Mutations play a significant role in crop improvement by introducing genetic variability, which can be harnessed to develop new and improved crop varieties. A mutation is defined as a sudden and heritable change in an organism’s characteristics. This definition implies that the change must be inheritable, though it does not specify the genetic mechanisms underlying these changes.
Mutations can arise from various genetic alterations. Point mutations involve changes in the base sequences of genes due to base pair transitions, transversions, deletions, duplications, or inversions. These gene mutations are often detectable through sophisticated genetic analysis techniques, especially in microorganisms. Therefore, point mutations in crops are studied with advanced genetic tools to understand their impacts on plant traits.
In addition to gene mutations, chromosomal mutations occur when there are alterations in chromosome structure or number. These can include translocations, inversions, large deletions, or duplications. Gross chromosomal changes are typically visible under a microscope, whereas smaller changes might be harder to detect and are often categorized as gene mutations. In higher organisms like plants, chromosomal changes are sometimes overlooked due to less refined genetic analysis techniques compared to microorganisms. Consequently, what are often referred to as gene mutations in plants might also encompass minor chromosomal alterations.
Bud mutations, or somatic mutations, are another important category. These occur in the buds or other somatic tissues used for propagation, such as in clonal crops. Such mutations can influence the genetic makeup of the propagated plants and, consequently, their traits. Therefore, the term ‘mutation’ encompasses both gene and chromosomal changes, as well as variations occurring in somatic tissues, depending on the context and the detection methods employed.
In summary, mutations, whether they involve changes in gene sequences or chromosomal structures, contribute to genetic diversity in crops. This genetic variability is crucial for breeding programs aiming to enhance crop performance and resilience.
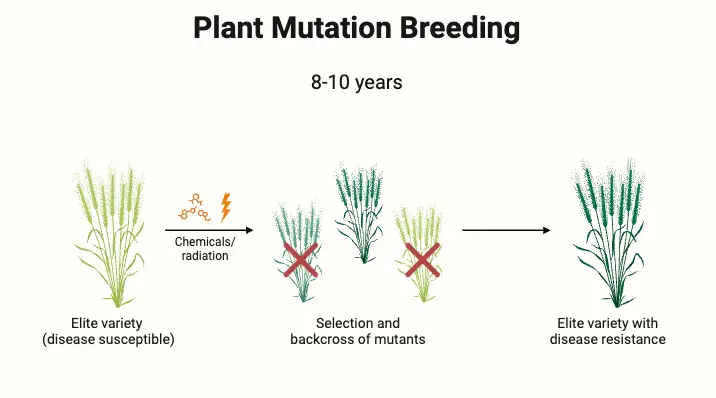
Classification of mutations
Mutations can be classified based on their origin and the magnitude of their phenotypic effects. Understanding these classifications is essential for both basic genetic research and practical applications in crop improvement.
Classification Based on Origin:
- Spontaneous Mutations:
- Definition: These occur naturally within populations without external intervention. The frequency of spontaneous mutations is typically low, around 10^-6 per gene per generation, though this rate can vary significantly between different genes.
- Example: In maize, the R-locus mutates at a frequency of 4.92 × 10^-4 (1 in 20,000 plants), while the Su locus mutates at a much lower rate of 2.4 × 10^-6 (1 in 2.5 million plants). The Wx locus, by contrast, is considered highly stable.
- Influencing Factors: The mutation rate can be influenced by factors such as the genetic background of the organism, the presence of mutator genes, and environmental conditions.
- Induced Mutations:
- Definition: These are artificially introduced through exposure to physical or chemical agents. Induced mutations generally do not create new alleles but rather produce variants that are already known from spontaneous mutations.
- Characteristics: Although induced mutations occur at a higher frequency than spontaneous ones, they are comparable in terms of the variability and effects they produce. This higher frequency makes them practical for experimental use and breeding programs.
Classification Based on Magnitude of Phenotypic Effects:
- Macro Mutations:
- Definition: These mutations result in significant phenotypic changes that are easily recognizable at the individual plant level.
- Characteristics: Macro mutations often affect a few genes (oligogenic mutations) and can be observed in the M2 generation. For instance, the transition from ancon breed sheep to pod maize and cob maize are examples of macro mutations.
- Application: Due to their prominent effects, these mutations are readily identifiable and useful in breeding programs.
- Micro Mutations:
- Definition: These mutations produce minor phenotypic changes that are not visible in individual plants but become apparent when observed across a group of plants.
- Characteristics: Micro mutations involve many genes (polygenic mutations) and require selection in later generations (such as M3 or beyond) to be effectively recognized and utilized.
- Application: Due to their subtle effects, micro mutations necessitate a larger sample size and longer observation periods to identify their impact.
