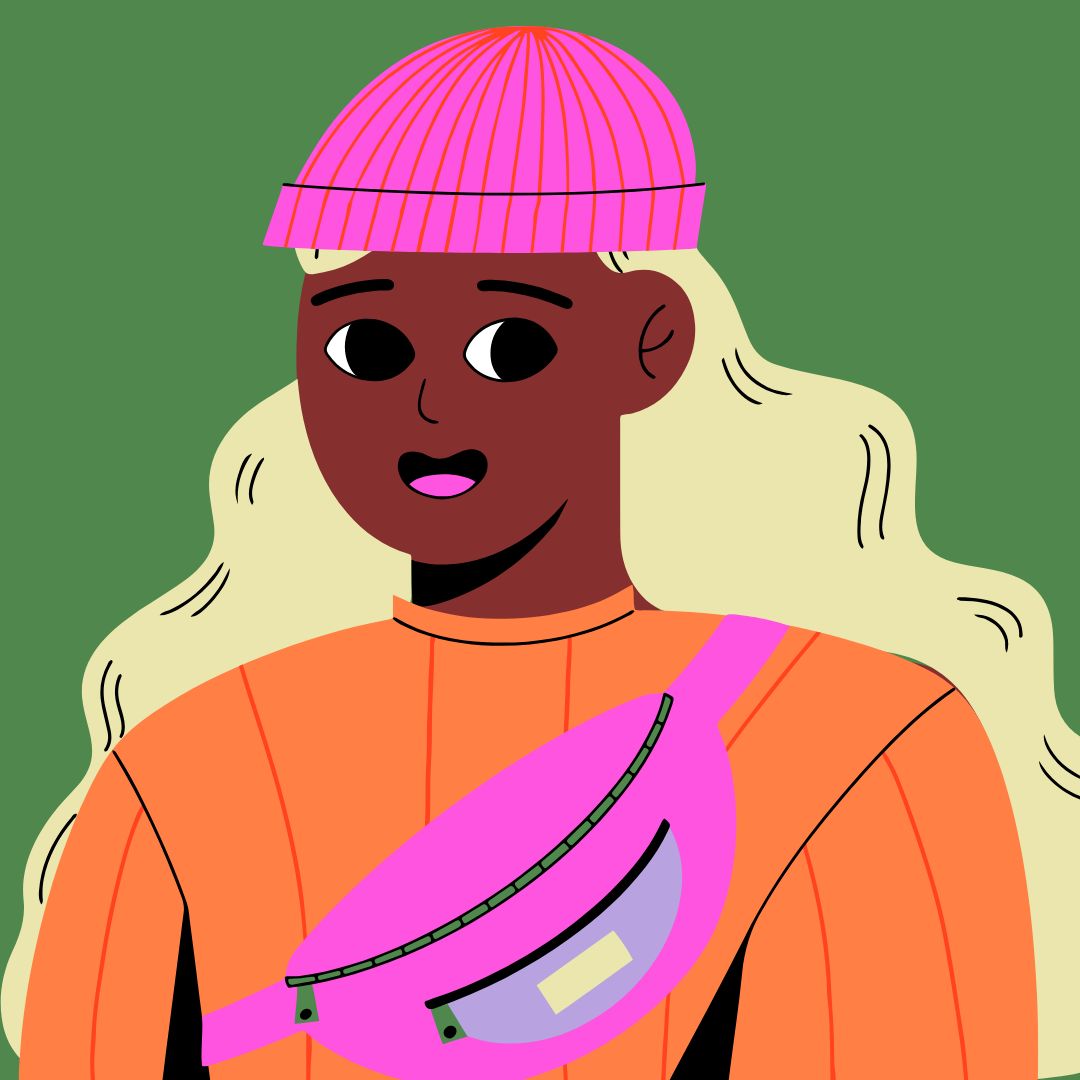
Sourav Pan
Transcript
What is osmotic pressure?
Osmotic pressure is the pressure required to prevent the flow of solvent through a semi-permeable membrane when there’s a difference in solute concentration between two solutions.
Let’s visualize this concept. Here we have two solutions separated by a semi-permeable membrane.
The left side has a high concentration of solute particles, while the right side has a lower concentration.
The natural tendency is for water to flow from the low concentration side to the high concentration side to equalize the concentrations.
Osmotic pressure is the external pressure that must be applied to prevent this solvent flow across the membrane.
Importantly, osmotic pressure is a colligative property, meaning it depends on the number of particles in solution, rather than their chemical identity.
For example, a solution with twenty salt ions would have higher osmotic pressure than a solution with ten sugar molecules, even though the sugar molecules are larger.
To summarize, osmotic pressure is the pressure needed to prevent solvent flow through a semi-permeable membrane, and it depends on the number of particles in solution rather than their identity.
The mechanism of osmosis occurs at the molecular level across a semi-permeable membrane.
A semi-permeable membrane separates two solutions with different solute concentrations.
The left side has a lower solute concentration, while the right side has a higher concentration of solute molecules.
The semi-permeable membrane allows the smaller water molecules to pass through its pores, but blocks the larger solute molecules.
This process continues until equilibrium is reached, or until osmotic pressure prevents further water movement.
In summary, osmosis is the movement of water molecules through a semi-permeable membrane from an area of lower solute concentration to higher solute concentration, continuing until equilibrium is reached.
The Van’t Hoff equation provides a way to calculate the theoretical osmotic pressure of a solution.
The equation is expressed as Pi equals i C R T.
This equation shows that osmotic pressure is directly proportional to concentration and temperature.
Let’s calculate the osmotic pressure of a 0.1 molar glucose solution at twenty-five degrees Celsius, or two hundred ninety-eight Kelvin.
Since glucose doesn’t dissociate in water, the van’t Hoff factor is one. Plugging all values into our equation, we get an osmotic pressure of two point four five atmospheres.
The Van’t Hoff equation provides scientists and engineers with a powerful tool to predict and understand osmotic pressure in various solutions and biological systems.
