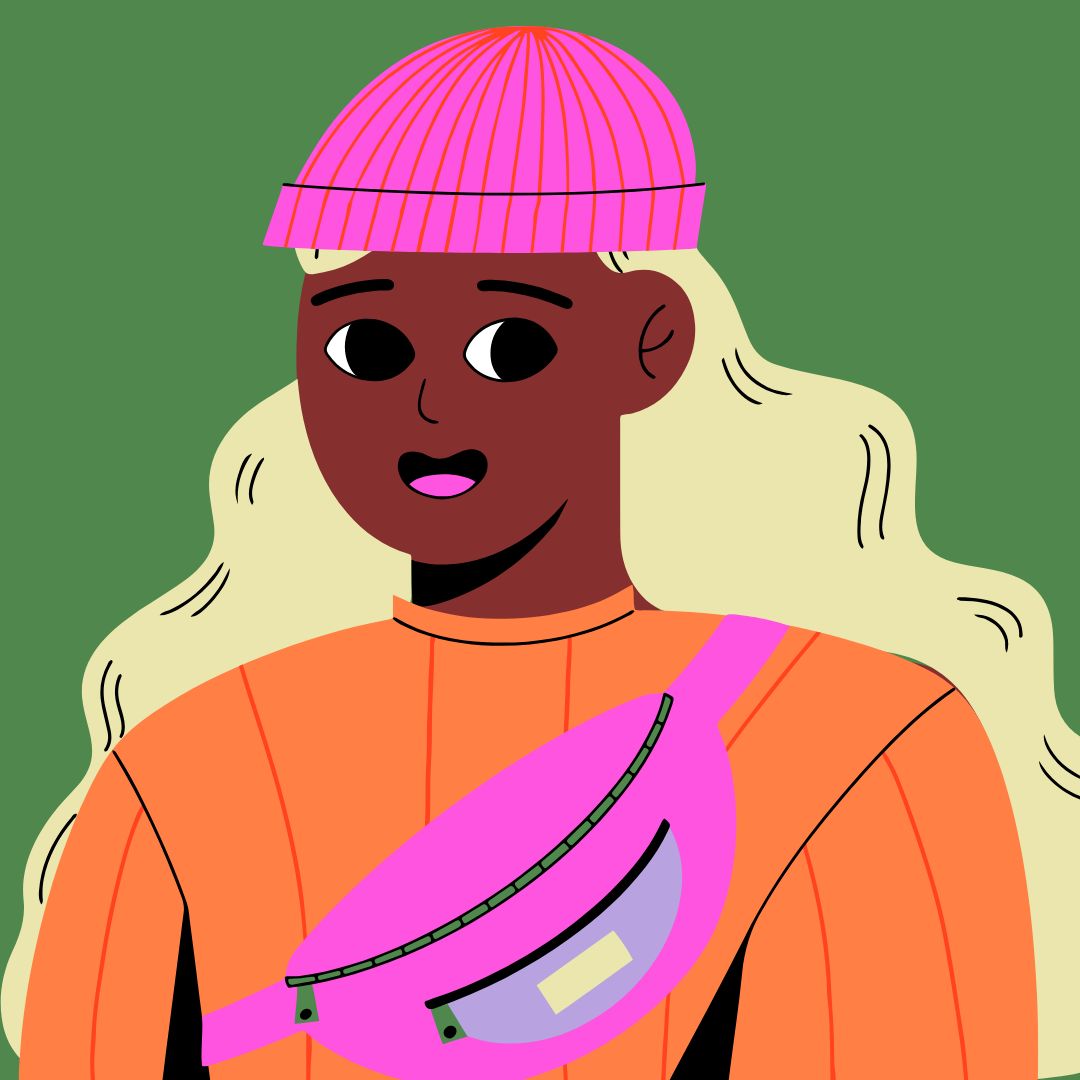
Sourav Pan
Transcript
What are autotrophs?
Autotrophs are organisms that can produce their own food from simple inorganic substances.
The word autotroph comes from the Greek: ‘auto’ meaning self, and ‘troph’ meaning nourishing.
The key characteristic of autotrophs is their ability to create complex organic compounds without consuming other organisms.
Let’s look at some examples of autotrophs.
Plants are the most familiar autotrophs. They use sunlight, water, and carbon dioxide to make their own food through photosynthesis.
Algae, including seaweeds and phytoplankton, are aquatic autotrophs that also use photosynthesis.
Some bacteria are autotrophs too. While many use photosynthesis, others can use chemical energy sources instead of sunlight.
All autotrophs share the amazing ability to transform simple inorganic substances into the complex organic compounds needed for life.
Let’s understand autotrophs by comparing them to chefs in a kitchen.
Autotrophs are like the master chefs of the natural world.
Just as a chef starts with basic ingredients like flour, eggs, and sugar…
…autotrophs begin with simple molecules like carbon dioxide, water, and capture energy from sunlight.
The chef transforms these raw ingredients into a complex dish such as a cake.
Similarly, autotrophs transform these simple molecules into complex organic compounds like glucose and starch.
Both processes involve the transformation of simple ingredients into something more complex and useful.
There are several key similarities between chefs and autotrophs. Both transform simple inputs into complex outputs. Both require energy to drive their processes. And importantly, both are self-sufficient creators, able to produce what they need without relying on others.
This self-sufficiency is what makes autotrophs unique in nature’s food web.
Autotrophs are organisms that can produce their own food from inorganic substances.
They are classified into two main categories based on their energy source.
Photoautotrophs use light energy from the sun to create organic compounds.
Examples include plants, algae, and some bacteria like cyanobacteria.
Chemoautotrophs, on the other hand, obtain energy from oxidizing inorganic chemicals in their environment.
Examples include certain bacteria and archaea, often found in extreme environments like deep-sea hydrothermal vents.
The key difference between these two types of autotrophs is their energy source: light energy versus chemical energy.
Both types are essential to Earth’s ecosystems, serving as the base of food chains and contributing to nutrient cycling.
Photoautotrophs are organisms that harness sunlight as their primary energy source.
These organisms capture light energy and convert it into chemical energy to produce their own food.
The most common examples of photoautotrophs include plants, algae, and cyanobacteria.
Photoautotrophs contain special molecules called photosynthetic pigments that capture light energy. The most important of these is chlorophyll.
Chlorophyll comes in several forms, with chlorophyll a and b being the most common in plants. Other pigments like carotenoids capture different wavelengths of light.
Photoautotrophs thrive in well-lit environments where they can access sunlight for photosynthesis.
On land, photoautotrophs like plants dominate forests, grasslands, and gardens where they receive abundant direct sunlight.
In aquatic environments, photoautotrophs such as algae and cyanobacteria are found primarily in the upper layers of water bodies where light can penetrate. The abundance decreases with depth as light diminishes.