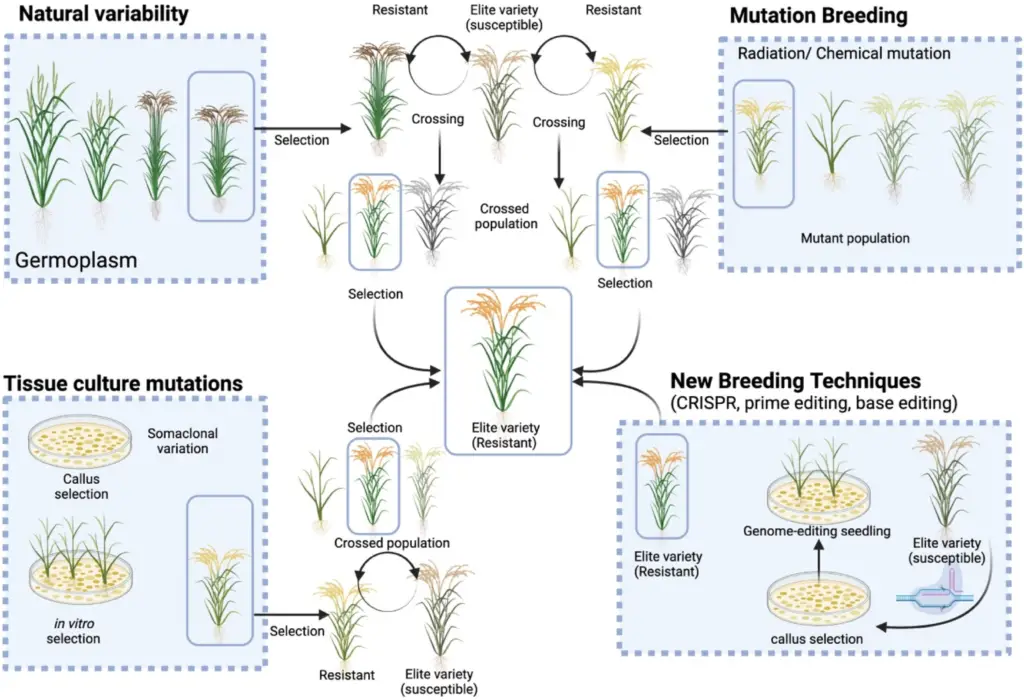
Effects of mutation
Mutations can significantly impact organisms in various ways, often influencing their viability and overall fitness. Understanding these effects is crucial for both basic genetic research and applied fields such as crop improvement. Mutations are generally classified into four categories based on their impact on viability: lethal, sublethal, subvital, and vital.
- Lethal Mutations:
- Definition: Lethal mutations result in the death of all individuals that possess them when present in the appropriate genotype.
- Characteristics: These mutations are often dominant, making them unobservable in a heterozygous state because the organism cannot survive. Recessive lethal mutations can be studied, but only in homozygous individuals. For example, mutations affecting chlorophyll production, leading to albino plants, can be lethal.
- Implications: Since lethal mutations cause the death of the carriers, they do not contribute to genetic diversity in a population and are generally excluded from practical applications in crop improvement.
- Sublethal Mutations:
- Definition: Sublethal mutations decrease the viability of the carriers but do not kill all individuals with the mutation.
- Characteristics: These mutations result in the death of more than 50% of the individuals carrying them, but a portion of the population can survive. This category represents a significant reduction in fitness but not complete lethality.
- Implications: Sublethal mutations may have limited practical value in breeding programs due to their detrimental effects on survival rates. However, they are important for understanding mutation impacts on organism viability.
- Subvital Mutations:
- Definition: Subvital mutations also reduce the viability of individuals, though they do not lead to widespread death.
- Characteristics: These mutations cause a reduction in fitness that is less severe than that caused by sublethal mutations. The majority of individuals with subvital mutations survive, but they may have reduced performance or other issues.
- Implications: While subvital mutations can impact overall fitness, they are less critical compared to lethal and sublethal mutations. They can be of academic interest but are less likely to be utilized in crop improvement due to their negative effects.
- Vital Mutations:
- Definition: Vital mutations do not adversely affect the viability of the carriers.
- Characteristics: These mutations occur with lower frequency compared to lethal, sublethal, and subvital mutations. They do not reduce the survival of individuals and can potentially contribute to beneficial traits.
- Implications: Vital mutations are valuable for crop improvement because they provide opportunities for enhancing desirable traits without compromising survival. These mutations are sought after in breeding programs to develop improved crop varieties.
Mutagens
Mutagens are agents that induce mutations by causing alterations in the genetic material of organisms. These agents can be categorized into two main types: physical mutagens and chemical mutagens. Each type functions through different mechanisms to induce genetic changes.
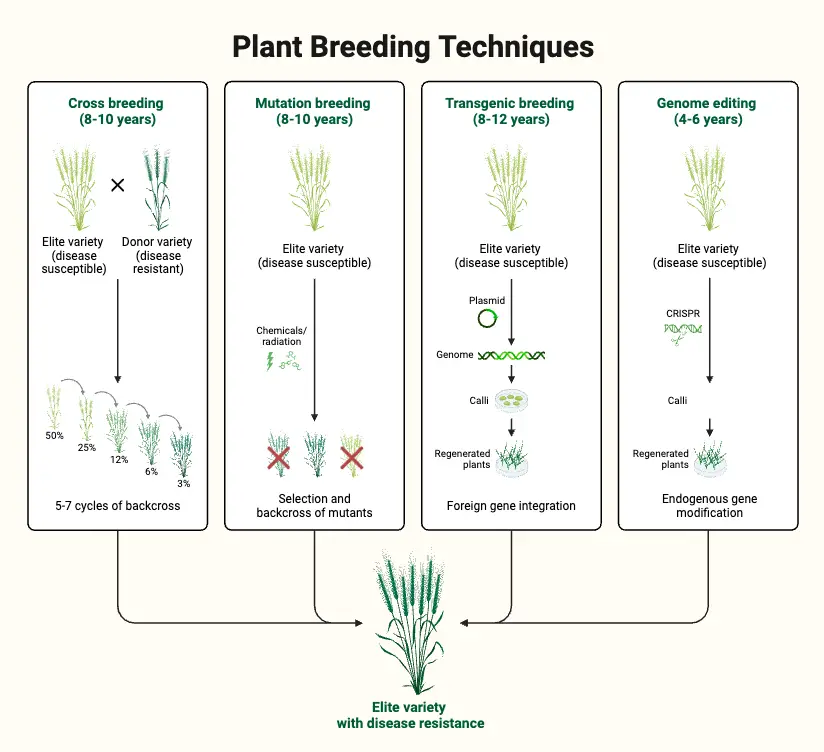
A. Physical Mutagens:
- Ionizing Radiations:
- Particulate Radiations:
- Alpha Rays (α-rays): Heavily charged particles that can cause significant damage to DNA when they interact with biological tissues.