This fundamental equation connects osmotic pressure to basic solution properties, helping us understand osmotic phenomena across chemistry, biology, and medicine.
Hypoosmotic or hypotonic solutions have a lower solute concentration than a reference solution.
This lower concentration results in lower osmotic pressure compared to the reference solution.
When cells are placed in a hypotonic environment, the concentration gradient causes water to flow into the cells.
Let’s compare a reference solution with a hypotonic solution. Notice how the hypotonic solution has fewer solute particles.
When a cell is placed in a hypotonic environment, water flows into the cell due to the concentration gradient.
As water enters the cell, it begins to swell, increasing in volume while the solute concentration inside decreases.
If too much water enters the cell, it may eventually burst – a process called lysis. This happens because the cell membrane cannot withstand the increasing pressure.
Let’s look at some real-world examples of hypotonic solutions. Distilled water is hypotonic to blood cells, which is why red blood cells burst when placed in pure water.
Fresh water is hypotonic to marine organisms. This is why saltwater fish cannot survive in fresh water – their cells would take in too much water and rupture.
Sports drinks that are diluted too much with water can become hypotonic to your body’s cells.
In medical settings, intravenous fluids with salt concentrations less than 0.9% sodium chloride are considered hypotonic and must be used carefully.
Hypotonic solutions have important clinical applications. They can be used therapeutically to hydrate cells when needed.
However, hypotonic conditions can be dangerous, especially for brain cells, potentially leading to cerebral edema or brain swelling.
They may also damage fragile tissues by causing excessive swelling, which is why proper osmotic balance is crucial in medical treatments.
Hyperosmotic or hypertonic solutions contain a higher concentration of solutes than the reference solution.
In a hyperosmotic environment, the solute concentration outside the cell is higher than inside the cell.
This creates an osmotic pressure gradient that causes water to flow out of the cell through the semi-permeable membrane.
As water leaves the cell, the cell shrinks and may undergo a process called crenation, where the cell membrane becomes irregular and wrinkled.
The loss of water causes the cell to shrink, and in extreme cases, the cell membrane becomes wrinkled and irregular in a process called crenation.
Examples of hyperosmotic solutions include concentrated salt solutions, seawater, three percent sodium chloride solution used in laboratories, and hypertonic intravenous solutions used in medical settings.
Plant cells rely on osmotic pressure to maintain their structure and rigidity.
Unlike animal cells, plant cells have a rigid cell wall surrounding the cell membrane, with a large central vacuole filled with water and dissolved substances.
Turgor pressure occurs when water moves into the plant cell through osmosis.
Water molecules flow from areas of lower solute concentration to higher solute concentration across the semi-permeable cell membrane.
As water enters, it creates pressure that pushes outward against the rigid cell wall. This pressure is called turgor pressure.
Turgor pressure is essential for maintaining plant structure. It provides the rigidity plants need to stand upright.
When plants lose water, turgor pressure decreases, and the cells become less rigid. This causes the plant to wilt and droop.
Let’s compare a turgid cell with a plasmolyzed cell. A turgid cell is filled with water, pressing against the cell wall.
In a hypertonic environment, where the concentration of solutes outside the cell is higher, water leaves the cell through osmosis. This causes the cell membrane to pull away from the cell wall, a process called plasmolysis.
Let’s observe how a plant wilts and recovers. When a plant loses water, the cells lose turgor pressure, and the plant begins to droop.
When water is reintroduced, osmosis causes water to flow back into the cells, restoring turgor pressure. The plant becomes rigid and upright again.
To summarize the key points about osmotic pressure in plant cells: Turgor pressure results from osmotic water movement into the cell. The rigid cell wall provides structure to withstand this pressure. When plants lose water, turgor pressure decreases, causing wilting. And in hypertonic environments, water leaves the cell, causing plasmolysis.
Kidneys use osmotic pressure to filter blood and regulate water balance in the body.
The functional unit of the kidney is the nephron, which consists of several specialized segments.
The kidney creates and maintains a concentration gradient in the medulla, with increasing solute concentration toward the inner medulla.
Blood filtration begins in the glomerulus, where water and small solutes pass into Bowman’s capsule due to pressure gradients.
In the proximal tubule, essential nutrients, ions, and water are reabsorbed into the bloodstream.
The Loop of Henle is crucial for creating the concentration gradient through a process called countercurrent multiplication.
As fluid flows down the descending loop, water leaves via osmosis. In the ascending loop, sodium chloride is actively pumped out, but the tubule is impermeable to water.
In the collecting duct, water reabsorption occurs via osmosis, following the concentration gradient established by the Loop of Henle.
The hormone ADH regulates water permeability in the collecting duct, allowing the body to produce either concentrated or dilute urine depending on hydration status.
When ADH levels are low, the collecting duct remains relatively impermeable to water, resulting in dilute urine. When ADH levels are high, water is reabsorbed, producing concentrated urine.
Through these osmotic processes, the kidneys precisely regulate water and solute balance, maintaining homeostasis in the body.
Neurons rely on specific ion concentrations and osmotic balance to function properly.
The neuronal membrane separates different ion concentrations. Inside the cell, potassium is high, while sodium and chloride are low.
These ion channels regulate the movement of specific ions across the membrane.
These ion concentration differences create the resting membrane potential of about negative seventy millivolts.
When a neuron is stimulated, it generates an action potential – a rapid change in membrane voltage that propagates along the axon.
Osmotic imbalances can severely disrupt neuron function. In normal conditions, ion concentrations are carefully regulated.
In a hypoosmotic environment, water flows into the cell, causing it to swell and diluting ion concentrations.
In a hyperosmotic environment, water leaves the cell, causing it to shrink and concentrating ions.
These osmotic imbalances lead to various neurological symptoms. Hypoosmotic conditions can cause seizures, while hyperosmotic conditions can lead to confusion and impaired neural function.