Photoautotrophs are the foundation of most ecosystems on Earth, producing over ninety-nine percent of the planet’s organic matter through photosynthesis.
Chemoautotrophs are remarkable organisms that produce their own food without using sunlight.
Unlike photoautotrophs, they derive energy from inorganic chemical reactions to create organic compounds.
They harness energy by oxidizing inorganic compounds like hydrogen sulfide or ferrous iron, converting this chemical energy into food.
There are several types of chemoautotrophs found in nature.
Sulfur bacteria obtain energy by oxidizing hydrogen sulfide, a process that often produces elemental sulfur as a byproduct.
Iron bacteria convert ferrous iron to ferric iron, which creates the rusty deposits often seen in iron-rich water sources.
Some archaea, like methanogens, convert carbon dioxide and hydrogen into methane, thriving in oxygen-free environments.
Chemoautotrophs thrive in environments where sunlight is scarce or absent, but chemical energy is abundant.
Deep-sea hydrothermal vents are one of the most remarkable habitats, where superheated water rich in minerals creates an oasis of life in the dark ocean depths.
Hot springs contain diverse communities of chemoautotrophs that thrive in the mineral-rich, high-temperature environments.
Sulfur caves harbor specialized bacteria that metabolize the hydrogen sulfide gas present in these light-deprived environments.
These remarkable organisms have developed unique adaptations that allow them to thrive in some of Earth’s most extreme environments.
Photosynthesis is the fundamental process used by photoautotrophs to create their own food.
This remarkable process harnesses light energy, typically from the sun, to power a chemical reaction.
The basic reactants are carbon dioxide from the air and water absorbed through the roots.
When light energy from the sun interacts with chlorophyll in the plant’s leaves, it initiates a complex series of chemical reactions.
Through these reactions, the plant transforms these simple compounds into glucose, a type of sugar, and releases oxygen as a byproduct.
The complete photosynthesis equation can be written as: six molecules of carbon dioxide plus six molecules of water, plus light energy, yields one molecule of glucose and six molecules of oxygen.
This process effectively converts light energy from the sun into chemical energy stored in the bonds of glucose molecules.
The plant uses simple inorganic compounds – carbon dioxide and water – to create complex organic molecules like glucose.
The oxygen released during photosynthesis is essential for most life on Earth, including humans who require it for respiration.
The light-dependent reactions are the first phase of photosynthesis where plants capture energy from sunlight.
These reactions take place in the thylakoid membranes of the chloroplast.
The thylakoid membranes contain chlorophyll molecules, which are pigments that absorb light energy.
When sunlight strikes the chlorophyll molecules, they absorb specific wavelengths of light.
When chlorophyll absorbs light energy, its electrons become energized and move to a higher energy level.
The energized electrons are passed to an electron transport chain, which consists of several protein complexes embedded in the thylakoid membrane.
These electrons move through the chain, losing energy at each step. This energy is used to pump hydrogen ions across the membrane.
The hydrogen ion gradient creates potential energy, which is harnessed by an enzyme called ATP synthase.
As hydrogen ions flow through ATP synthase, their energy is used to combine ADP and phosphate to produce ATP, the energy currency of the cell.
In addition to ATP, the light-dependent reactions also produce NADPH, which carries high-energy electrons and will be used in the next phase of photosynthesis.
To replace the electrons that leave the chlorophyll, photosystem II splits water molecules.
This process, called photolysis, breaks water into protons, electrons, and oxygen. The electrons replace those lost from chlorophyll, while oxygen is released as a byproduct.
To summarize, the light-dependent reactions capture light energy using chlorophyll and convert it into chemical energy in the form of ATP and NADPH.
Water molecules are split to replace the electrons lost from chlorophyll, releasing oxygen as a byproduct. The ATP and NADPH produced will power the next stage of photosynthesis, the light-independent reactions or Calvin cycle.
The light-independent reactions, also known as the Calvin Cycle, represent the second major phase of photosynthesis.
Unlike the light-dependent reactions, these processes don’t directly require light energy, though they do use the ATP and NADPH produced during the light-dependent phase.
The Calvin Cycle occurs in the stroma, the fluid-filled space inside the chloroplast surrounding the thylakoid membranes.
The Calvin Cycle consists of three main stages: carbon fixation, reduction, and regeneration of the CO₂ acceptor molecule.
In the first stage, carbon fixation, the enzyme RuBisCO incorporates carbon dioxide from the atmosphere into an organic molecule.
During the reduction stage, ATP provides energy and NADPH provides high-energy electrons to convert the fixed carbon into carbohydrates.
The final stage involves regenerating the original CO₂ acceptor, RuBP, so the cycle can continue. This requires additional ATP from the light-dependent reactions.
After multiple turns of the cycle, the plant can produce glucose and other carbohydrates essential for growth.
The Calvin Cycle is remarkably efficient but requires significant energy input. It takes six turns of the cycle, using eighteen ATP and twelve NADPH molecules, to fix six carbon dioxide molecules into one glucose molecule.
This remarkable process is responsible for creating most of the organic matter on Earth, transforming atmospheric carbon dioxide into the sugars that fuel nearly all life.
Chemoautotrophs generate energy through a process called chemosynthesis. Unlike photosynthesis, chemosynthesis doesn’t require light. Instead, these organisms obtain energy by oxidizing inorganic chemicals.
The basic chemosynthesis process involves converting inorganic chemicals into energy, which is then used to produce organic compounds.
Chemoautotrophs can use various inorganic chemicals as energy sources. Here are some common examples:
Some bacteria oxidize hydrogen sulfide. This reaction produces sulfur as a byproduct, which can create visible deposits around hydrothermal vents.
Another group of chemoautotrophs uses ammonia as their energy source, converting it to nitrite in the process.
Iron-oxidizing bacteria can derive energy from converting ferrous iron to ferric iron. These bacteria often create rusty deposits in their habitats.
Let’s break down the chemosynthesis process into three main stages:
First, chemoautotrophs oxidize inorganic compounds. This oxidation process transfers electrons to electron carriers like NAD+ or FAD, reducing them to NADH or FADH2.
These electron carriers then enter the electron transport chain, which generates a proton gradient used to produce ATP, the energy currency of the cell.
Finally, the ATP produced powers carbon fixation. This is where chemoautotrophs are similar to photoautotrophs – both use the Calvin Cycle to convert carbon dioxide into organic compounds.
While the energy sources for photosynthesis and chemosynthesis differ, both processes lead to the same carbon fixation pathway.
In both cases, the energy captured – whether from sunlight or chemical oxidation – powers the Calvin Cycle to fix carbon dioxide into organic compounds like glucose.
To summarize what we’ve learned about chemosynthesis:
Chemosynthesis derives energy from oxidizing inorganic chemicals. Common energy sources include hydrogen sulfide, ammonia, ferrous iron, methane, and hydrogen gas.