- Beta Rays (β-rays): Less massive than alpha rays and penetrate tissues to a greater extent, causing DNA damage.
- Fast Neutrons: High-energy neutrons that can induce complex DNA damage by displacing protons in the atom’s nucleus.
- Thermal Neutrons: Lower energy neutrons that cause mutations primarily through their interactions with atomic nuclei.
- Nonparticulate Radiations:
- X-rays: Electromagnetic radiation that penetrates tissues and induces mutations through interactions with DNA molecules.
- Gamma Rays (γ-rays): High-energy electromagnetic radiation that can penetrate deep into tissues and induce mutations by causing breaks in DNA strands.
- Particulate Radiations:
- Nonionizing Radiations:
- Ultraviolet (UV) Radiation: Causes mutations by forming pyrimidine dimers in DNA, which can lead to errors during DNA replication if not repaired.
B. Chemical Mutagens:
- Alkylating Agents:
- Sulphur Mustards: Chemicals like mustard gas that add alkyl groups to DNA bases, leading to incorrect base pairing.
- Nitrogen Mustards: Similar to sulphur mustards, these also cause alkylation of DNA.
- Epoxides: Reactive molecules that can form covalent bonds with DNA, resulting in mutations.
- Ethylene-Imines: Chemicals such as ethylene imine that modify DNA structure and function.
- Sulphates and Sulphonates: Compounds like ethylmethane sulphonate (EMS) and methylmethanesulphonate (MMS) that introduce alkyl groups into DNA.
- Diazoalkanes: Chemicals that induce mutations through alkylation.
- Nitroso Compounds: Examples include N’-methyl-N-nitro-N-nitroso-guanidine (MNNG), which can alter DNA bases.
- Acridine Dyes:
- Examples: Acriflavine, proflavine, acridine orange, acridine yellow, and ethidium bromide. These dyes intercalate into DNA, causing frameshift mutations during DNA replication.
- Base Analogues:
- Examples: 5-bromouracil and 5-chlorouracil. These compounds resemble normal DNA bases and can be incorporated into DNA, leading to mispairing during replication.
- Other Chemical Mutagens:
- Nitrous Acid: Causes deamination of DNA bases, leading to mispairing.
- Hydroxylamine: Adds hydroxyl groups to DNA bases, affecting base pairing.
- Sodium Azide: Causes mutations by introducing nitrogen into DNA, which can disrupt normal base pairing.
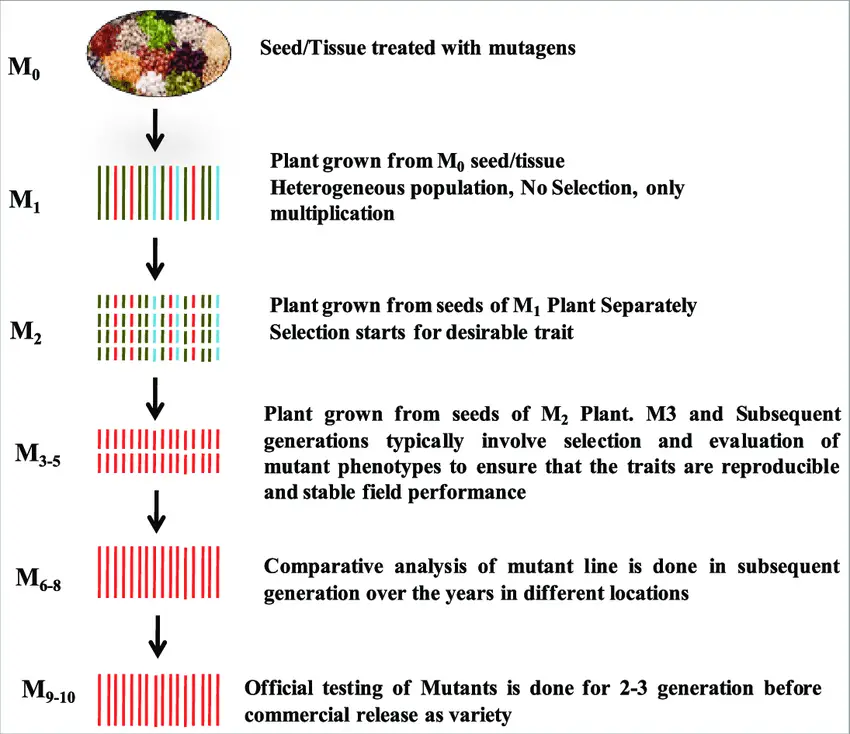
Procedure for Irradiation in Mutation Breeding
The irradiation of plant material is a critical step in mutation breeding, and the procedure varies depending on the type of plant material and the goals of the breeding program. The following outlines the common procedures and considerations for effective irradiation:
- Selection of Plant Material
- Seeds
- Pre-treatment: Seeds are often soaked prior to irradiation to increase the likelihood of inducing mutations. Soaked seeds typically exhibit a higher frequency of mutations compared to air-dried seeds.