In summary, proper osmotic balance is critical for maintaining normal neuronal function and preventing neurological disorders.
Reverse osmosis is an industrial water purification technology that applies pressure to overcome natural osmotic pressure.
To understand reverse osmosis, let’s first look at natural osmosis, where water naturally moves from low to high solute concentration.
In normal osmosis, water molecules move across the membrane from an area of low solute concentration to high solute concentration.
In reverse osmosis, external pressure is applied to overcome the natural osmotic pressure, forcing water in the opposite direction.
A typical reverse osmosis system consists of several key components. Feed water enters a high-pressure pump, which forces it through a semi-permeable membrane.
The membrane allows water molecules to pass through while blocking contaminants like salts, bacteria, and other impurities. The clean water or permeate is collected, while the concentrate containing rejected contaminants is discharged.
The pressure forces water molecules through the membrane, separating them from contaminants. This produces clean water while concentrating the impurities in the waste stream.
Reverse osmosis has several major industrial applications. The most notable is desalination, which converts seawater into freshwater by removing salt and other minerals.
In municipal water treatment, reverse osmosis removes contaminants, bacteria, and viruses from drinking water supplies, improving water quality, taste, and odor.
Industries use reverse osmosis to recycle process water, treat wastewater, and concentrate valuable compounds, reducing both resource consumption and disposal costs.
To summarize, reverse osmosis is a pressure-driven membrane process that overcomes natural osmotic pressure to purify water. It’s a versatile technology with applications spanning from desalination to industrial water recycling.
Food preservation through osmotic pressure is one of the oldest and most effective methods of extending food shelf life.
When microorganisms encounter a hyperosmotic environment rich in salt or sugar, water is drawn out of their cells through osmosis.
This dehydration causes the microbial cells to shrink, deactivates their enzymes, inhibits their growth, and ultimately leads to their death.
Traditional food preservation methods leverage osmotic pressure to extend shelf life.
Many popular preserved foods rely on osmotic principles. Beef jerky uses salt to draw out moisture. Fruit jams use sugar’s osmotic properties. Pickles combine salt and acid in a brining process.
While traditional osmotic preservation methods have been used for millennia, modern food technology has refined these techniques with precise control of water activity and combined preservation approaches.
In summary, osmotic food preservation creates environments where water is drawn out of microorganisms, preventing their growth and extending food shelf life using natural principles.
There are several laboratory methods for measuring osmotic pressure, each with specific applications and limitations.
Osmotic pressure can be measured using both direct and indirect methods.
Direct measurement uses osmometers, which typically consist of a chamber divided by a semi-permeable membrane. The pressure required to prevent water flow across the membrane equals the osmotic pressure.
Membrane osmometers directly measure the hydrostatic pressure needed to halt osmosis. Colloid osmometers are specifically designed to measure oncotic pressure from proteins in biological fluids.
Indirect methods utilize colligative properties like freezing point depression and vapor pressure lowering to calculate osmotic pressure.
Freezing point osmometers are most commonly used in clinical laboratories. They measure how much the freezing point of a solution is lowered compared to pure water, then convert this to osmolality, expressed in milliosmoles per kilogram.
Measuring osmolality is crucial in clinical settings for assessing fluid and electrolyte balance. The normal serum osmolality ranges from 275 to 295 milliosmoles per kilogram.
Clinical applications include assessing hydration status, diagnosing electrolyte disorders, monitoring conditions like diabetes insipidus, evaluating kidney function, and detecting toxic ingestions.
Modern laboratory equipment for measuring osmotic pressure includes freezing point and vapor pressure osmometers.
These devices offer automated sampling and measurement, providing results in just one to two minutes. They typically require only a small sample volume, usually between twenty and fifty microliters.
To summarize, osmotic pressure can be measured through both direct methods using membrane osmometers and indirect methods using freezing point depression or vapor pressure lowering. These measurements provide critical information for clinical assessment of fluid and electrolyte balance.
Let’s summarize the key concepts of osmotic pressure.
Osmotic pressure is the pressure required to prevent the flow of water across a semi-permeable membrane from a region of lower solute concentration to a region of higher solute concentration.
The Van’t Hoff equation, Pi equals i times C times R times T, mathematically describes osmotic pressure.
Here, Pi represents osmotic pressure, i is the Van’t Hoff factor accounting for dissociation, C is molar concentration, R is the gas constant, and T is absolute temperature.
Solutions can be classified based on their osmotic pressure relative to a reference solution.
Isoosmotic solutions have equal concentrations of solutes, resulting in no net water movement.
Hypoosmotic solutions have lower concentrations of solutes, causing water to flow into cells.
Hyperosmotic solutions have higher concentrations of solutes, drawing water out of cells.
Osmotic pressure plays critical roles in various biological systems.
It regulates cell volume and homeostasis, maintains plant cell turgor, drives kidney filtration, balances blood plasma, and supports nerve cell function.
The principles of osmotic pressure are applied in numerous practical technologies.
These include medical applications like IV fluids and dialysis, industrial processes like reverse osmosis, food preservation, and environmental technologies like desalination.
All these concepts interconnect in a framework centered around osmotic pressure.
At the center is osmotic pressure itself, connected to the mathematical Van’t Hoff equation, different solution types, biological significance, practical applications, measurement methods, and factors affecting osmotic pressure.
In conclusion, osmotic pressure is a fundamental physical principle that underlies countless natural processes and technologies. Understanding this concept is essential in fields ranging from cell biology to industrial applications.
Study Materials
No study materials available for this video.
