What is Plant Metabolism?
- Plant metabolism encompasses a vast array of chemical reactions occurring within plant cells, all of which are meticulously organized and interconnected. Collectively, these reactions form the foundation of life in plants and are essential for growth, development, and response to environmental stimuli. Metabolism can be divided into two primary categories: catabolism and anabolism, each serving distinct yet interrelated functions.
- Catabolism refers to the breakdown of complex molecules into simpler units, typically accompanied by the release of energy. In plants, this process is crucial for converting stored energy in the form of carbohydrates, fats, and proteins into usable forms, primarily through cellular respiration. During catabolism, compounds like glucose are oxidized to produce adenosine triphosphate (ATP), the energy currency of cells, which powers various physiological processes.
- Conversely, anabolism encompasses the biosynthetic pathways that construct complex molecules from simpler precursors. This process is vital for building cellular structures, such as proteins, nucleic acids, and polysaccharides, which are essential for growth and reproduction. Anabolic reactions often require energy input, typically derived from ATP generated during catabolic processes. Although catabolic and anabolic pathways are distinct, they are intricately linked through shared intermediates, illustrating the dynamic nature of plant metabolism.
- A metabolic pathway, often visualized as a metabolic map, represents a sequence of enzymatic reactions leading to the formation of specific products, termed metabolites. Each step in these pathways is catalyzed by enzymes that facilitate the conversion of substrates into intermediates and ultimately into final products. These metabolites play crucial roles in various physiological functions, including energy production, synthesis of biomolecules, and regulation of metabolic processes.
What is Catabolism?
Catabolism is a fundamental metabolic process that plays a crucial role in living organisms, including plants and animals. The primary aim of catabolism is to extract energy from biomolecules, converting it into adenosine triphosphate (ATP), which serves as an energy currency for cellular activities. Additionally, catabolism generates essential precursors required for synthesizing complex molecules. The catabolic process can be divided into three distinct stages:
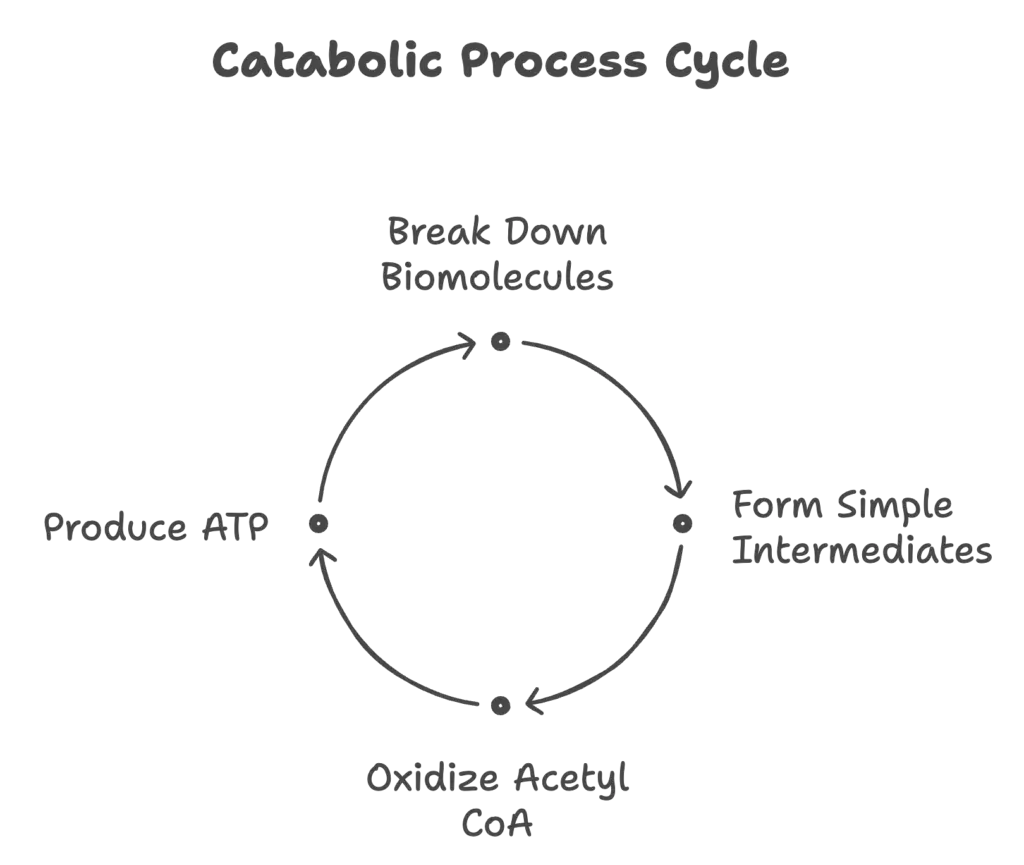
- Conversion of Complex Molecules into Building Blocks: In the initial stage, larger biomolecules are broken down into their constituent parts. Polysaccharides are hydrolyzed into monosaccharides, lipids are separated into free fatty acids and glycerol, and proteins are degraded into amino acids. This breakdown is essential for making the components available for further degradation and energy extraction.