- Rationale: This pre-treatment helps in enhancing the sensitivity of seeds to irradiation, leading to better outcomes in mutation frequency.
- Seedlings
- Timing: Seedlings can be irradiated at various stages of their life cycle. However, it is common practice to select seedlings that are neither too young nor too old. This ensures ease of handling and effective transportation from the nursery.
- Considerations: Proper selection of the developmental stage of seedlings is crucial for achieving optimal results.
- Flowers
- Meiotic Sensitivity: Flowers are often chosen for irradiation because meiotic cells within the flowers are more sensitive to radiation compared to mitotic cells. This approach targets the developing gametes, which are critical for mutation induction.
- Objective: By irradiating flowers, breeders aim to induce mutations in the gametes, which can then be passed on to subsequent generations.
- Cuttings
- Clonal Propagation: For fruit trees propagated by cloning, cuttings are exposed to irradiation. This method ensures that the desired traits can be transferred through cloned plant material.
- Purpose: Irradiation of cuttings allows for the introduction of mutations into asexually propagated varieties, enabling the development of new traits in cloned plants.
- Seeds
- Determination of Mutagen Dose
- Optimal Dosage: Selecting an appropriate dose of the mutagen is crucial. The goal is to use a dose that maximizes the frequency of induced mutations while minimizing lethality.
- Optimum Dose: An optimal dose is typically close to the LD50, which is the dose required to kill 50% of the treated individuals. This balance ensures effective mutation induction with manageable levels of plant mortality.
- Dosage Adjustment: Careful calibration of the mutagen dose is necessary to achieve the desired mutation frequency and to avoid excessive damage to the plant material.
- Optimal Dosage: Selecting an appropriate dose of the mutagen is crucial. The goal is to use a dose that maximizes the frequency of induced mutations while minimizing lethality.
Procedure for Mutation Breeding
Mutation breeding involves the induction of mutations in biological materials to enhance or alter specific traits. This process includes treating plant materials with mutagens and then selecting for desirable mutations. Below is a detailed, step-by-step guide to the procedure for mutation breeding:
- Define Objectives
- A mutation breeding program must have well-defined objectives. This involves specifying the traits to improve and the goals of the breeding effort. Since the ratio of beneficial to harmful mutations is relatively low, a clear objective helps in efficiently directing resources and efforts. Identifying desirable mutations among many is challenging, so a precise focus is crucial.
- Select the Variety for Mutagen Treatment
- The choice of variety for mutagenesis typically involves selecting a superior variety for polygenic trait improvement. However, in some cases, such as the search for dwarfing genes in cereals, mutants may be isolated from varieties that are not the best performing. The selected variety should ideally be adapted to the desired outcome of the breeding program.
- Choose the Plant Part for Treatment
- The part of the plant to be treated depends on the propagation method of the crop and the type of mutagen used. For sexually propagated crops, seeds are most commonly used, though pollen grains can be used less frequently due to practical difficulties. For asexually propagated crops, such as those propagated through buds or cuttings, these parts are treated with mutagens. Radiations can be applied to all plant parts, including whole plants, while chemical mutagens are usually applied to seeds.
- Determine the Mutagen Dose
- The dose of the mutagen is critical for effective mutation induction. An optimal dose balances between inducing a high frequency of mutations and minimizing plant mortality. The dose often corresponds to the LD50 (lethal dose for 50% of the population). Preliminary experiments are usually conducted to determine the appropriate dose, with adjustments made based on the mutagen type and crop species.
- Apply the Mutagen Treatment
- For irradiation, plant parts are exposed to the mutagenic dose, and then planted to grow the M1 generation. Chemical mutagens often require pre-soaking of seeds, followed by exposure and washing to remove residual chemicals before planting. This ensures that the treated material can effectively produce the desired mutations in subsequent generations.
- Handle the Mutagen-Treated Population
- The M1 generation often includes chimeras—plants with mixed genotypes due to the mutagen treatment. Chimeras can be categorized as periclinal or sectorial, based on the layers affected. Only inner chimeras are transmitted in sexually reproducing species, while both inner and outer chimeras can be utilized in clonal crops. Stable mutant phenotypes require multiple generations and selection to isolate and propagate desirable traits.
- Selection and Evaluation
- For oligogenic traits (controlled by a few genes), selection typically involves screening the M2 generation for distinct mutations. In contrast, for polygenic traits (controlled by multiple genes), selection begins in later generations (M3 and beyond). Detailed evaluation and testing are required to identify and propagate mutations that provide improvements over the original variety.
- Conduct Yield Trials
- Once desirable mutants are identified, they undergo preliminary and replicated yield trials to assess their performance compared to control varieties. Promising mutants are tested in various locations to ensure their effectiveness and stability across different environments.
- Release New Varieties
- Based on the results of yield trials and evaluations, outstanding mutant lines may be released as new varieties. This involves a final round of testing and approval to ensure that the new variety meets the desired criteria and performs well under diverse conditions.
- Precautions
- To prevent outcrossing, especially in self-pollinated species, it is essential to isolate the mutation-treated plants. Additionally, careful management is required to avoid mechanical mixtures, which can result in confusion between true mutants and existing genotypes.
Mutation Breeding for Oligogenic Traits
Mutation breeding for oligogenic traits focuses on modifying traits controlled by a small number of genes. The process involves several distinct stages, each crucial for identifying and developing beneficial mutations. Here is a step-by-step guide to this procedure:
- M1 Generation: Initiation
- Treatment and Planting: Several hundred seeds are treated with a mutagen to induce mutations. These seeds are then spaced and planted to ensure that approximately 500 fertile M1 plants are produced. This number provides a sufficient sample size for effective mutation screening.