Helpful: 0%
Related Videos
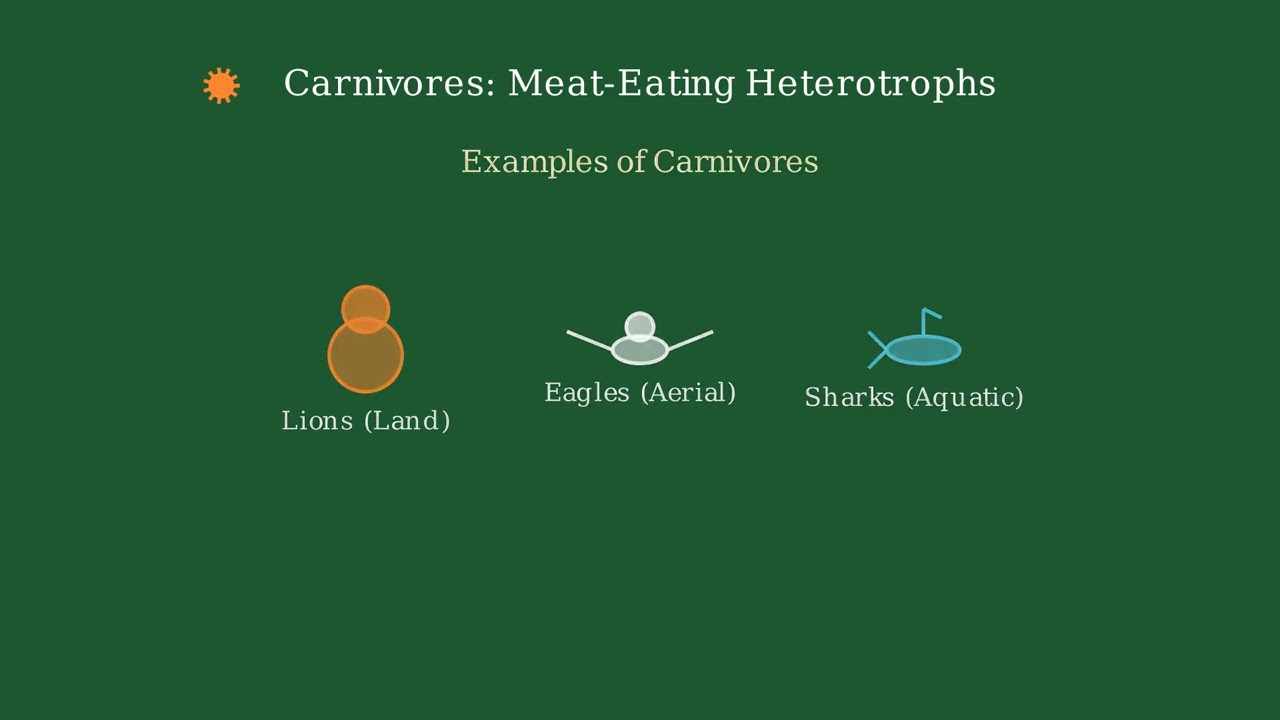