- Formation of Simple Intermediates: The building blocks produced in the first stage undergo further degradation to yield simple intermediates, such as pyruvate and acetyl CoA. These intermediates do not conform to the traditional classifications of carbohydrates, lipids, or proteins. During this stage, a small quantity of energy is captured in the form of ATP, which is critical for fueling subsequent cellular activities.
- Final Oxidation of Acetyl CoA: The final stage involves the complete oxidation of acetyl CoA, resulting in the production of carbon dioxide (CO₂). This oxidation process liberates reducing equivalents in the form of NADH and FADH₂. These reduced coenzymes are subsequently oxidized in the electron transport chain, leading to the generation of a substantial amount of ATP. The Krebs cycle, also known as the citric acid cycle, serves as a pivotal metabolic pathway in the final oxidation of all energy-rich molecules, accepting carbon compounds derived from carbohydrates, lipids, or proteins.
What is Anabolism?
Anabolism is a vital component of metabolism that focuses on the synthesis of complex molecules from simpler precursors. This process is essential for growth, repair, and maintenance of cellular structures in living organisms. Anabolism relies on a limited number of starting materials, which include key intermediates such as pyruvate, acetyl CoA, and various components derived from the citric acid cycle. The following points elaborate on the mechanisms and significance of anabolic pathways:
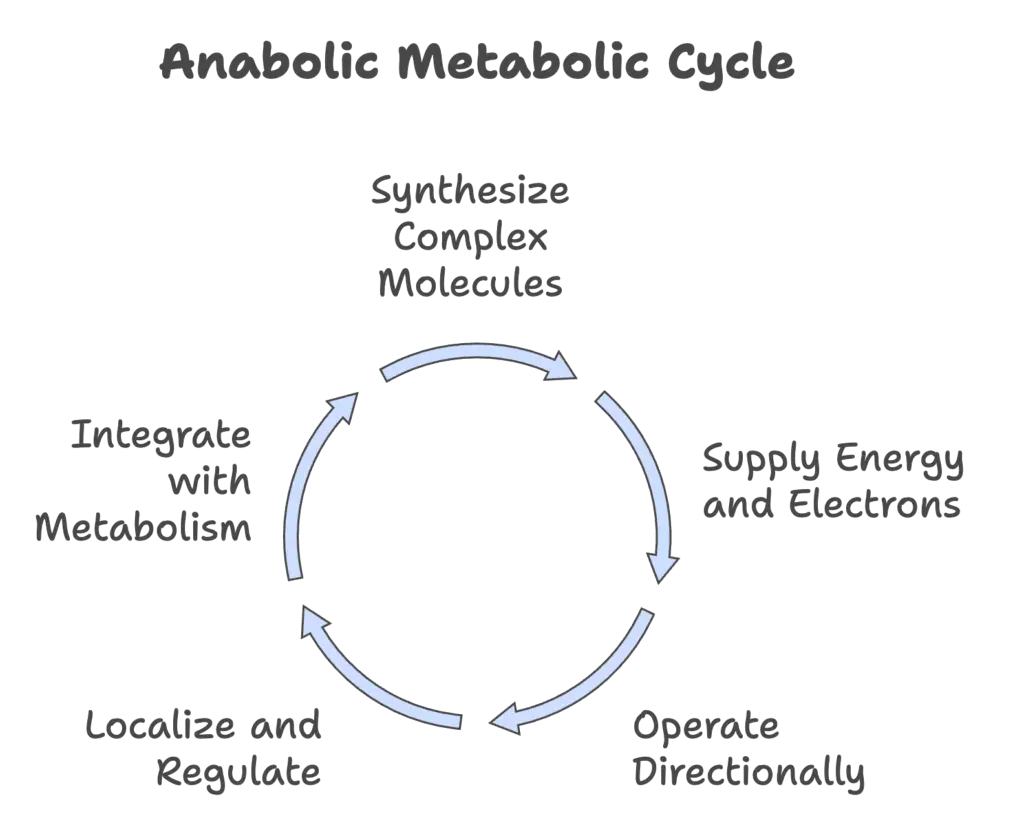
- Synthesis of Complex Molecules: Anabolic reactions are responsible for building a diverse array of macromolecules, including proteins, nucleic acids, lipids, and polysaccharides. These complex molecules are crucial for cellular function and structural integrity.
