-glucanases, which degrade components of the cell walls of fungal pathogens.
- Ribosome-inactivating proteins (RIPs) that inhibit protein synthesis in pathogens.
- Antifungal proteins (AFPs) that directly inhibit fungal growth.
- Enzymes that facilitate the production of antimicrobial phytoalexins, which are secondary metabolites that play a crucial role in plant defense.
- Other components such as wall-bound phenolics, osmotins, thionins, and lectins, which further bolster the plant’s defense mechanisms.
- Reactive oxygen species, such as hydrogen peroxide, which can damage pathogens and trigger defense responses.
- Pathogenesis-Related Proteins (PR Proteins): These proteins are low molecular weight compounds that accumulate significantly in infected tissues. Major classes include PR-1, PR-2 (β-1,3-glucanase), PR-3 (chitinases), and PR-5 (thaumatin-like proteins). The utilization of hydrolytic enzymes like chitinase has been pivotal in enhancing crop resistance. For instance, a chitinase gene from Serratia marcescens was integrated into tobacco, leading to improved resistance against Rhizoctonia solani. While the expression of β-1,3-glucanase alone has not shown significant resistance, its combination with chitinase has provided substantial fungal resistance in various plant species.
- Antimicrobial Proteins: Beyond induced defenses, many plants inherently contain antimicrobial proteins. For example, the barley α-thionin gene, when transferred to tobacco, conferred resistance to Pseudomonas syringae. Transgenic crops have also incorporated lytic peptides, such as cecropins and attacins, which form pores in bacterial membranes, leading to bacterial cell death. The expression of the bacteriophage T4 lysozyme gene in potato has conferred resistance to Erwinia carotovora, demonstrating the potential of such proteins in crop protection.
- Active Oxygen Species (AOS): Transgenic plants that express fungal genes for glucose oxidase have shown elevated hydrogen peroxide levels, enhancing resistance to both fungal and bacterial pathogens. For instance, Brassica napus expressing the oxalate oxidase (OXO) gene exhibited significant resistance to Sclerotinia sclerotiorum, effectively detoxifying oxalic acid secreted by the pathogen.
- Phytoalexins: These low molecular weight metabolites are synthesized in response to pathogen attack. They serve as antimicrobial agents. The introduction of stilbene synthase (STS) genes from peanut into tobacco has led to the production of resveratrol, which exhibits fungitoxic properties. This manipulation is extended to other crops, including rice and Brassica napus.
- Manipulation of Disease Resistance Genes: Advances in map-based cloning and gene tagging have enabled the isolation of specific disease resistance (R) genes. For example, the maize HM1 gene, which confers resistance to Cochliobolus carbonum, has been successfully cloned. Other notable R genes include those from Arabidopsis, tomato, and rice. The introduction of these genes into susceptible varieties has been effective; for instance, transgenic tomato plants expressing the Pto resistance gene showed resistance to Pseudomonas syringae.
- Hypersensitive Response and Elicitor Production: Engineering a hypersensitive cell death response to fungal attacks has shown promise. A strategy utilizing the barnase-barstar system demonstrated that the selective expression of these proteins can enhance disease resistance by inducing localized cell death at infection sites. Transgenic tobacco plants expressing a fusion of the tobacco hsr203J gene promoter with a Phytophthora cryptogea gene exhibited heightened resistance to multiple pathogens, illustrating the versatility of this approach.
Resistance of Transgenic Crops To Abiotic Stresses
Abiotic stresses, which include drought, low temperatures, salinity, and alkalinity, negatively affect plant growth, leading to senescence, cell death, and reduced crop yields. These stresses often result in cellular water deficits or osmotic stress, necessitating specific plant responses to survive and thrive in such challenging environments.
- Cellular Responses to Water Deficit:
- To counteract osmotic stress, plants synthesize and accumulate low molecular weight compounds known as osmoprotectants.
- These osmoprotectants lower the osmotic potential within cells, thus helping to maintain turgor pressure.
- They consist of a diverse group of compounds, including:
- Inorganic ions
- Organic ions
- Soluble carbohydrates such as polyols (sugars and alcohols)
- Amino acids, notably proline
- Quaternary ammonium compounds, like glycine betaine
- Genetic Basis of Osmolyte Synthesis:
- Numerous plant genes encoding key enzymes involved in osmolyte biosynthesis have been successfully cloned.