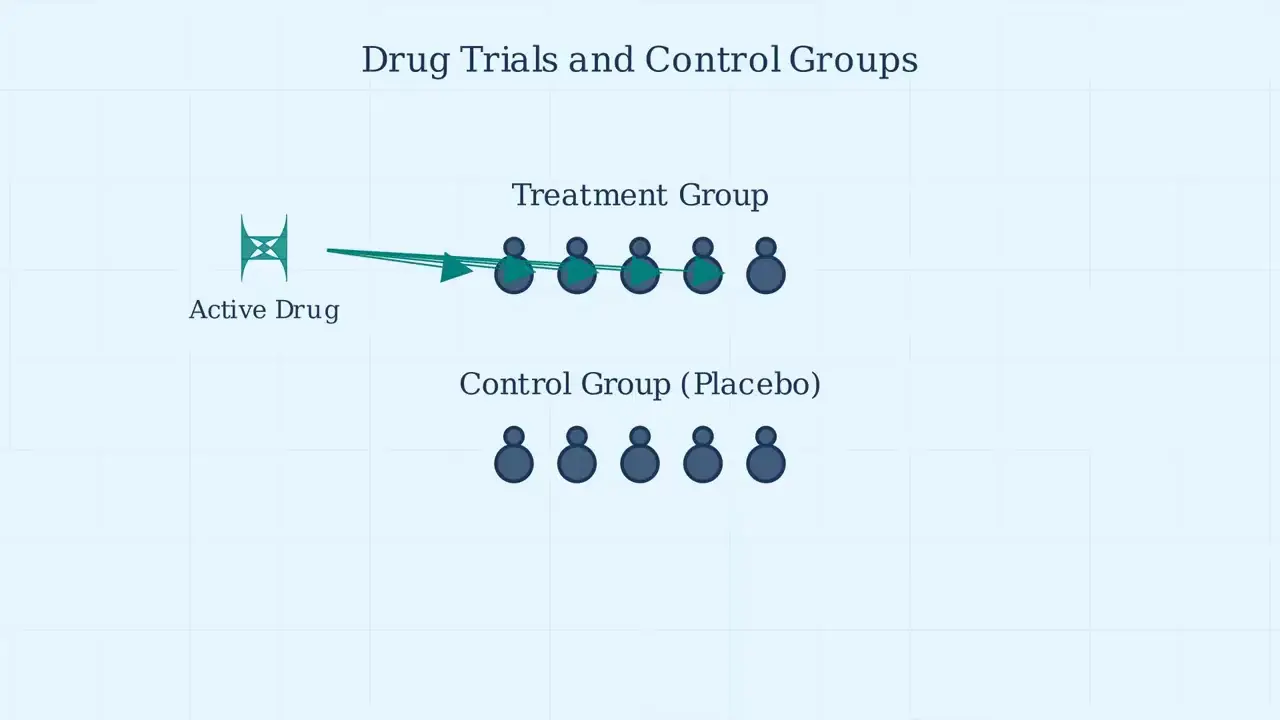
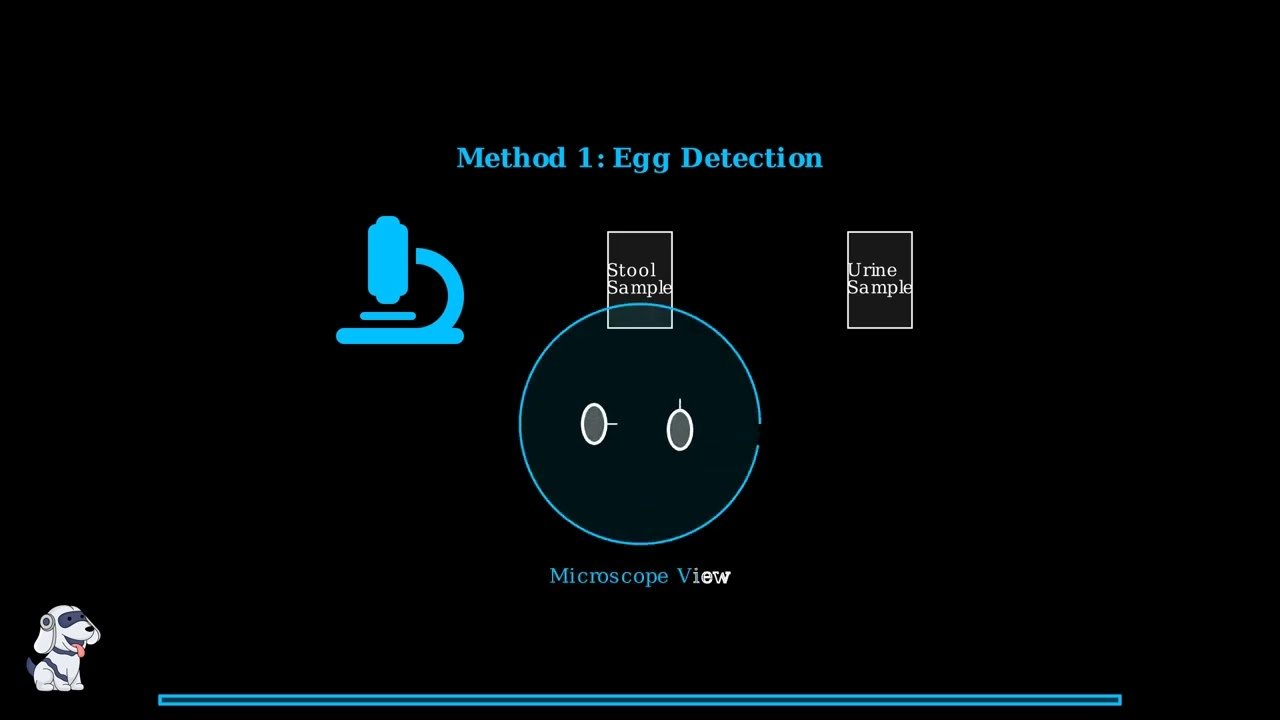
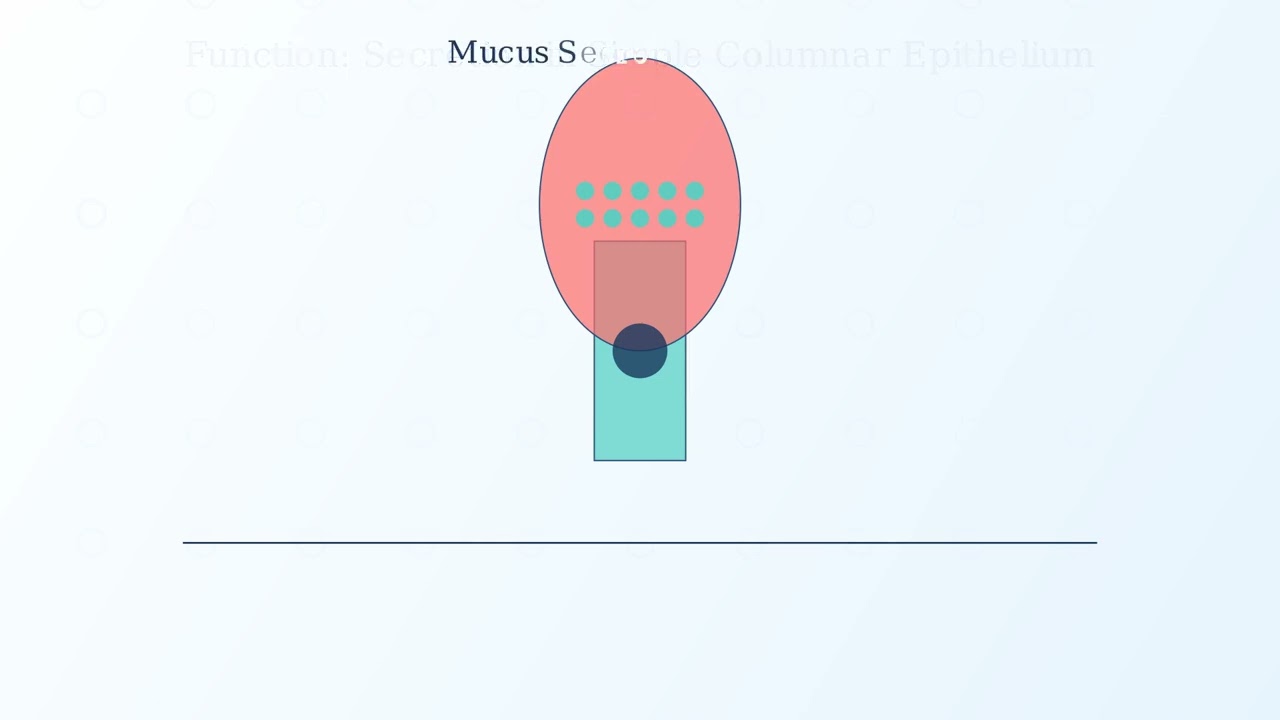