- Energy and Reducing Equivalents: Anabolism requires a supply of energy, typically in the form of adenosine triphosphate (ATP) or guanosine triphosphate (GTP). Additionally, reducing equivalents, specifically NADPH + H⁺, play a crucial role in these reactions by providing the necessary electrons for biosynthetic processes.
- Directional Pathways: Anabolic and catabolic pathways are distinct and operate independently, meaning that they are not reversible. This directional nature of metabolic pathways ensures that the processes of energy production (catabolism) and biosynthesis (anabolism) do not interfere with one another, thus maintaining cellular efficiency.
- Cellular Location and Regulation: Anabolic reactions are localized within specific cellular compartments, such as the mitochondria and microsomes, where the necessary enzymes and substrates are available. Furthermore, these pathways are regulated by different signaling mechanisms that respond to the metabolic needs of the cell.
- Intermediary and Energy Metabolism: The broader context of metabolism includes intermediary metabolism, which encompasses the full spectrum of anabolic and catabolic reactions that do not involve nucleic acids. Energy metabolism, on the other hand, specifically focuses on pathways that manage the storage and release of energy, highlighting the interconnectedness of anabolic processes with overall energy management in the cell.
Types of Metabolic Reactions
Metabolic reactions are essential biochemical processes that enable living organisms to convert nutrients into energy, synthesize necessary biomolecules, and maintain cellular functions. These reactions can be categorized into four primary types, each characterized by distinct mechanisms and roles in metabolism. Understanding these types provides insight into how organisms manage their biochemical pathways efficiently.
- Oxidation-Reduction Reactions (Redox Reactions): These reactions involve the transfer of electrons between molecules, leading to changes in their oxidation states. In biological systems, oxidation refers to the loss of electrons, while reduction denotes the gain of electrons. Oxidation-reduction reactions are critical for energy production, particularly in processes like cellular respiration, where glucose is oxidized to generate ATP. Enzymes such as dehydrogenases facilitate these reactions by transferring electrons to electron carriers like NAD⁺ or FAD.
- Group Transfer Reactions: This type of reaction involves the transfer of a specific functional group from one molecule to another. Group transfer reactions are fundamental in metabolic pathways, as they enable the modification of substrates to form new compounds. For instance, in phosphorylation reactions, a phosphate group is transferred from ATP to a substrate, converting it into a more reactive form. This is crucial for energy metabolism and the activation of various biomolecules.
- Rearrangement and Isomerization Reactions: These reactions involve the rearrangement of atoms within a molecule to form isomers, which are compounds with the same molecular formula but different structural configurations. Isomerization is vital for metabolic flexibility, as it allows the organism to adapt and utilize different substrates efficiently. Enzymes known as isomerases catalyze these reactions, facilitating transformations that are essential for various metabolic pathways.
- Make and Break of Carbon-Carbon Bonds: These reactions are critical for the synthesis and degradation of organic compounds. Making carbon-carbon bonds is essential for building larger, more complex molecules, while breaking these bonds is key for catabolism and energy release. Enzymes such as ligases catalyze the formation of these bonds, while hydrolases and lyases are involved in the cleavage of carbon-carbon bonds. This category of reactions underpins many fundamental metabolic processes, including the synthesis of fatty acids and the breakdown of glucose.