- Isolation Measures: To prevent outcrossing, the M1 plants must be isolated. This can be achieved by planting the M1 population in a controlled environment, bagging the inflorescences, or isolating the entire plants. This isolation is critical to avoid contamination from other varieties.
- Harvesting Seeds: Seeds are harvested from each M1 plant separately, usually around 20 to 25 seeds per plant. These seeds will be used to generate the M2 progeny rows.
- M2 Generation: Screening for Mutants
- Progeny Rows: Approximately 2,000 M2 progeny rows are planted. Each row represents seeds harvested from individual M1 plants.
- Observation and Selection: Careful and regular observations are made to identify distinct mutations. Due to the large number of rows and the individual nature of mutations, only those showing clear, new traits are noted. Most mutations will be non-beneficial, with only 1-3% of rows expected to contain advantageous traits.
- Harvesting and Propagation: Plants suspected of containing useful mutations are harvested and used to produce M3 progeny rows for further evaluation.
- M3 Generation: Further Evaluation
- Progeny Rows: M3 progeny rows are grown from individual selected plants. This stage involves eliminating poor or inferior mutant rows.
- Bulk Harvesting: Homogeneous M3 rows with consistent mutations may be bulked together. These rows are then harvested in bulk for preliminary yield trials in the M4 generation.
- M4 Generation: Preliminary Yield Trials
- Trial Conduct: A preliminary yield trial is conducted using a suitable control variety for comparison. Promising mutant lines are selected based on their performance in these trials.
- Selection for Multilocation Trials: The selected lines are advanced to replicated multilocation trials to test their performance across different environments.
- M5-M7 Generations: Advanced Yield Trials
- Multilocation Trials: The promising mutant lines undergo replicated multilocation yield trials. These trials assess the performance and stability of the mutations in diverse conditions.
- Release of New Varieties: Outstanding mutant lines that perform well in trials may be released as new varieties. Conversely, low-yielding lines are retained for use in hybridization programs to potentially improve other breeding lines.
Mutation Breeding for Polygenic Traits
Mutation breeding for polygenic traits aims to enhance characteristics governed by multiple genes. The approach involves several stages designed to identify and develop beneficial mutations affecting complex traits. Here is a detailed description of the process:
- M1 and M2 Generations: Initial Selection
- Planting and Growth: M1 and M2 generations are grown following similar protocols as for oligogenic traits. In M2, plants that appear vigorous, fertile, and normal, without exhibiting the mutant phenotype, are selected.
- Seed Harvesting: Seeds from these selected M2 plants are harvested separately to produce M3 progeny rows. This step ensures that the plants used for further evaluation are not showing unwanted mutant traits.
- M3 Generation: Evaluation of Phenotypic Deviations
- Progeny Rows: M3 progeny rows are cultivated from the seeds of selected M2 plants. The focus here is on identifying any small deviations in phenotype from the parent variety.
- Selection Process: Careful and regular observations are made to detect mutants with altered quantitative traits, such as partial or horizontal disease resistance. Given the polygenic nature of these traits, many M3 rows will display segregation.
- Discarding Inferior Rows: Rows that do not exhibit desirable traits or show poor performance are discarded. This step helps in focusing resources on more promising lines.
- Identification of Mutants: Intensive evaluation of a large number of M3 progeny rows helps in identifying useful mutants. These mutants often occur at relatively high frequencies, making the process cost-effective.
- M4 Generation: Preliminary Yield Testing
- Bulk Planting: Seeds from homogeneous M3 rows are bulked and planted for preliminary yield trials. These trials include a suitable check variety to assess performance.
- Selection for Multilocation Trials: Superior progenies from these preliminary tests are selected for further replicated multilocation trials. Individual plant progenies from M3 are also observed critically to ensure their potential for improvement.
- M5-M8 Generations: Advanced Trials and Release
- Yield Trials: In M5 through M8 generations, the focus is on conducting replicated multilocation yield trials. This testing determines the performance and stability of the selected mutants across various environments.
- Final Selection and Release: Outstanding progenies that show consistent improvement in polygenic traits may be released as new varieties. Any lines with suboptimal performance are retained for hybridization programs or further testing.
Mechanism of Action of Radiations
Radiation affects biological molecules through both direct and indirect mechanisms. Understanding these mechanisms is crucial for comprehending how radiation induces genetic mutations and other biological changes. The primary effects of radiation can be categorized into two types: direct and indirect.
- Direct Effect
- Energy Transfer: In the direct effect, radiation transfers its energy directly to biological molecules. This energy transfer results in ionization or excitation of the molecules. For instance, when high-energy radiation such as gamma rays or X-rays interacts with a molecule, it can eject electrons from the molecule, creating ions.
- Ion-Pair Formation: The ejected electron is captured by another atom, forming a negatively charged ion, while the original molecule becomes a positively charged ion. This results in the formation of an ion-pair. For example, when radiation ionizes water, it generates pairs of ions, such as H₂O⁺ and e⁻.
- Indirect Effect
- Role of Free Radicals: The indirect effect of radiation is mediated by free radicals, which are highly reactive molecules with unpaired electrons. These radicals are primarily formed from the ionization of water molecules, a process known as radiolysis of water. The ionization of water produces hydroxyl radicals (OH•) and hydrogen atoms (H•).