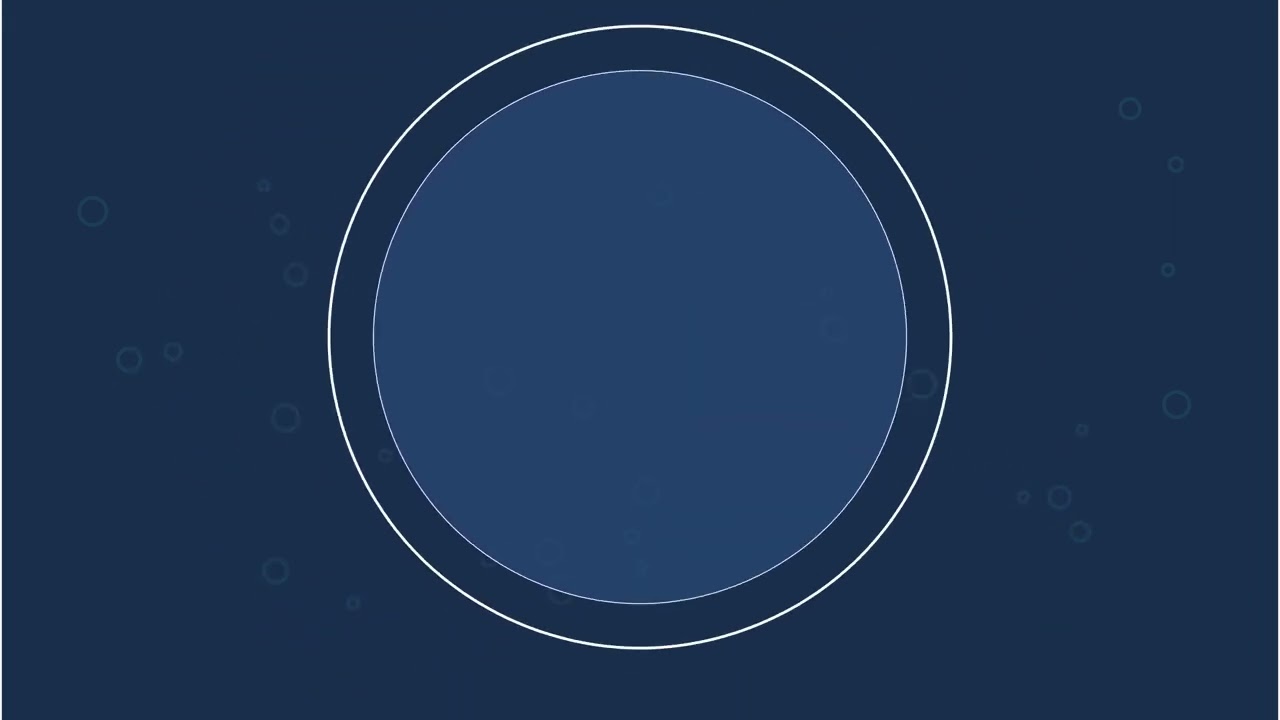
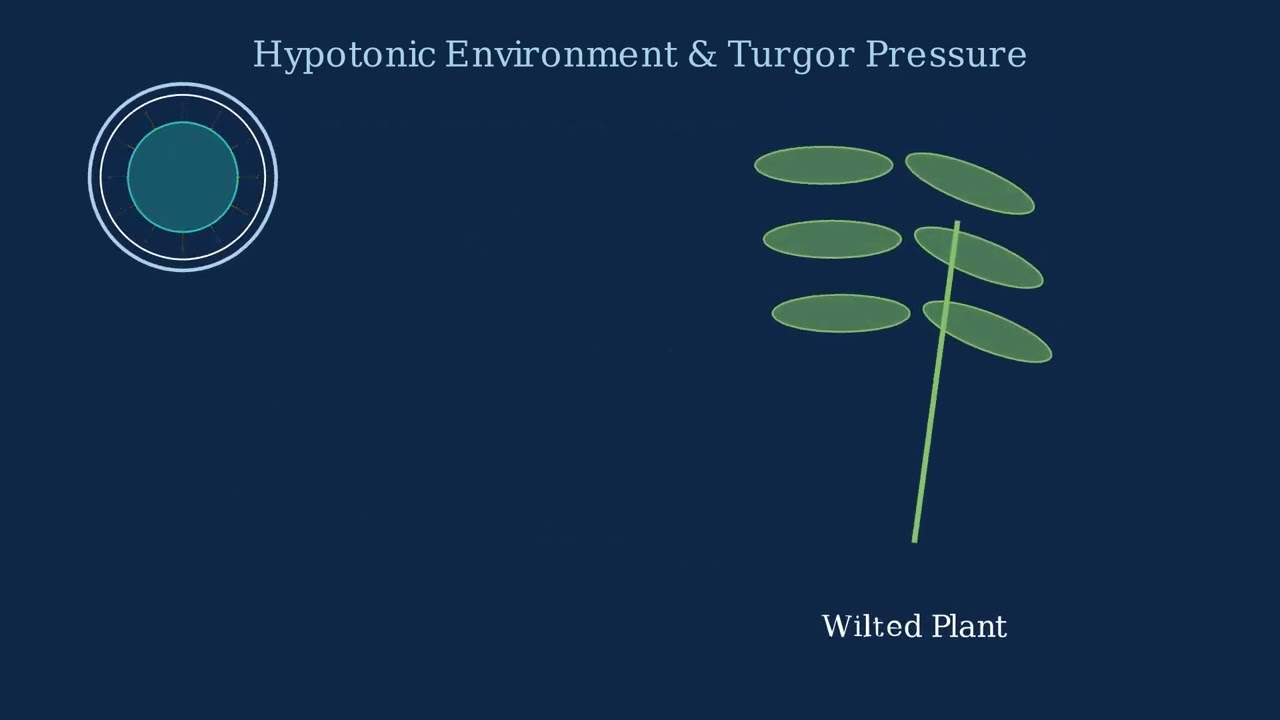