Methods Employed to Study Metabolism
Studying metabolism presents unique challenges due to the interdependent nature of metabolic reactions, which are integrated into specific pathways. However, the fundamental metabolic pathways are remarkably conserved across various organisms, facilitating research efforts. To elucidate these biochemical reactions and pathways, several experimental approaches are employed, broadly categorized into three primary methods:
- Use of Whole Organisms or Their Components:
- Whole Organisms: Techniques such as the glucose tolerance test (GTT) are commonly used to assess how an entire organism metabolizes glucose. This method provides insight into the functional status of metabolic pathways in response to glucose intake.
- Isolated Components: Researchers often study specific organs, tissue slices, whole cells, or subcellular organelles to investigate particular biochemical reactions. These isolated systems allow for more controlled experiments, which help elucidate the mechanisms underlying metabolic pathways.
- Utility of Metabolic Probes:
- Metabolic Inhibitors: These are compounds that selectively inhibit specific enzymes within metabolic pathways. By observing the effects of these inhibitors, scientists can determine the roles of particular enzymes and trace the flow of metabolites through various pathways.
- Mutations: Genetic mutations can be utilized to disrupt normal metabolic functions, providing insights into the consequences of altered biochemical pathways. Studying these mutations allows researchers to identify the significance of specific genes and proteins in metabolism.
- Application of Isotopes:
- Isotopes are used to trace the pathways of metabolic reactions. By incorporating stable or radioactive isotopes into substrates, researchers can track their incorporation into metabolites and monitor metabolic flux. This method provides a detailed understanding of how compounds are transformed within metabolic pathways.
The methods mentioned above can be employed either in vivo (within a living system) or in vitro (in a controlled laboratory environment, such as a test tube), with many studies utilizing a combination of both approaches.
Furthermore, metabolism is a continuous process involving thousands of reactions occurring simultaneously within cells. Biochemists often present metabolism in terms of discrete reactions and pathways for clarity and ease of understanding.
Additionally, understanding the integration of metabolism involves considering energy demands and supplies within organisms. Since energy requirements can vary significantly, the metabolic fuel consumed may either be oxidized to produce carbon dioxide (CO₂) and water (H₂O) or stored for future use. Adenosine triphosphate (ATP) serves as the energy currency of the cell, facilitating energy transfer and utilization.
For instance, humans possess a remarkable capacity for food consumption, often consuming up to 100 times their basal energy requirements. This propensity for overconsumption can lead to disorders such as obesity, particularly prevalent in affluent societies, emphasizing the need to balance energy intake and expenditure.
Integration of Major Metabolic Pathways of Energy Metabolism
The integration of major metabolic pathways is essential for understanding energy metabolism within living organisms. These interconnected pathways facilitate the conversion of various substrates into usable energy forms, primarily through the synthesis of adenosine triphosphate (ATP). The following points provide a detailed overview of the key metabolic pathways involved in energy metabolism:
- Glycolysis: This pathway represents the initial step in glucose metabolism, where glucose is broken down into pyruvate. In the process, glycolysis generates a net yield of 8 ATP molecules. Under anaerobic conditions, pyruvate can be converted to lactate, while in aerobic conditions, it is further transformed into acetyl CoA, a crucial intermediate in metabolic processes.
- Fatty Acid Oxidation: Fatty acids are degraded through a series of enzymatic reactions, releasing two-carbon fragments known as acetyl CoA. This process not only produces acetyl CoA for entry into the citric acid cycle but also traps energy in the forms of reduced coenzymes, specifically NADH and FADH₂.
- Degradation of Amino Acids: Amino acids, particularly those consumed in excess of requirements for protein synthesis, are metabolized to meet energy demands. Glucogenic amino acids can be converted into glucose through pathways that involve pyruvate or intermediates of the citric acid cycle. Conversely, ketogenic amino acids can be directly transformed into acetyl CoA, thereby integrating into energy metabolism.
- Citric Acid Cycle (Krebs Cycle): Acetyl CoA serves as a central metabolite derived from various fuel sources, including carbohydrates, lipids, and amino acids. Upon entering the citric acid cycle, acetyl CoA undergoes oxidation, resulting in the release of carbon dioxide (CO₂). This cycle is crucial as it functions as the final common metabolic pathway for the oxidation of all foodstuffs, trapping most of the resulting energy in the form of NADH and FADH₂.