- Chemical Reactions: Free radicals have a very short life span, typically around 10⁻¹⁰ seconds. Despite their brevity, they are highly reactive and can interact with other cellular molecules, including DNA. These interactions can lead to the formation of hydrogen peroxide (H₂O₂) and organic peroxides, which contribute significantly to the biological effects of radiation.
- Radiation-Induced Molecular Changes
- DNA Damage: The biological effects of radiation are often observed as changes in DNA structure. These include:
- Base Loss: Radiation can cause the loss of nitrogenous bases from DNA.
- Hydrogen Bond Breakage: Disruption of hydrogen bonds in DNA can affect its double-helix structure.
- Strand Breakage: Both single and double-strand breaks in DNA are common outcomes of radiation exposure. Double-strand breaks are particularly severe as they can lead to chromosomal aberrations.
- Cross-Linking: Radiation can induce cross-linking of DNA strands, which complicates DNA repair processes and can lead to mutations.
- DNA Damage: The biological effects of radiation are often observed as changes in DNA structure. These include:
- Protection and Repair
- Protein Coating: DNA located in chromatin is generally more protected than naked DNA due to its protein coating. This protein coating can shield the DNA from some of the direct effects of radiation.
- Free Radical Removal: Biological systems have mechanisms to mitigate radiation damage. Free radicals are neutralized through reactions such as:
- H• + OH• → H₂O
- H• + H• → H₂
- OH• + OH• → H₂O₂
- Oxygen Effect: The presence of oxygen increases the formation of reactive oxygen species (ROS), such as hydrogen peroxide (H₂O₂), which can further damage DNA. This phenomenon is known as the oxygen enhancement ratio, where oxygen enhances the effects of radiation on cells.
- Mutation Induction
- Gene Mutation: Changes in the base sequence of DNA due to radiation-induced damage can lead to gene mutations. These mutations can result from base loss, base alterations, or incorrect base pairing due to intrastrand dimerization.
Mechanism of Action of Chemical Mutagens
Chemical mutagens influence DNA through various mechanisms, which can broadly be categorized into mutagenic and inactivating changes. The actions of different chemical mutagens vary significantly, but the following summary outlines the general mechanisms by which they induce genetic mutations.
- Types of Mutagenic Changes
- Mutagenic Changes: These alterations do not prevent DNA replication but instead modify the structure of nucleotides. They result in changes in base pairing and hydrogen bonding properties, which can lead to mutations during DNA replication. These changes are generally categorized as:
- Substitution: This involves replacing one nucleotide with another, which can be further classified into:
- Transition: A substitution where a purine is replaced by another purine (A ↔ G) or a pyrimidine is replaced by another pyrimidine (C ↔ T).
- Transversion: A substitution where a purine is replaced by a pyrimidine or vice versa (A ↔ C or T, G ↔ C or T).
- Deletion: The removal of one or more nucleotides from the DNA sequence.
- Insertion: The addition of one or more nucleotides into the DNA sequence.
- Substitution: This involves replacing one nucleotide with another, which can be further classified into:
- Consequences:
- Frame-Shift Mutations: Deletions and insertions can disrupt the reading frame of the codons in a gene, leading to significant changes in the amino acid sequence downstream of the mutation site.
- Mutagenic Changes: These alterations do not prevent DNA replication but instead modify the structure of nucleotides. They result in changes in base pairing and hydrogen bonding properties, which can lead to mutations during DNA replication. These changes are generally categorized as:
- Inactivating Alterations
- Inactivating Changes: These mutations impede DNA replication and can cause chromosome breaks or mutations. They include:
- Base Removal: The loss of a nucleotide base can result in incomplete DNA sequences.
- Dimer Formation: The formation of pyrimidine dimers (e.g., thymine dimers) can create kinks in the DNA strand.
- Cross-Linking: Chemical agents may cross-link DNA strands, preventing proper separation during replication and transcription.
- Strand Breaks: Single or double-strand breaks in the DNA backbone disrupt the integrity of the genetic material.
- Inactivating Changes: These mutations impede DNA replication and can cause chromosome breaks or mutations. They include:
- Mechanism of Action of Alkylating Agents
- Alkylating Agents: These mutagens are known for their ability to form covalent bonds with DNA, leading to various types of DNA damage. They can be classified based on the number of reactive groups:
- Monofunctional Agents: React with a single site on DNA.
- Bifunctional Agents: Have two reactive groups that can form cross-links between different DNA strands.
- Polyfunctional Agents: Possess multiple reactive groups, leading to extensive cross-linking and damage.
- Specific Actions:
- Reaction with DNA Backbone: Alkylating agents can react with the phosphate-sugar backbone of DNA, leading to the formation of semistable triesters. This reaction can cause DNA strand breaks.
- Reaction with Ring Nitrogens: Particularly in guanine, alkylation at the N7 position can lead to the loss of the base (depurination), potentially resulting in backbone breaks.
- Transition Mutations: Alkylation of guanine may cause errors in base pairing, leading to transitions from Gpairs to Apairs.
- Alkylating Agents: These mutagens are known for their ability to form covalent bonds with DNA, leading to various types of DNA damage. They can be classified based on the number of reactive groups:
- Repair Mechanisms
- Cellular Repair: Many of the inactivating alterations caused by chemical mutagens are repaired by cellular enzymatic mechanisms. These repair systems include:
- Base Excision Repair: Removes and replaces damaged bases.
- Nucleotide Excision Repair: Removes and replaces damaged DNA segments.
- Homologous Recombination and Non-Homologous End Joining: Repair double-strand breaks.