This process is common in extreme environments where light cannot reach, such as deep-sea hydrothermal vents and hot springs.
While the energy source differs from photosynthesis, both processes use the Calvin Cycle for carbon fixation. This makes chemosynthesis crucial for ecosystem functioning in environments where photosynthesis is impossible.
Autotrophs like plants don’t just create their own food through photosynthesis—they must also break it down to release energy.
Like all living organisms, autotrophs perform cellular respiration in specialized organelles called mitochondria.
Cellular respiration is the process where glucose is broken down using oxygen to produce carbon dioxide, water, and energy.
The glucose that autotrophs produce through photosynthesis becomes the fuel for cellular respiration.
Cellular respiration occurs in three main stages.
First, glycolysis breaks down glucose into pyruvate, generating a small amount of ATP energy.
Next, in the citric acid cycle, pyruvate is further broken down, releasing carbon dioxide and more energy.
Finally, the electron transport chain uses oxygen to generate the majority of ATP energy while producing water as a byproduct.
Autotrophs complete an elegant cycle between photosynthesis and cellular respiration.
During photosynthesis, they take in carbon dioxide and water, using sunlight to produce glucose and oxygen.
Then, during cellular respiration, they use that same glucose with oxygen to release energy for their cellular functions, while producing carbon dioxide as a byproduct.
This incredible cycle demonstrates how autotrophs efficiently manage their energy needs while maintaining environmental balance.
Let’s examine the fundamental differences between autotrophs and heterotrophs.
The key distinction is how they obtain energy. Autotrophs make their own food, while heterotrophs must consume other organisms.
Autotrophs harness energy directly from sunlight through photosynthesis, or from chemicals through chemosynthesis.
Heterotrophs, in contrast, must obtain their energy by consuming and digesting other organisms, either living or dead.
This fundamental difference in energy acquisition shapes their ecological roles and biological characteristics.
Examples of autotrophs include plants, algae, cyanobacteria, and certain types of archaea.
Heterotrophs include humans, animals, fungi, and most bacteria. These organisms must consume autotrophs or other heterotrophs to survive.
It’s important to remember that all food chains begin with autotrophs as primary producers. Without them, heterotrophs like us could not exist.
Section 12: Energy Acquisition Differences
Let’s contrast how autotrophs and heterotrophs obtain their energy.
Autotrophs, like plants, convert environmental energy into food. They harness energy from external sources such as sunlight.
In contrast, heterotrophs like animals must extract energy by consuming organic compounds. They get their energy by eating other organisms.
Let’s summarize the key differences in energy acquisition.
This fundamental difference in energy acquisition shapes the entire structure and behavior of these organisms, as well as their ecological roles.
Let’s explore how different feeding strategies affect the mobility and structure of organisms.
The fundamental difference between autotrophs and heterotrophs centers on how they obtain energy.
Autotrophs like plants are typically stationary organisms. They don’t need to move to find food.
Instead, they have specialized structures like leaves that maximize energy capture from sunlight.
Their roots extend into the soil to absorb water and nutrients, and their leaves are oriented to maximize exposure to sunlight.
In contrast, heterotrophs like animals must actively search for their food.
They have evolved mobility, allowing them to move toward food sources.
Heterotrophs possess specialized structures for finding, catching, and consuming other organisms, along with digestive systems to break down complex organic matter.
Let’s summarize the key structural and mobility differences that result from these different feeding strategies.
Autotrophs are typically stationary, with structures designed to maximize energy capture directly from the environment.
Heterotrophs, on the other hand, have evolved mobility and specialized structures for locating, capturing, and consuming food.
These structural and mobility differences reflect their fundamental feeding strategies, shaping how these organisms interact with their environments.
Food chains show how energy flows through ecosystems, and they all begin with autotrophs.
Autotrophs, also known as producers, form the foundation of all food chains. These organisms make their own food through photosynthesis or chemosynthesis.
Primary consumers, which are heterotrophs, feed directly on producers. These include herbivores like grasshoppers, rabbits, and many insects.
Secondary consumers feed on primary consumers. These are carnivores like frogs and small birds.
Tertiary consumers are at the top of many food chains. These are often apex predators, like snakes and hawks.
Energy flows through the food chain, starting from the producers. However, only about ten percent of energy transfers to each subsequent level.
Autotrophs are essential for ecosystems because they capture energy from the sun or chemicals and convert it into food. All other organisms depend on them directly or indirectly.
Heterotrophs, in contrast, must consume other organisms to obtain energy. They include herbivores that eat plants, carnivores that eat other animals, and omnivores that eat both.
In this simplified ecosystem diagram, we can see how energy flows from the sun to producers, then to consumers, with decomposers recycling nutrients back to producers.
Autotrophs capture energy from external sources, while heterotrophs must obtain energy by consuming other organisms. This fundamental difference determines their positions in food chains.
Primary production is the foundation of all ecosystems on Earth.
Autotrophs are responsible for converting inorganic carbon, like carbon dioxide, into organic compounds such as glucose.
This conversion requires energy, typically from sunlight for photoautotrophs or chemical energy for chemoautotrophs.
Autotrophs form the foundation of all food webs as primary producers.
The energy captured by autotrophs flows through the food web, starting with primary consumers that eat plants.
At each level of the food web, approximately 90 percent of energy is lost as heat, leaving only 10 percent for the next level.
The total energy available in any ecosystem is fundamentally limited by how much energy autotrophs can capture.
Autotrophs capture only about one percent of the sunlight energy that reaches Earth. This limitation determines how much life an ecosystem can support.
To summarize, primary production by autotrophs is essential because it creates the organic compounds that form the foundation of all food webs.
Global primary production from oceans and forests supports all life on Earth and sets the ultimate limits for biodiversity in ecosystems.
Photoautotrophs play a critical role in producing the oxygen we breathe.
These organisms, primarily plants, algae, and certain bacteria, use photosynthesis to convert sunlight into energy.
During photosynthesis, they take in carbon dioxide and water, and with the help of sunlight, convert these into glucose and oxygen.
Over billions of years, photosynthetic organisms have transformed Earth’s atmosphere.
The early Earth’s atmosphere contained virtually no oxygen, being composed mainly of carbon dioxide, nitrogen, and water vapor.
Around 2.4 to 2.2 billion years ago, in what scientists call the Great Oxygenation Event, photosynthetic cyanobacteria began releasing significant amounts of oxygen into the atmosphere.
Today, our atmosphere contains about 21 percent oxygen, making Earth suitable for complex aerobic life.
This oxygen is essential for aerobic organisms, including humans, fish, birds, and countless other species.
Through cellular respiration, these organisms use oxygen to convert glucose back into carbon dioxide and water, releasing energy that powers their life processes.