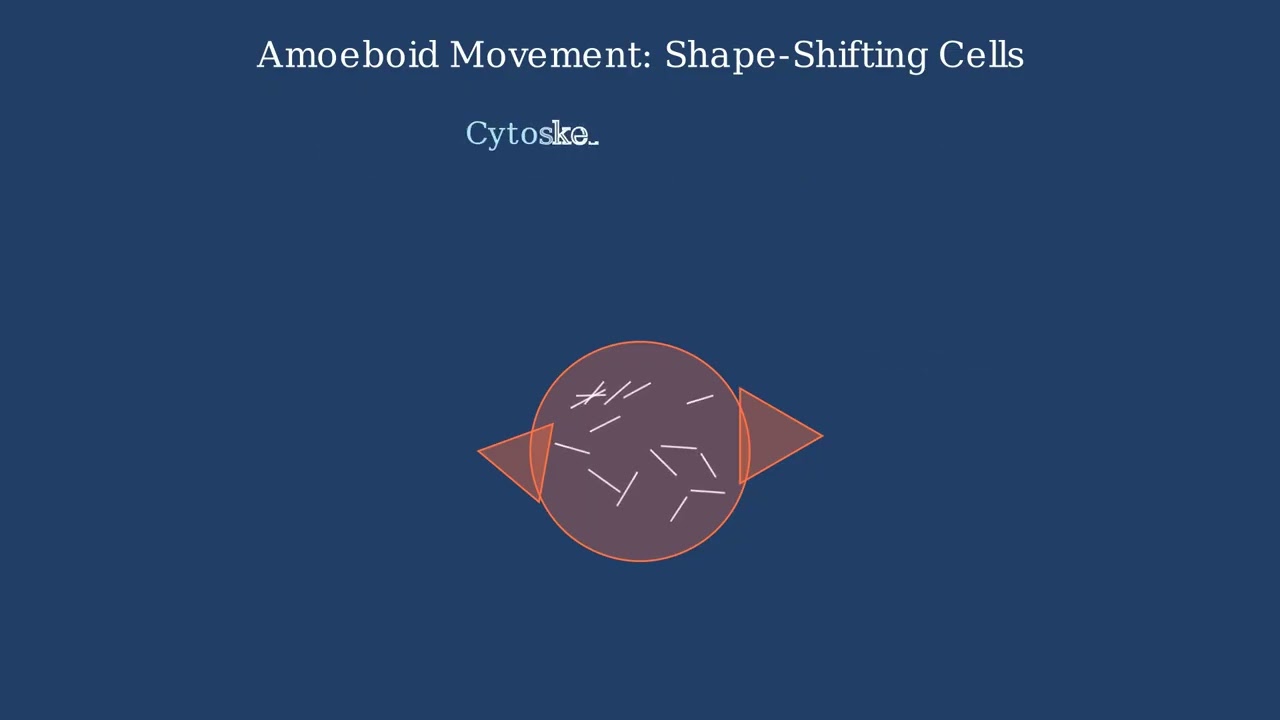
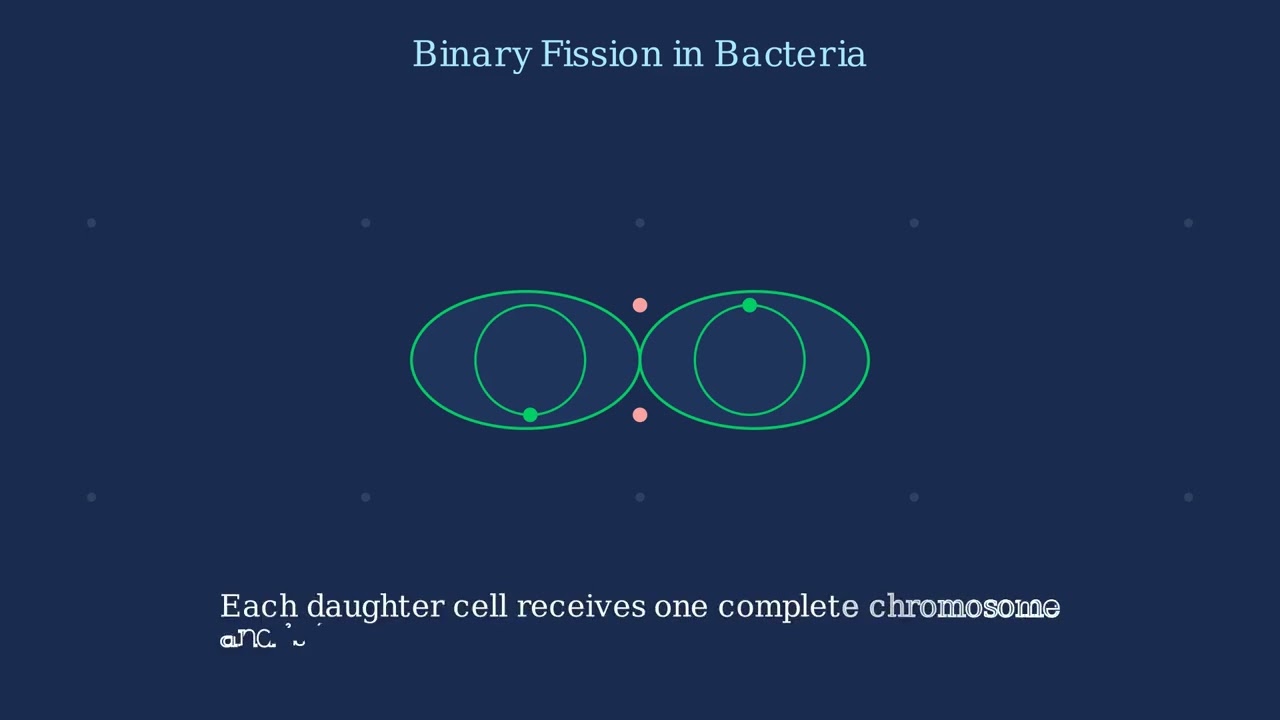