- Cellular Repair: Many of the inactivating alterations caused by chemical mutagens are repaired by cellular enzymatic mechanisms. These repair systems include:
Applications of Mutation Breeding
Mutation breeding is a technique employed to enhance various plant traits through induced genetic variations. It is applicable in improving both oligo-genic and polygenic characteristics, and it serves several key functions in crop development:
- Induction of Desirable Mutant Alleles
- Objective: Mutation breeding can create or enhance mutant alleles that are not present in the existing germplasm or are inaccessible due to geographical or political constraints.
- Function: By inducing mutations, breeders can generate new genetic variations that may not be available through conventional germplasm collection. This method supplements rather than replaces the need for diverse germplasm sources.
- Limitation: While mutation breeding provides additional options, it cannot entirely eliminate the necessity for germplasm collections. It is a valuable tool for expanding genetic diversity and addressing gaps in available genetic resources.
- Improvement of Specific Characteristics in High-Yielding Varieties
- Objective: In well-adapted, high-yielding varieties, mutation breeding can enhance specific traits without altering the overall genetic composition of the variety.
- Application in Clonal Crops: For clonal crops, where individuals are genetically identical, mutation breeding is crucial for modifying specific traits. This approach is especially important for improving characteristics in clonal crops without changing their genetic makeup.
- Application in Self-Pollinated Species: In self-pollinated species, mutation breeding can improve particular traits of otherwise superior varieties. However, this method may not always be more efficient than standard backcrossing, especially when desirable mutations are associated with unwanted side effects like chromosomal aberrations or sterility.
- Enhancing Genetic Variability in Hybrid Crops
- Objective: Mutagenesis can be applied to F1 hybrids from intervarietal crosses to increase genetic variability and facilitate the recombination of linked genes.
- Function: Treatment of F1 hybrids with mutagens can induce mutations that enhance variability within the population. This increased variability can lead to a broader range of phenotypic traits, aiding in the selection of desirable characteristics.
- Evidence and Usage: While there is evidence that mutagenesis can increase variability and recombination, this application is not yet widely adopted in mutation breeding practices.
- Production of Chromosomal Translocations
- Objective: Irradiation of interspecific hybrids can produce translocations by transferring chromosome segments carrying desirable genes from alien species to cultivated crops.
- Function: This technique allows for the introduction of new genetic material into cultivated species, potentially enhancing traits such as disease resistance or yield. However, this application primarily involves irradiation rather than traditional mutation breeding techniques.
Limitations of Mutation Breeding
Mutation breeding, despite its advantages, faces several limitations that impact its efficacy and applicability. These constraints are crucial for understanding the scope and challenges of this technique in crop improvement:
- Resource-Intensive Process
- Large Populations Required: Mutation breeding necessitates the growth and thorough examination of large populations to identify and select desirable mutations. This process is both time-consuming and labor-intensive.
- High Resource Demands: The need to screen extensive populations for mutations requires substantial resources, including time, labor, and financial investment. Efficient screening techniques are necessary to manage these requirements effectively.
- Selection Challenges
- Need for Efficient Techniques: To identify desirable mutations, rapid and cost-effective selection methods are essential. Mutation breeding is more effective for traits where quick screening is possible, such as disease resistance.
- Complexity in Quality Traits: For traits requiring detailed and extensive testing, such as quality characteristics, mutation breeding is less efficient. The complexity of evaluating these traits can hinder the effectiveness of mutation breeding.
- Association of Unwanted Side Effects
- Undesirable Mutations: Desirable mutations often come with undesirable side effects, such as chromosomal aberrations or other genetic defects. These side effects can negatively impact the overall performance and quality of the mutant line.
- Need for Backcrossing: To eliminate these unwanted traits, mutant lines frequently need to be backcrossed to the original parent variety. This process adds to the time and labor required for successful mutation breeding.
- Difficulty with Recessive Mutations
- Challenges in Detection: Recessive mutations are often difficult to detect, particularly in clonal crops where all individuals are genetically identical, and in polyploid species with multiple sets of chromosomes.
- Increased Population Sizes: In polyploid species, the detection of recessive mutations requires the cultivation of larger populations and the application of higher doses of mutagens. This further escalates the resource requirements for mutation breeding.
- Limited Applicability in Certain Species
- Preference for Diploid Species: Mutation breeding has been most commonly applied to diploid species that reproduce sexually, especially self-pollinated varieties. Its application to other species, such as polyploids, is less straightforward and requires additional adjustments.
Advantages of Mutation Breeding
Mutation breeding offers several distinct advantages in crop improvement and genetic research. The following points outline the key benefits of employing mutation breeding techniques:
- Creation of Inexhaustible Variation
- Broad Genetic Variation: Mutation breeding generates a wide range of genetic variations. By introducing mutations, it is possible to create numerous new phenotypes, which may exhibit desirable traits not present in the original population.
- Enhanced Selection Opportunities: This genetic diversity allows breeders to select from a broader pool of variants, increasing the likelihood of identifying individuals with improved characteristics such as disease resistance, yield, or quality.
- Application When Conventional Methods Are Exhausted
- Alternative Approach: Mutation breeding serves as a valuable alternative when traditional breeding methods have reached their limits. In cases where conventional techniques cannot produce the desired improvements, induced mutations provide an avenue for further enhancement.
- Overcoming Genetic Barriers: For crops with limited genetic variability or where conventional breeding has been ineffective, mutation breeding can introduce new traits and overcome genetic constraints that hinder progress.