- Oxidative Phosphorylation: The NADH and FADH₂ generated from different metabolic pathways are subsequently oxidized in the electron transport chain (ETC). This process is coupled with oxidative phosphorylation, where the energy released during electron transfer is used to produce ATP, thus highlighting the critical link between metabolic pathways and energy production.
- Hexose Monophosphate Shunt (PPP): This pathway is primarily focused on generating NADPH and ribose sugars. NADPH plays a pivotal role in the biosynthesis of various biomolecules, including fatty acids, while ribose is a crucial component of nucleotides and nucleic acids, such as DNA and RNA (note that DNA specifically contains deoxyribose).
- Gluconeogenesis: This metabolic pathway synthesizes glucose from non-carbohydrate precursors, including pyruvate, glycerol, and amino acids. Gluconeogenesis is vital for maintaining blood glucose levels, especially during fasting or low-carbohydrate intake.
- Glycogen Metabolism: Glycogen, the storage form of glucose, is primarily found in the liver and muscle tissues. It is subject to degradation (glycogenolysis) and synthesis (glycogenesis) through independent pathways. Glycogen serves as a readily available fuel reserve to meet energy needs between meals, illustrating the importance of carbohydrate storage in energy homeostasis.
Regulation of Metabolic Pathways
The regulation of metabolic pathways is essential for maintaining homeostasis within organisms. This regulation ensures that biochemical processes respond appropriately to various physiological needs and environmental conditions. Four primary mechanisms govern the regulation of metabolic pathways:
- Availability of Substrates: The concentration of substrates directly influences metabolic pathways. When substrates are abundant, metabolic processes can proceed efficiently, facilitating the production of necessary products. Conversely, a lack of substrates can slow down or halt metabolic reactions. Therefore, substrate availability is a fundamental determinant of metabolic flux and overall pathway activity.
- Covalent Modification of Enzymes: Enzymes can undergo covalent modifications that alter their activity. These modifications typically involve the addition or removal of chemical groups, such as phosphate groups through phosphorylation. Such alterations can significantly affect enzyme function, enabling rapid responses to changes in cellular conditions. Consequently, this form of regulation allows for precise control over metabolic reactions based on cellular demands.
- Allosteric Regulation: Allosteric regulation involves the binding of effector molecules to specific sites on enzymes, distinct from the active site. This binding can induce conformational changes that either enhance or inhibit enzyme activity. Allosteric enzymes often exhibit sigmoidal kinetics, indicating that their activity is sensitive to changes in substrate concentration. Through allosteric regulation, metabolic pathways can be finely tuned to respond to fluctuations in the cellular environment, ensuring that metabolic outputs align with physiological needs.
- Regulation of Enzyme Synthesis: The synthesis of enzymes themselves can be regulated at the genetic level. This regulation can occur through transcriptional control, wherein the production of mRNA for specific enzymes is upregulated or downregulated in response to varying metabolic demands. By modulating enzyme levels, the cell can effectively control the capacity of metabolic pathways over time, ensuring an adequate supply of enzymes for metabolic processes based on the organism’s state and needs.
In addition to these mechanisms, regulatory enzymes play a crucial role in the control of metabolic pathways. Regulatory enzymes are characterized by their ability to respond to specific biomolecules, thereby modulating pathway activity. They often exist at relatively high concentrations and possess low maximum reaction velocities (Vmax), which allows their activity to be easily adjusted with changes in substrate concentration.
These enzymes are integral to pathways where the products are required in variable amounts over time, such as in hormone production. The ability to increase or decrease the activity of regulatory enzymes based on the availability of substrates or effector molecules allows the organism to adapt to changing metabolic demands efficiently.
Role of Regulatory Enzymes
Allosteric Enzymes
Allosteric enzymes play a critical role in the regulation of biochemical pathways through their unique structural and functional characteristics. These enzymes possess two distinct binding sites: one for the substrate and another for effectors, which are small molecules that modulate enzyme activity. Understanding allosteric enzymes is essential for grasping how metabolic processes are fine-tuned within the cell.