To summarize, photoautotrophs are responsible for producing the oxygen we breathe through photosynthesis. This process has transformed Earth’s atmosphere over billions of years, enabling the evolution and survival of complex aerobic life.
Autotrophs play a crucial role in regulating atmospheric carbon dioxide levels.
During photosynthesis, autotrophs remove carbon dioxide from the atmosphere. This simple chemical equation shows how carbon dioxide and water, with the help of light energy, are transformed into glucose and oxygen.
Autotrophs act as carbon sinks. This means they store carbon in their tissues, effectively removing it from the atmosphere for extended periods.
By removing carbon dioxide from the atmosphere, autotrophs help mitigate climate change. This graph shows how global temperatures would rise more rapidly without the carbon dioxide regulation provided by autotrophs.
Despite autotrophs’ vital role in carbon dioxide regulation, human activities like burning fossil fuels are releasing carbon dioxide faster than autotrophs can remove it from the atmosphere.
This is causing carbon dioxide levels to rise and overwhelming the natural regulatory processes that have kept our climate stable for millennia.
To summarize, autotrophs help regulate carbon dioxide levels by removing it from the atmosphere through photosynthesis and storing it in their tissues. This natural process helps mitigate climate change, but is currently being overwhelmed by human activities.
Autotrophs are ecosystem architects that create and maintain habitats supporting diverse life forms.
Forests are one of the most complex habitats created by autotrophs. Trees form multi-layered environments providing food, shelter, and protection for countless species.
Grasslands, dominated by grasses and other herbaceous plants, create open habitats with extensive root systems that stabilize soil and provide grazing areas.
Coral reefs represent a unique habitat where coral animals form symbiotic relationships with photosynthetic algae, creating complex three-dimensional structures that support incredible marine biodiversity.
Across different ecosystems, autotroph-created habitats serve multiple vital functions. They provide food resources, shelter, breeding grounds, protection from predators, and even regulate local climate conditions.
Autotrophs have evolved remarkable adaptations to survive and thrive in virtually every habitat on Earth, from scorching deserts to freezing polar regions and the deepest ocean floors.
Plants in desert environments have evolved specialized tissues to store water and minimize loss. These adaptations include succulent leaves and stems, thick waxy cuticles to reduce evaporation, and extensive root systems for efficient water collection.
Cacti are excellent examples of desert adaptation. Their specialized tissues can store large amounts of water during infrequent rainfall, allowing them to survive long periods of drought.
In the depths of the ocean, bacteria near hydrothermal vents have evolved specialized chemosynthetic pathways to derive energy from inorganic compounds like hydrogen sulfide and methane, rather than sunlight.
At hydrothermal vents, where no sunlight penetrates, bacteria have evolved to utilize chemicals like hydrogen sulfide and methane to produce energy through chemosynthesis.
In frigid arctic environments, algae and plants have developed specialized adaptations like antifreeze proteins that prevent ice crystal formation in their cells, allowing them to photosynthesize even at subzero temperatures.
These antifreeze proteins bind to ice crystals as they form, preventing them from growing larger and damaging the cell. This remarkable adaptation allows algae to maintain membrane fluidity and continue photosynthesis even in freezing waters.
Through these remarkable evolutionary adaptations, autotrophs have developed the ability to thrive in virtually every environment on Earth, from the hottest deserts to the coldest polar regions and the deepest ocean floors.
Autotrophs face numerous threats that endanger their survival and ecological functions. Let’s examine four major threats.
Deforestation removes vast areas of photosynthetic plants, primarily for agriculture, urban development, and logging.
Ocean acidification occurs as oceans absorb more carbon dioxide, lowering pH levels and harming marine autotrophs like algae and phytoplankton.
Pollution, including air pollutants and water contaminants, damages plant tissues and disrupts photosynthetic processes.
Climate change brings rising temperatures, altered precipitation patterns, and extreme weather events that disrupt plant growth cycles and habitats.
These threats to autotrophs don’t exist in isolation. Because autotrophs form the foundation of food webs, damage to these organisms cascades throughout entire ecosystems.
Let’s visualize how damage to autotrophs affects the entire ecosystem. At the base, we have autotrophs, the primary producers.
Above them are primary consumers that feed directly on autotrophs.
Then secondary consumers that prey on the primary consumers.
And finally top predators at the highest trophic level.
When autotrophs are damaged or destroyed, the effects cascade throughout the entire food web.
As autotrophs decline, there is less food available for primary consumers, whose populations then decline.
With fewer primary consumers, secondary consumers face food shortages, affecting their populations.
Finally, top predators face dwindling prey, potentially leading to local extinctions and ecosystem collapse.
This is why protecting autotrophs is not just about saving plants or algae—it’s about preserving entire ecosystems and biodiversity.
As we conclude our exploration of autotrophs, let’s reflect on their critical role in Earth’s future.
Autotrophs are truly fundamental to all life on Earth. They form the base of every food web, generate oxygen, and play a crucial role in carbon sequestration.
Conservation efforts worldwide are now focusing on protecting autotrophs both on land and in our oceans.
Sustainable practices like reforestation, reducing agricultural runoff, and creating marine protected areas are vital for maintaining healthy autotroph populations.
Autotrophs are the foundation of ecosystem stability. They directly impact biodiversity, climate regulation, water quality, and food security.
We cannot overstate how essential autotrophs are for human survival. Beyond providing food and oxygen, they moderate climate impacts, support medical discoveries, and maintain water and soil health.
As stewards of Earth, we have a responsibility to protect autotrophs through conservation policies, sustainable choices, and education. In protecting them, we protect our planet’s future and our own.
Study Materials
No study materials available for this video.