Achievements of Mutation Breeding
Mutation breeding has led to significant advancements in agricultural productivity and crop improvement across various plant species. This technique has successfully produced over 337 mutant varieties globally, with substantial contributions to the improvement of cereals, oilseeds, pulses, millets, vegetables, and fruit trees. Notably, wheat, barley, and rice account for approximately 50% of these mutant varieties. Below are some key achievements and examples illustrating the impact of mutation breeding:
- Development of New Varieties
- Primax White Mustard: The first variety developed through mutation breeding, released in 1950, followed by Regina II Summer Rape in 1953. These varieties showed modest improvements in yield and content compared to their parent varieties.
- NP 836 Wheat: Developed from the awnless variety NP 799, this variety demonstrated the ability of mutation breeding to alter specific traits without changing the overall genetic makeup of the variety.
- Jagannath Rice: A gamma-ray induced semidwarf mutant from the tall cultivar T 141. Jagannath rice exhibits enhanced lodging resistance, higher yield, and increased responsiveness to fertilizer compared to its parent.
- Enhanced Crop Traits
- CRM 13-324 Rice: Created through mutation breeding, this variety features reduced plant height and days to maturity, coupled with increased yield compared to its parent.
- Prabhavati Rice: An induced mutant from Ambemohar Local, showing improvements in yield, lodging resistance, and responsiveness to fertilizer and nitrogen. Prabhavati produces 35-40 quintals per hectare, outperforming the 25-30 quintals per hectare yield of the parent variety.
- High-Yielding Varieties
- Jute Variety JRO 3690: Developed by crossing two low-yielding mutants of Corchorus olitorius, this variety demonstrates the potential for mutation breeding to produce high-yielding progeny from initially low-yielding lines.
- Sugarcane Variety Co 8152: A gamma-ray induced mutant from Co 527, this variety offers a 40% higher cane yield than its parent. However, it also presents a challenge with faster drying of lower leaves, which has affected its adoption despite its higher yield.
- Diverse Mutant Varieties
- Cotton Varieties MCU 7, MCU 10, and DB-3-12: MCU 7 and MCU 10 were developed through induced mutations, with MCU 7 being early maturing and suitable for rice fallow areas, while MCU 10 performs better under rainfed conditions. DB-3-12, a spontaneous mutant, matures 20 days earlier than its parent variety.
- Mutagenesis Techniques and Proportions
- Physical vs. Chemical Mutagens: By 1982, approximately 94% of mutant varieties were developed using physical mutagens, 5% through chemical mutagens, and the remaining 1% through a combination of both. The higher prevalence of physical mutagens in asexually propagated crops reflects their extensive application and availability.
Role of mutations in crop improvement
Mutations play a pivotal role in crop improvement by introducing genetic variations that can be harnessed to develop superior crop varieties. The following outlines the various ways in which mutations contribute to the advancement of crop genetics and agriculture:
- Introduction of New Traits
- Novel Characteristics: Mutations can lead to the emergence of new traits that are not present in the original plant varieties. These traits may include improved disease resistance, enhanced yield, better nutritional quality, or altered growth habits.
- Examples: The development of disease-resistant varieties, such as those resistant to specific pathogens or pests, is often achieved through the introduction of mutations.
- Enhancement of Existing Traits
- Trait Improvement: Mutations can enhance existing traits by increasing their effectiveness or modifying them in beneficial ways. For example, mutations can improve the quality of fruits, increase the size of seeds, or boost the stress tolerance of plants.
- Examples: The creation of high-yielding varieties through mutations can lead to crops that produce more grain per plant, thereby increasing overall productivity.
- Development of Varieties with Specific Adaptations
- Environmental Adaptation: Mutations enable the development of crop varieties that are better suited to specific environmental conditions, such as drought tolerance, heat resistance, or soil salinity tolerance.
- Examples: Crops developed through mutation breeding that can thrive in arid or saline conditions help in expanding agriculture into less favorable regions.
- Acceleration of Breeding Programs
- Faster Selection: By inducing mutations, breeders can create a wide range of genetic variations in a shorter period compared to traditional breeding methods. This accelerates the selection process for desirable traits.
- Examples: Mutation breeding can quickly generate a diverse pool of genetic variants, from which superior lines can be selected and further developed.
- Overcoming Limitations of Conventional Breeding
- Genetic Diversity: Mutation breeding introduces new genetic diversity that may not be achievable through conventional cross-breeding alone. This is particularly useful when genetic resources are limited.
- Examples: In crops with limited genetic variability, mutations provide an additional source of genetic material that can be used to develop new varieties with unique attributes.
- Increased Resistance to Biotic and Abiotic Stress
- Stress Tolerance: Mutations can lead to the development of crop varieties with increased resistance to biotic stresses (e.g., pests and diseases) and abiotic stresses (e.g., drought, salinity).
- Examples: Varieties with enhanced resistance to fungal diseases or improved drought tolerance can significantly reduce crop losses and improve food security.
- Improvement in Quality Attributes
- Nutritional Value and Processing Quality: Mutations can enhance the nutritional value of crops or improve their processing qualities. This includes increasing vitamin content, improving taste, or altering texture.
- Examples: Mutant varieties with higher vitamin content or improved processing qualities for food products contribute to better human health and more efficient food production.
- http://courseware.cutm.ac.in/wp-content/uploads/2020/05/Download-Notes-27.pdf
- https://agriindiatoday.in/July%202021-28-32.pdf
- https://openknowledge.fao.org/server/api/core/bitstreams/a25f529d-592b-4368-9965-6c77696f00f1/content
- https://www.iaea.org/sites/default/files/34405682533.pdf