- Binding Sites: Allosteric enzymes feature a catalytic site where the substrate binds, and an allosteric site where effectors can bind. The binding of an effector to the allosteric site induces conformational changes in the enzyme, impacting its catalytic activity without participating directly in the chemical reaction.
- Role of Effectors: Effectors can be categorized based on their influence on enzyme activity:
- Positive Effectors (Allosteric Activators): When an effector enhances enzyme activity upon binding, it is termed a positive effector. For example, adenosine diphosphate (ADP) acts as an allosteric activator for phosphofructokinase, promoting the enzyme’s catalytic efficiency.
- Negative Effectors (Allosteric Inhibitors): Conversely, if binding of the effector decreases enzyme activity, it is classified as a negative effector. For instance, adenosine triphosphate (ATP) and citrate are negative effectors for phosphofructokinase, while glucose-6-phosphate serves as an allosteric inhibitor for hexokinase.
- Structural Characteristics: Allosteric enzymes are generally larger than conventional enzymes due to their multi-subunit composition. This structure allows for the presence of multiple active sites and regulatory binding sites, which work in concert to modulate enzyme function. Therefore, allosteric enzymes exhibit cooperative kinetics, wherein the conformational changes in one subunit affect the neighboring subunits, thereby amplifying the response of the entire enzyme complex.
- Classification Based on Modulation:
- Homotropic Allosteric Enzymes: In these enzymes, both the substrate and effector play roles in modulating enzyme activity. The binding of the substrate can influence the activity of other substrate binding sites, thereby affecting overall catalytic efficiency.
- Heterotropic Allosteric Enzymes: In contrast, these enzymes rely solely on the effector for modulation. Here, the effector can be a metabolite that influences enzyme activity independent of substrate binding.
- Mechanism of Allosteric Regulation: The binding of allosteric effectors leads to conformational changes within the enzyme. In the case of positive effectors, the catalytic site becomes more conducive to substrate binding, enhancing the enzyme’s activity. Conversely, negative effectors induce conformational changes that render the catalytic site less effective for substrate binding, thus reducing enzymatic activity.
- Kinetics of Allosteric Enzymes: A distinctive characteristic of allosteric enzymes is their kinetic behavior:
- When plotting reaction velocity against substrate concentration, allosteric enzymes typically display a sigmoidal curve rather than a hyperbolic one, indicating cooperative binding.
- Unlike typical Michaelis-Menten kinetics, allosteric enzymes do not adhere to the Michaelis-Menten equation. Instead, the Lineweaver-Burk plot of these enzymes reveals a concave upward shape, further demonstrating their unique kinetic properties.
Covalent Modulation
Covalent modulation refers to the alteration of enzyme activity through the formation of covalent bonds, leading to significant changes in biochemical pathways. This process is essential for regulating metabolic activities within the cell, and it encompasses several types of modifications, including phosphorylation, methylation, hydroxylation, and adenylation. Among these, phosphorylation is the most prominent and widely studied form of covalent modification, primarily influencing enzyme activity in various metabolic processes.
- Types of Covalent Modifications:
- Phosphorylation: This involves the addition of a phosphate group (PO₄) to an enzyme, typically at the hydroxyl group of amino acids such as serine, threonine, or tyrosine. Phosphorylation is catalyzed by specific enzymes known as protein kinases. This modification can either activate or inhibit enzyme function, depending on the context of the specific enzyme and its role in metabolism.
- Methylation: The addition of a methyl group (CH₃) to specific amino acids can alter enzyme activity, although this is less common compared to phosphorylation.
- Hydroxylation: This modification involves the addition of a hydroxyl group (-OH) to an enzyme, influencing its activity and interactions within metabolic pathways.
- Adenylation: The addition of adenylic acid can also affect enzyme function, although the specifics of this modification are less frequently discussed in the context of metabolic regulation.
- Phosphorylation Mechanism:
- Activation and Deactivation: The phosphorylation of enzymes can result in the activation of some enzymes while simultaneously inhibiting others. For example, in many cases, the phosphorylated form of an enzyme serves as the active variant. Protein kinases facilitate the phosphorylation process, thus enabling the activation of enzymes involved in degradative reactions.