Helpful: 0%
Related Videos
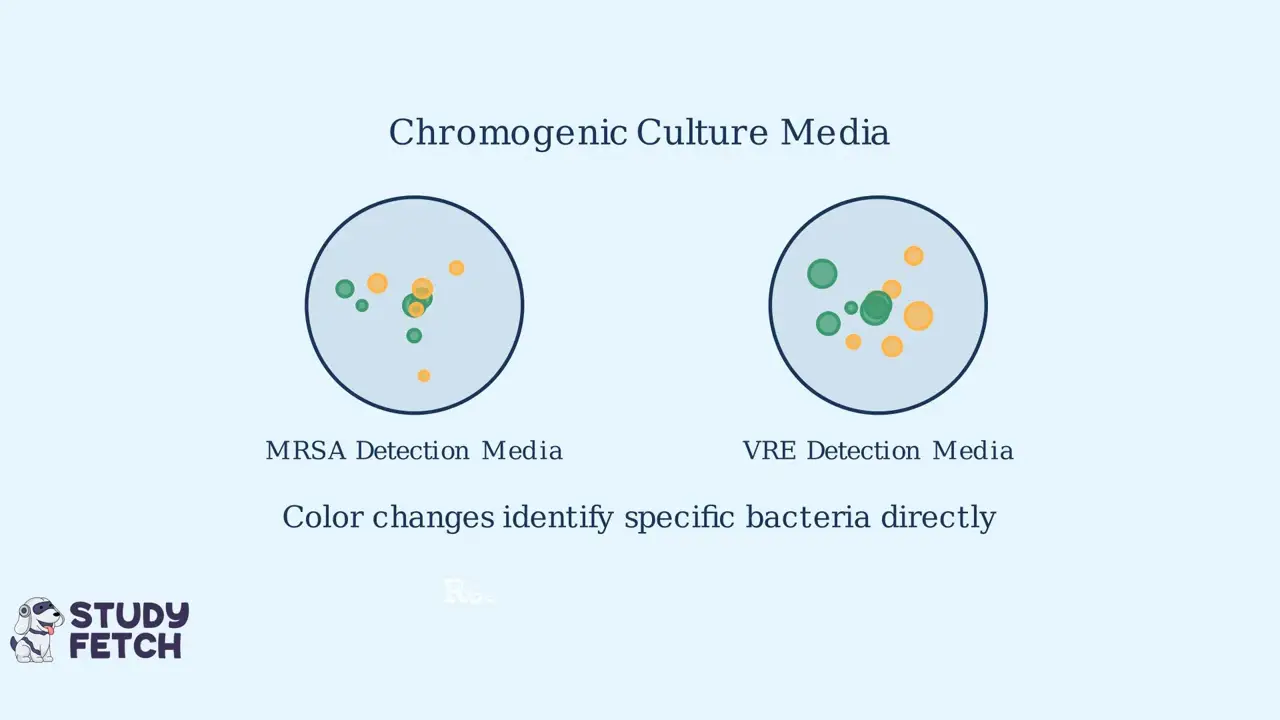
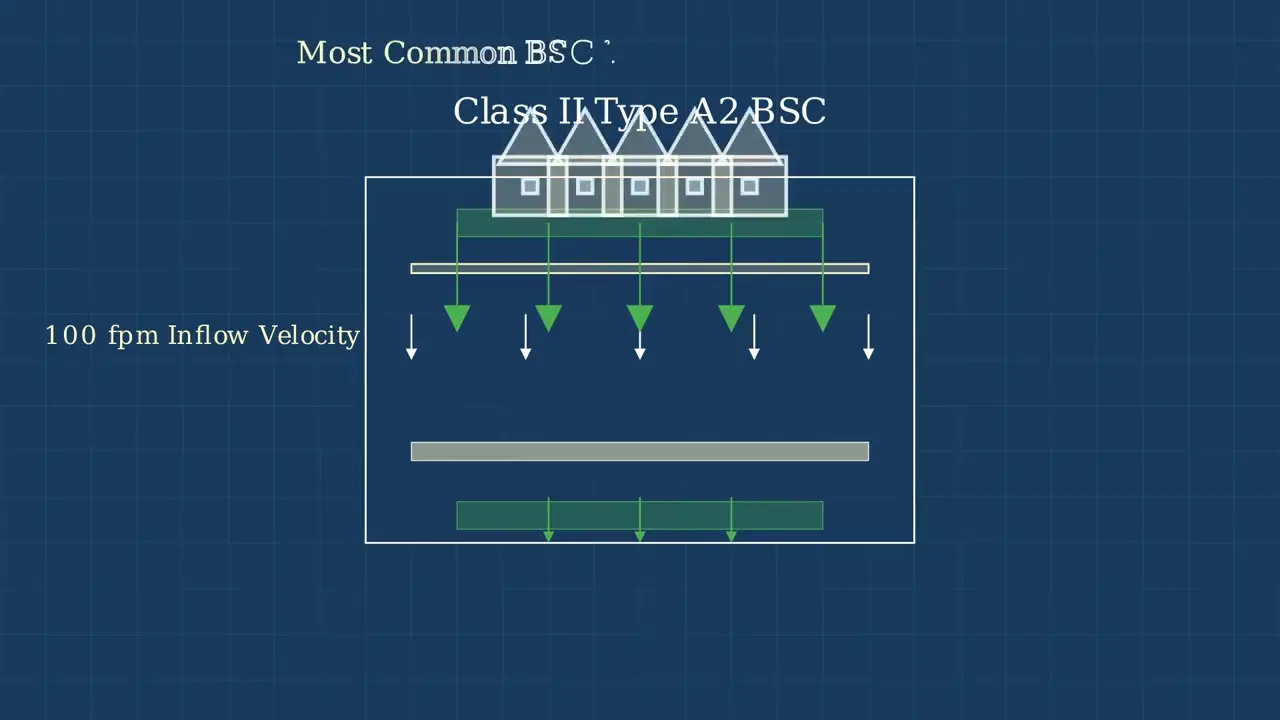