- For instance, a strong correlation exists between proline accumulation and enhanced tolerance to drought and salinity stress.
- The enzyme γ-Pyrroline-5-carboxylate synthetase (P5CS) is pivotal in proline synthesis, with transgenic tobacco plants expressing this enzyme demonstrating a 10–18 fold increase in proline levels, correlating with enhanced growth under salt and drought conditions.
- Glycine Betaine Accumulation:
- The synthesis of glycine betaine is prevalent among angiosperms, significantly contributing to drought and salt tolerance.
- The biosynthesis involves a two-step oxidation of choline, catalyzed by choline monooxygenase (CMO) and betaine aldehyde dehydrogenase (BADH).
- Transgenic tobacco, Arabidopsis, and rice expressing BADH have exhibited increased salt resistance, underscoring the importance of glycine betaine in osmotic stress response.
- Mannitol and Fructan Biosynthesis:
- The introduction of the E. coli gene mtlD (mannitol-1-phosphate dehydrogenase) into tobacco resulted in transgenic plants that exhibited greater shoot height and longer roots when exposed to saline conditions, despite limitations for agricultural application.
- Similarly, tobacco engineered for fructan biosynthesis demonstrated improved growth rates under drought stress conditions, suggesting potential pathways for enhancing osmotic stress resistance.
- Dehydration Responsive Transcription Factors (DREB):
- Research has focused on identifying DREB genes that mediate the transcription of numerous genes in response to cold and water stress.
- DREB genes, which are activated under stress, encode transcription factors that bind to specific promoter elements (DRE) of stress-related genes, activating their expression.
- For example, overexpression of a DREB gene in Arabidopsis has shown marked increases in tolerance to freezing, water stress, and salinity.
- Heat Shock Proteins (HSPs) and Thermotolerance:
- High temperature stress tolerance has been enhanced in transgenic Arabidopsis through modulating the expression of HSPs via the Arabidopsis heat shock protein transcription factor (AtHSF).
- This research indicates that the constitutive expression of HSPs can play a critical role in maintaining cellular integrity under thermal stress.
- Oxidative Stress Tolerance Mechanisms:
- The production of reactive oxygen species (ROS) is a common outcome of drought and salt stresses, emphasizing the importance of a plant’s ability to scavenge these toxic molecules.
- Various enzymatic antioxidants, such as superoxide dismutase (SOD), catalase (CAT), and peroxidase, play significant roles in detoxifying ROS.
- SOD converts superoxide radicals into hydrogen peroxide and molecular oxygen, maintaining low superoxide concentrations.
- Catalase and peroxidase further break down hydrogen peroxide, while the ascorbate-glutathione cycle comprises enzymes like ascorbate peroxidase and glutathione reductase, working collectively for detoxification.
- Transgenic Approaches to Enhance Oxidative Stress Tolerance:
- Research has demonstrated that transgenic plants overexpressing mitochondrial MnSOD in chloroplasts achieve paraquat tolerance.
- The introduction of other SODs from different species into plants, such as tomato chloroplast Cu/ZnSOD and pea chloroplastic SOD, also leads to improved oxidative stress tolerance.
- Notably, transgenic tobacco plants exhibiting enhanced glutathione reductase activity show increased resistance to photooxidative stress.
- Management of Hydroxyl Radicals:
- The ability to scavenge hydroxyl radicals is crucial for protecting sensitive metabolic processes and structures within the plant.
- For instance, the development of transgenic rice expressing ferritin, which aids in iron storage, has resulted in enhanced tolerance to oxidative damage.
- Responses to Anoxia and Hypoxia:
- Oxygen deprivation, either complete (anoxia) or partial (hypoxia), can severely impair plant health, especially during flooding.
- Genetic engineering approaches have aimed to introduce genes that enhance intracellular oxygen levels.
- Expression of non-symbiotic hemoglobin genes from barley and bacteria in plants has been associated with faster germination and improved growth under anaerobic conditions, indicating a role in maintaining energy levels during oxygen deficiency.