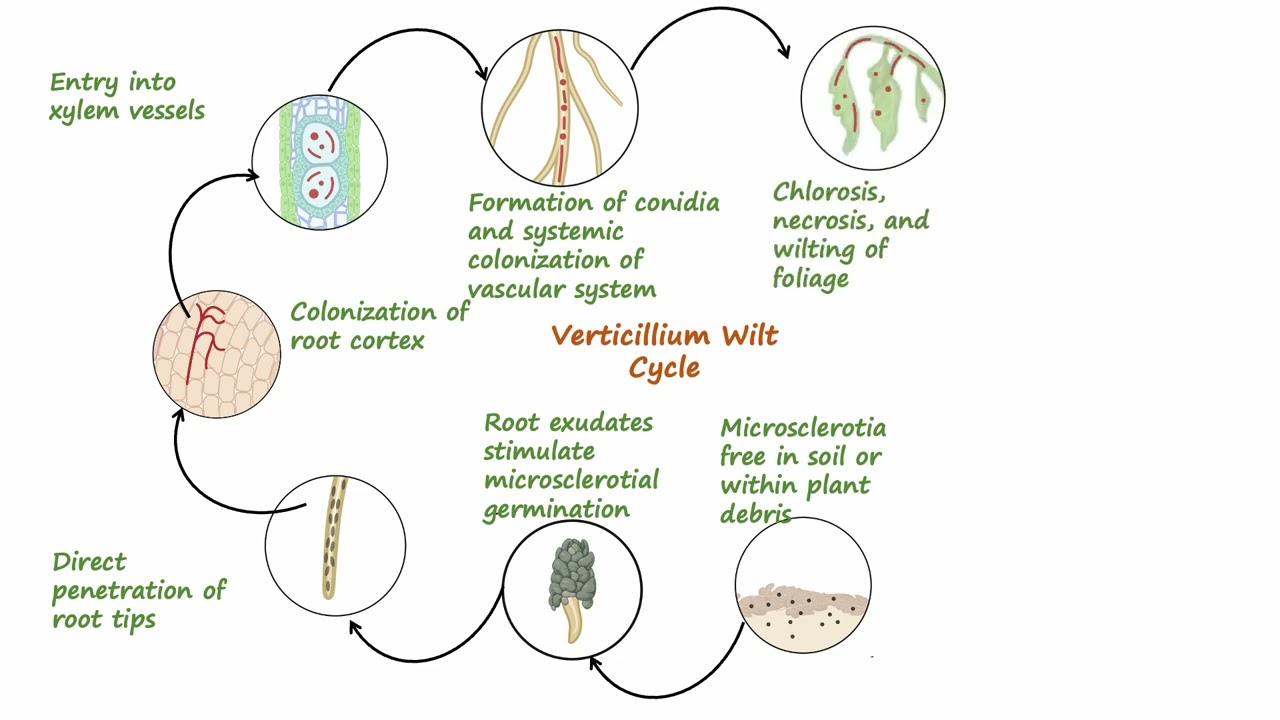
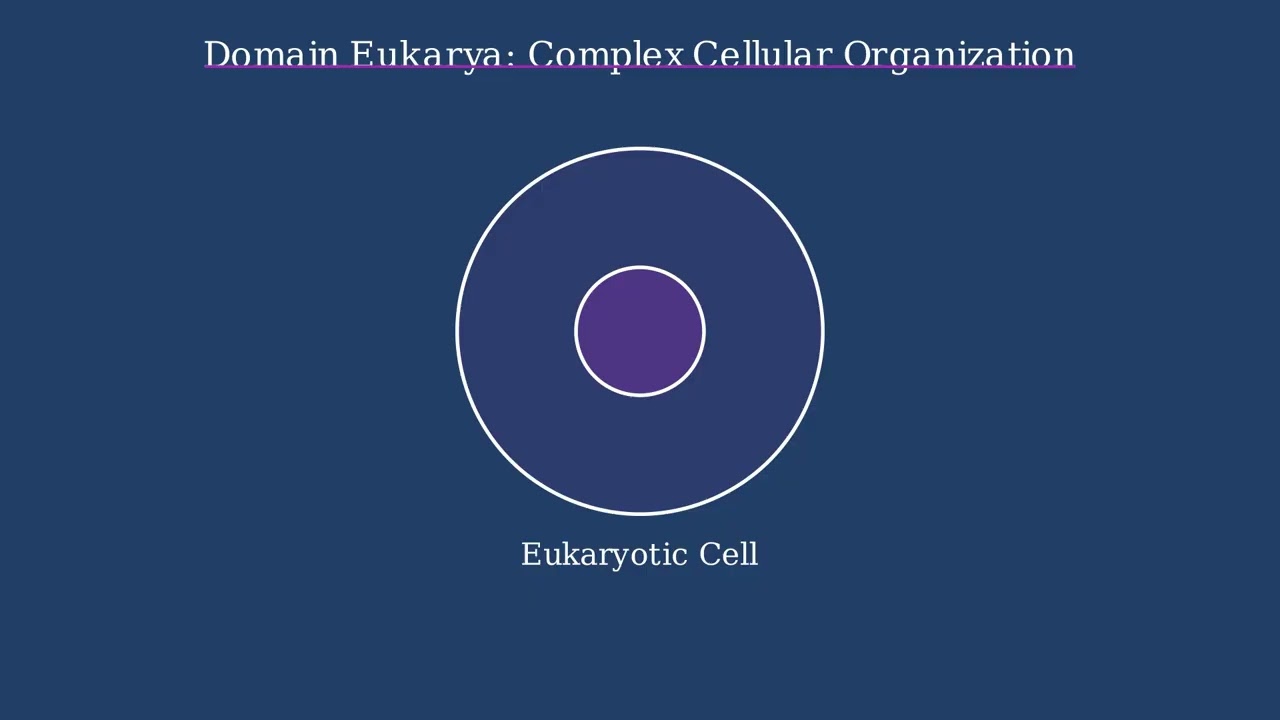