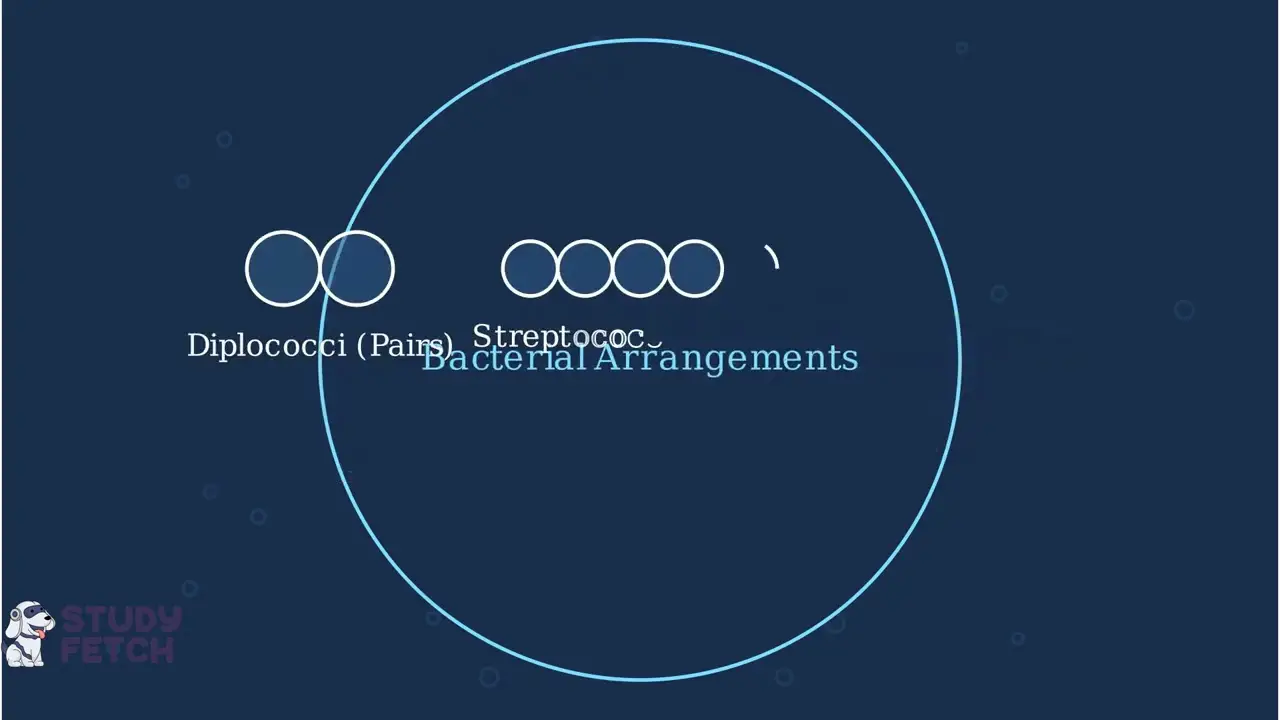
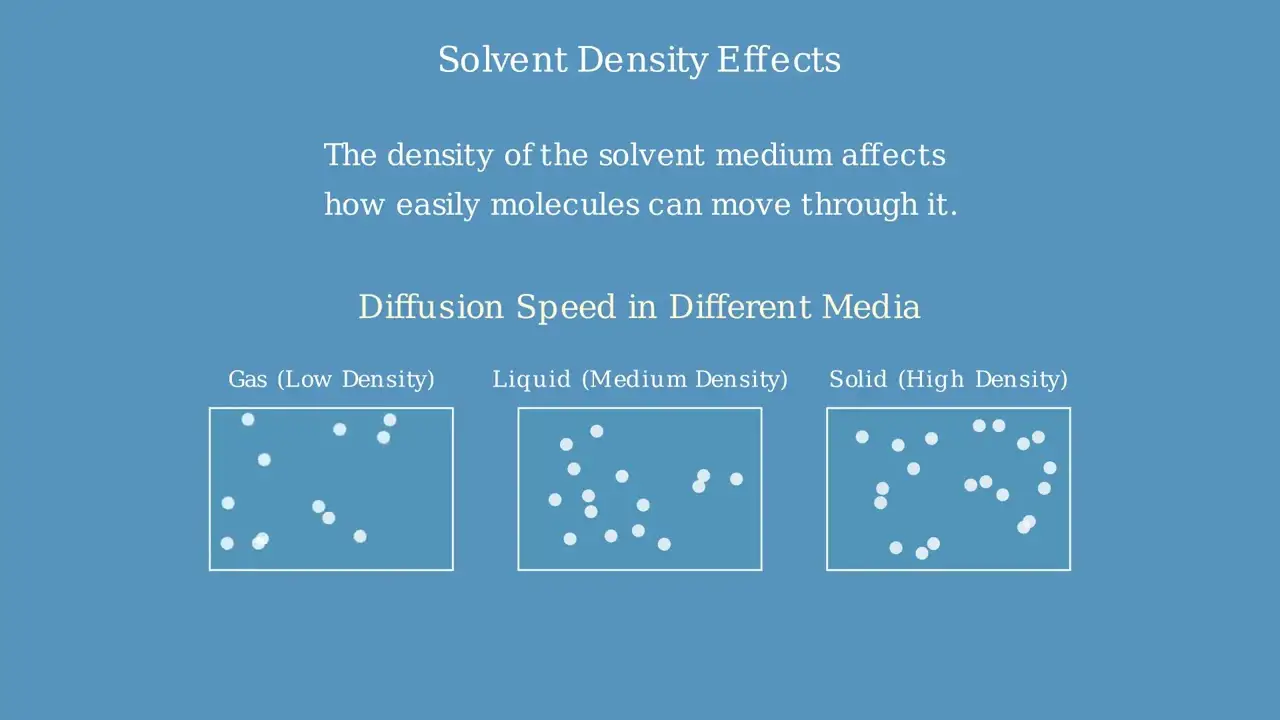
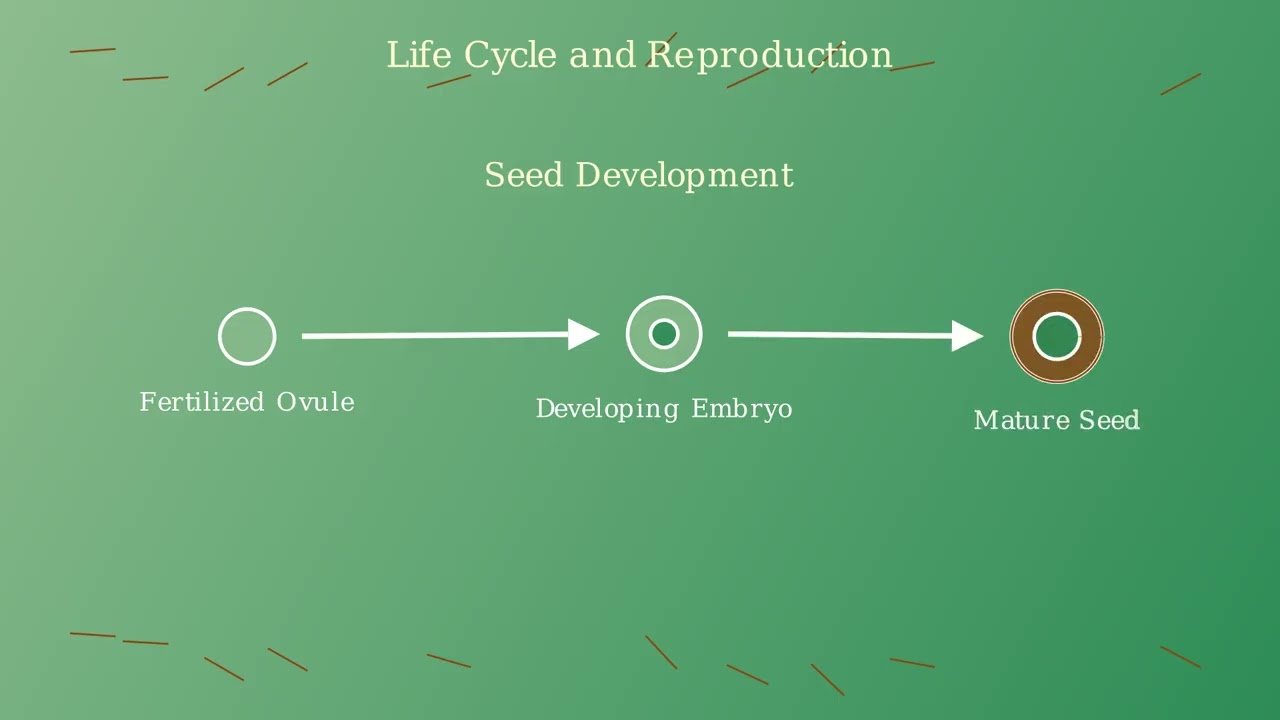