- Key Enzymes Activated by Phosphorylation:
- Citrate Lyase: This enzyme catalyzes the breakdown of citrate, thus playing a crucial role in the citric acid cycle.
- Glycogen Phosphorylase: This enzyme breaks down glycogen into glucose, providing a vital energy source during metabolic demand.
- Lipase: This enzyme hydrolyzes triglycerides into glycerol and fatty acids, facilitating fat metabolism.
- Enzymes Inactivated by Phosphorylation:
- In contrast, certain enzymes involved in biosynthetic reactions are inhibited when phosphorylated. Examples include:
- HMG CoA Reductase: This enzyme is crucial for cholesterol biosynthesis and is typically inhibited by phosphorylation, thus regulating lipid metabolism.
- Acetyl CoA Carboxylase: This enzyme plays a pivotal role in fatty acid biosynthesis and is also inactivated through phosphorylation.
- Glycogen Synthase: Responsible for catalyzing the biosynthesis of glycogen, this enzyme is inhibited when phosphorylated, thereby regulating glucose storage.
- In contrast, certain enzymes involved in biosynthetic reactions are inhibited when phosphorylated. Examples include:
- Role of Dephosphorylation:
- Dephosphorylation, the reverse process of phosphorylation, involves the removal of phosphate groups from enzymes, primarily at serine, threonine, or tyrosine residues. This process is facilitated by phosphatases. The functional state of the enzyme—whether it is active or inactive—depends on the specific biochemical context, and different enzymes may exhibit varying responses to these modifications.
Isoenzymes
Isoenzymes, also known as isozymes, are different forms of enzymes that catalyze the same chemical reaction but vary in structure and properties. These enzymes are typically multimeric proteins, meaning they consist of two or more polypeptide chains, which can be produced from different genes. The existence of isoenzymes allows for a fine-tuned regulation of metabolic processes, providing flexibility and specificity based on tissue type and physiological conditions.
- Characteristics of Isoenzymes:
- Structural Variability: Isoenzymes often differ in their polypeptide chain composition, which can lead to variations in kinetic properties, regulatory mechanisms, and response to inhibitors or activators. Despite these differences, isoenzymes maintain a high degree of similarity in their amino acid sequences, typically sharing about 75% of their sequences.
- Tissue Specificity: Isoenzymes exhibit tissue specificity, meaning they can be found in different locations within a single cell or in various tissues throughout the organism. This characteristic allows for the specialization of enzyme function in different metabolic contexts, accommodating the unique needs of specific tissues.
- Example of Isoenzymes: A prominent example of isoenzymes is lactate dehydrogenase (LDH), which plays a crucial role in anaerobic metabolism by catalyzing the conversion of pyruvate to lactate. LDH exists in several isozyme forms, each with distinct polypeptide compositions and tissue distributions:
- LDH2 (HHHM): Predominantly found in the heart and erythrocytes (red blood cells), this isozyme reflects the high demand for energy and anaerobic metabolism in these tissues.
- LDH3 (HHMM): Located primarily in the brain and kidneys, this isozyme is adapted to the specific metabolic requirements of these organs.
- LDH4 (HMMM): Found in skeletal muscle and the liver, this isozyme supports the unique metabolic functions in muscle contraction and energy storage.
- LDH5 (MMMM): This isozyme is also present in skeletal muscle and the liver, complementing the metabolic pathways involved in energy production and substrate utilization.
- Functional Implications: The presence of multiple isoenzymes allows for a versatile response to metabolic demands across different tissues. For instance, during exercise, LDH in skeletal muscle can facilitate lactate production to meet increased energy needs, while LDH in the heart may help manage lactate levels during intense activity. This specificity ensures that metabolic pathways are efficiently regulated according to the physiological state of the organism.
- Kochhar SL, Gujral SK. Concepts of Metabolism. In: Plant Physiology: Theory and Applications. Cambridge University Press; 2020:153-167.
- https://tmv.ac.in/ematerial/botany/db/SEM%20VI%20BOTANY%20C13T%20Plant%20Metabolism.pdf
- https://www.uvm.edu/~cparis/PBIO006/Chap4_Metabolism.pdf
- https://www.ncbi.nlm.nih.gov/pmc/articles/PMC157444/pdf/1080949.pdf