- Cold Stress Responses:
- The introduction of genes for fatty acid desaturases into plants has shown promise in enhancing cold tolerance.
- For instance, the expression of a chloroplast ω-3 fatty acid desaturase gene in tobacco has led to improved chilling tolerance, reflecting the significant impact of membrane fluidity on cold stress responses.
Herbicide resistance of transgenic crops
The development of herbicide-resistant crops represents a significant advancement in modern agriculture, enabling more efficient weed control and enhancing crop productivity. The engineering of herbicide resistance has focused on single-gene modifications, which have proven to be effective in conferring resistance to various herbicides. Several strategies have been employed to achieve this resistance, each targeting specific biochemical pathways within the plant.
- Strategies for Engineering Herbicide Resistance:
- Overproduction of Herbicide-Sensitive Targets: This strategy involves increasing the levels of a specific biochemical target that herbicides typically inhibit. By enhancing the target enzyme’s concentration, the plants can withstand the herbicide’s effects.
- Structural Alteration of Biochemical Targets: Here, the focus is on modifying the structure of the target enzyme, resulting in reduced affinity for the herbicide. This means that the herbicide cannot effectively bind to its target, allowing the plant to survive.
- Detoxification of Herbicides: In this approach, plants are engineered to produce enzymes that can detoxify or degrade the herbicide before it reaches its biochemical target. This prevents the herbicide from exerting its harmful effects.
- Key Genes and Mechanisms:
- Resistance to glyphosate and sulfonylurea herbicides has been achieved through the introduction of genes coding for mutant versions of two key enzymes involved in amino acid biosynthesis:
- 5-Enolpyruvylshikimate-3-phosphate Synthase (EPSPS): Mutated forms of EPSPS confer resistance to glyphosate, a widely used herbicide.
- Acetolactate Synthase (ALS): Mutations in the ALS enzyme provide resistance to sulfonylurea herbicides.
- The gox gene from the Achromobacter bacterial strain codes for glyphosate oxidase, which detoxifies glyphosate.
- For glufosinate ammonium resistance, genes that encode phosphinothricin acetyltransferase (PAT) have been isolated from bacteria. PAT modifies phosphinothricin into a form that does not exert toxic effects on the plant.
- Resistance to glyphosate and sulfonylurea herbicides has been achieved through the introduction of genes coding for mutant versions of two key enzymes involved in amino acid biosynthesis:
- Transgenic Plant Development:
- Transgenic plants resistant to a variety of herbicides, including phosphinothricin, glyphosate, sulfonylurea, imidazolinones, bromoxynil, atrazine, 2,4-D, and sethoxydim, have been successfully generated across multiple crop species.
- Specific crops such as cotton, flax, canola, corn, and soybean have seen the commercial release of herbicide-resistant varieties. The prevalence of herbicide-resistant crops has significantly increased, covering approximately 63% of the total 114.3 million hectares of global transgenic crop area as of 2007. Notably, herbicide-tolerant soybean accounts for 51% of this area, followed by maize (6%), canola (5%), and cotton (1%) .
Transgenics for Quality
Transgenic technology has revolutionized agricultural practices, enabling the development of crops with improved storage, longer shelf life, and enhanced ornamental qualities. This advancement employs various genetic engineering techniques to modify plants, ensuring they meet consumer demands for quality and durability.
- Transgenics for Improved Storage:
- The Flavr Savr tomato, developed by Calgene in 1994, was the first commercially approved transgenic food product. This tomato features delayed ripening, allowing for longer shelf life and suitability for food processing.
- Two main approaches were employed to achieve improved storage in tomatoes:
- Antisense RNA Technology: This involves creating an antisense gene that is complementary to the sense (endogenous) gene. The antisense RNA produced pairs with the sense RNA, leading to:
- Unavailability of mRNA for translation, preventing protein production.
- Degradation of double-stranded RNA molecules by specific RNases.
- Potential methylation of the promoter and coding regions of the normal genes, silencing them.
- Calgene utilized this technology to inhibit the expression of the polygalacturonase (PG) gene, responsible for fruit softening. The transformation of tomato plants with an antisense PG gene resulted in reduced softening and extended shelf life—approximately two weeks longer on store shelves.