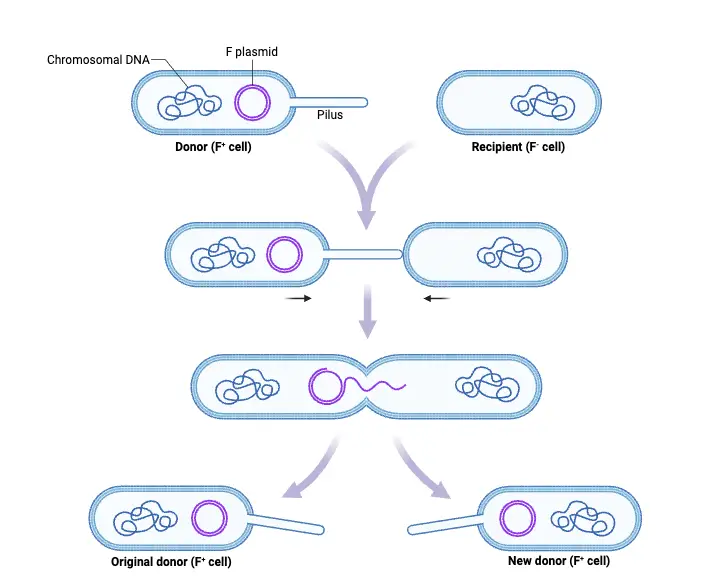
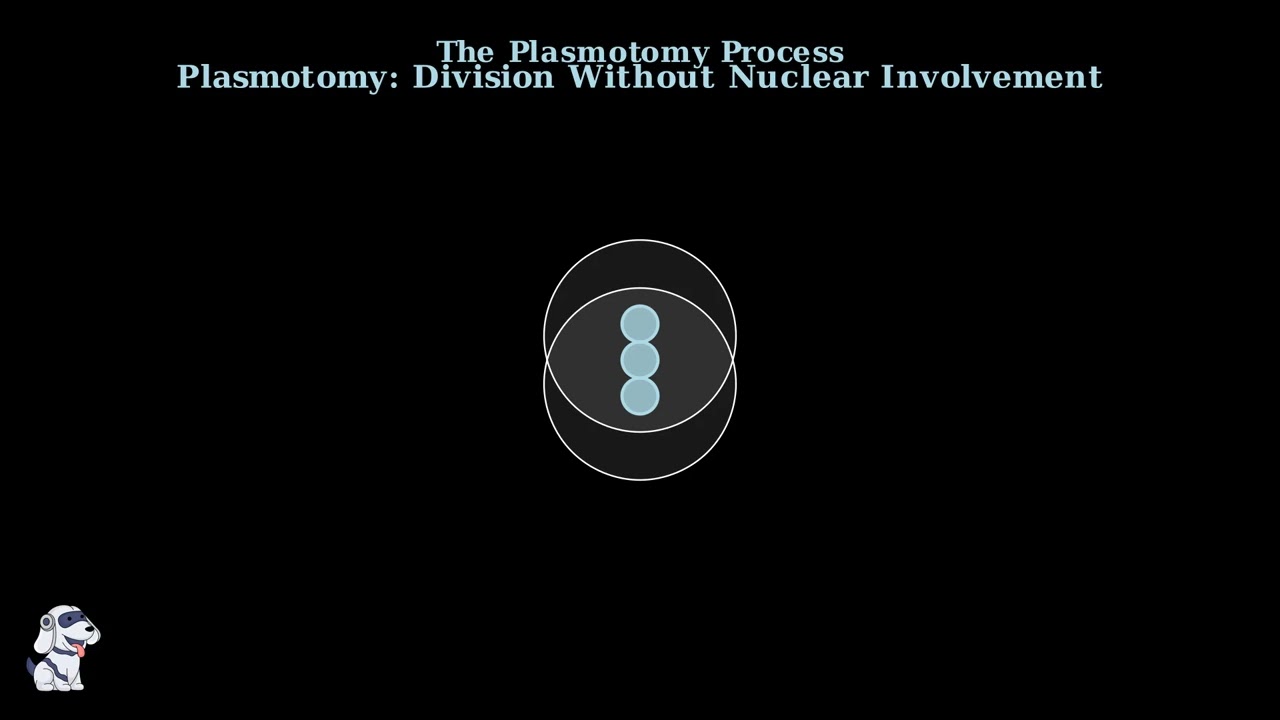
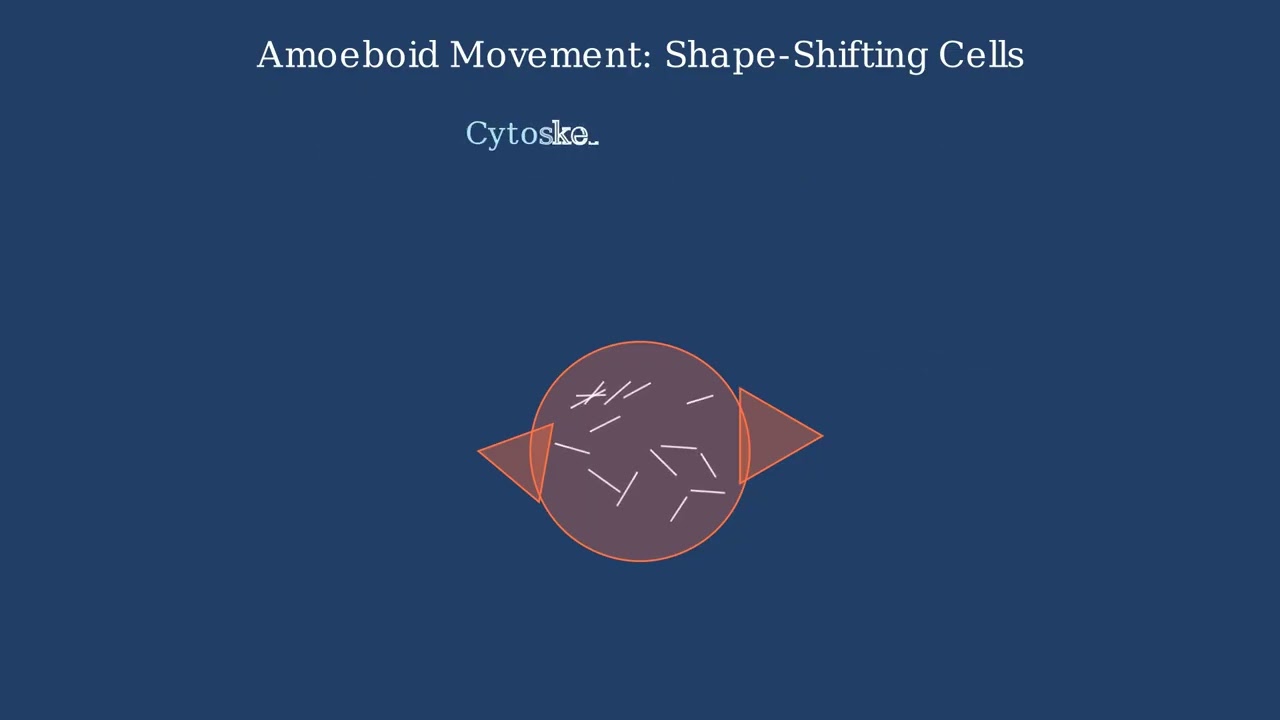
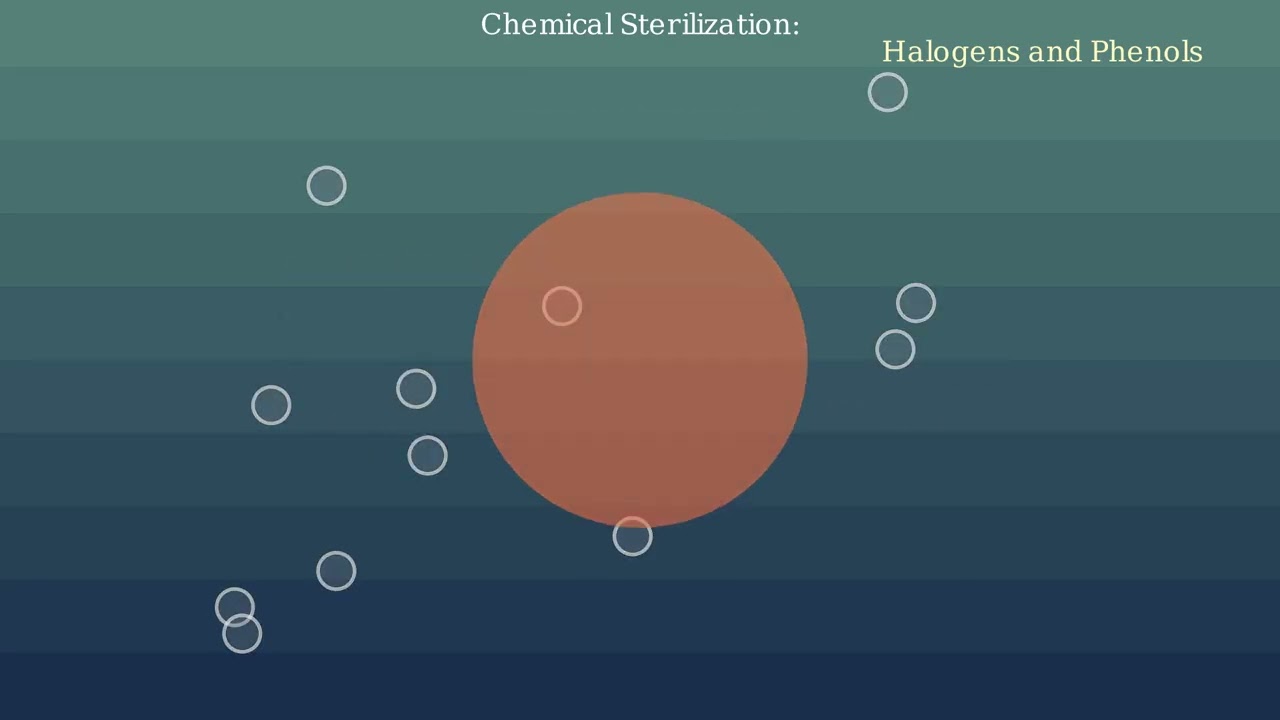
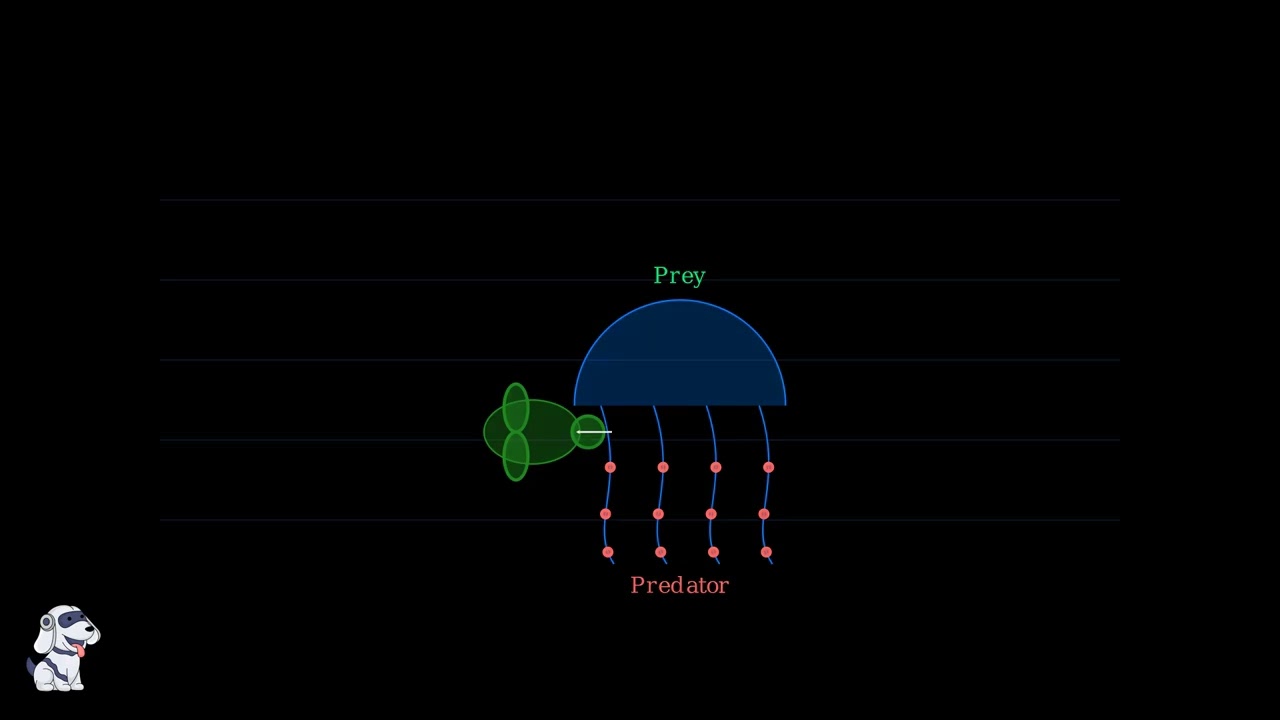