- 1-Aminocyclopropane-1-Carboxylic Acid (ACC) Deaminase: Ethylene, a phytohormone, plays a critical role in regulating fruit ripening. The conversion of S-adenosyl methionine (SAM) to ACC, and subsequently to ethylene, is facilitated by ACC synthase (ACS) and ACC oxidase (ACO). Genetic engineering approaches focused on expressing a gene encoding an enzyme capable of degrading ACC, thus reducing ethylene production and delaying ripening. Companies like Monsanto developed transgenic tomatoes exhibiting this trait through the overexpression of a SAM hydrolase gene.
- Antisense RNA Technology: This involves creating an antisense gene that is complementary to the sense (endogenous) gene. The antisense RNA produced pairs with the sense RNA, leading to:
- Longer Life Transgenic Flowers:
- The post-harvest life of flowers is often compromised by petal senescence, notably in carnations. Research led by Savin et al. (1996) produced transgenic carnations expressing antisense ACC oxidase, significantly delaying petal senescence and extending vase life by 200%. This genetically engineered carnation has received approval in Australia and the European Union.
- Transgenics for Flower Color and Shape:
- Flower color has been a primary focus for enhancement through biotechnology, particularly the development of blue flowers in species that do not naturally exhibit such pigmentation. Anthocyanins, primarily responsible for flower color, include delphinidin, which imparts blue hues.
- Researchers from Florigene identified and cloned a gene from Petunia hybrida encoding flavonoid 3′ 5′-hydroxylase (F3′5′H), crucial for delphinidin biosynthesis. The introduction of this gene into carnations resulted in the production of violet flowers, alongside new commercial varieties with modified colors, including Moonlite and Moonshadow.
- Modifications to flower color intensity have also been achieved through the introduction of chalcone synthase (chs) transgenes into various ornamental species, altering their color characteristics. Additionally, the expression of maize transcriptional factor ‘R’ in transgenic plants has elevated anthocyanin levels, affecting leaf color and potentially allowing for novel color patterns in ornamental species.
- Flower color has been a primary focus for enhancement through biotechnology, particularly the development of blue flowers in species that do not naturally exhibit such pigmentation. Anthocyanins, primarily responsible for flower color, include delphinidin, which imparts blue hues.
- Transgenics for Male Sterility:
- Male sterility can be induced in plants through nuclear and cytoplasmic mechanisms. Cytoplasmic male sterility (cms) typically arises from mitochondrial genome defects, leading to compromised pollen development while maintaining female fertility.
- Transgenic male sterility has been achieved in Brassica napus by introducing a ribonuclease gene under the control of an anther-specific promoter, resulting in selective cytotoxicity to tapetal cells and subsequent male sterility. This technology allows for the production of hybrid seeds in crops like canola, with restoration of male fertility through crossing with specific transgenic lines expressing a ribonuclease inhibitor.
- Male sterility can be induced in plants through nuclear and cytoplasmic mechanisms. Cytoplasmic male sterility (cms) typically arises from mitochondrial genome defects, leading to compromised pollen development while maintaining female fertility.
- Transgenics for Terminator Seed Technology:
- Terminator technology, or Genetic Use Restriction Technology (GURT), has garnered attention due to its implications for seed viability. This technology employs a lethal gene that ensures seeds produced in subsequent generations are infertile, compelling farmers to purchase new seeds each planting season.
- The technology is structured around three genetic components:
- Lethal Gene: Produces a toxic protein affecting seed germination, typically activated only in later stages of seed development through specific promoters like LEA.
- Recombinase Gene: Facilitates the excision of a blocking sequence between the lethal gene and its promoter, enabling the lethal gene’s expression at the appropriate developmental stage.
- Repressible Gene: Produces a repressor protein that can be inactivated by a specific chemical, allowing for the controlled expression of the recombinase gene during germination.
- The technology is structured around three genetic components:
- Terminator technology, or Genetic Use Restriction Technology (GURT), has garnered attention due to its implications for seed viability. This technology employs a lethal gene that ensures seeds produced in subsequent generations are infertile, compelling farmers to purchase new seeds each planting season.