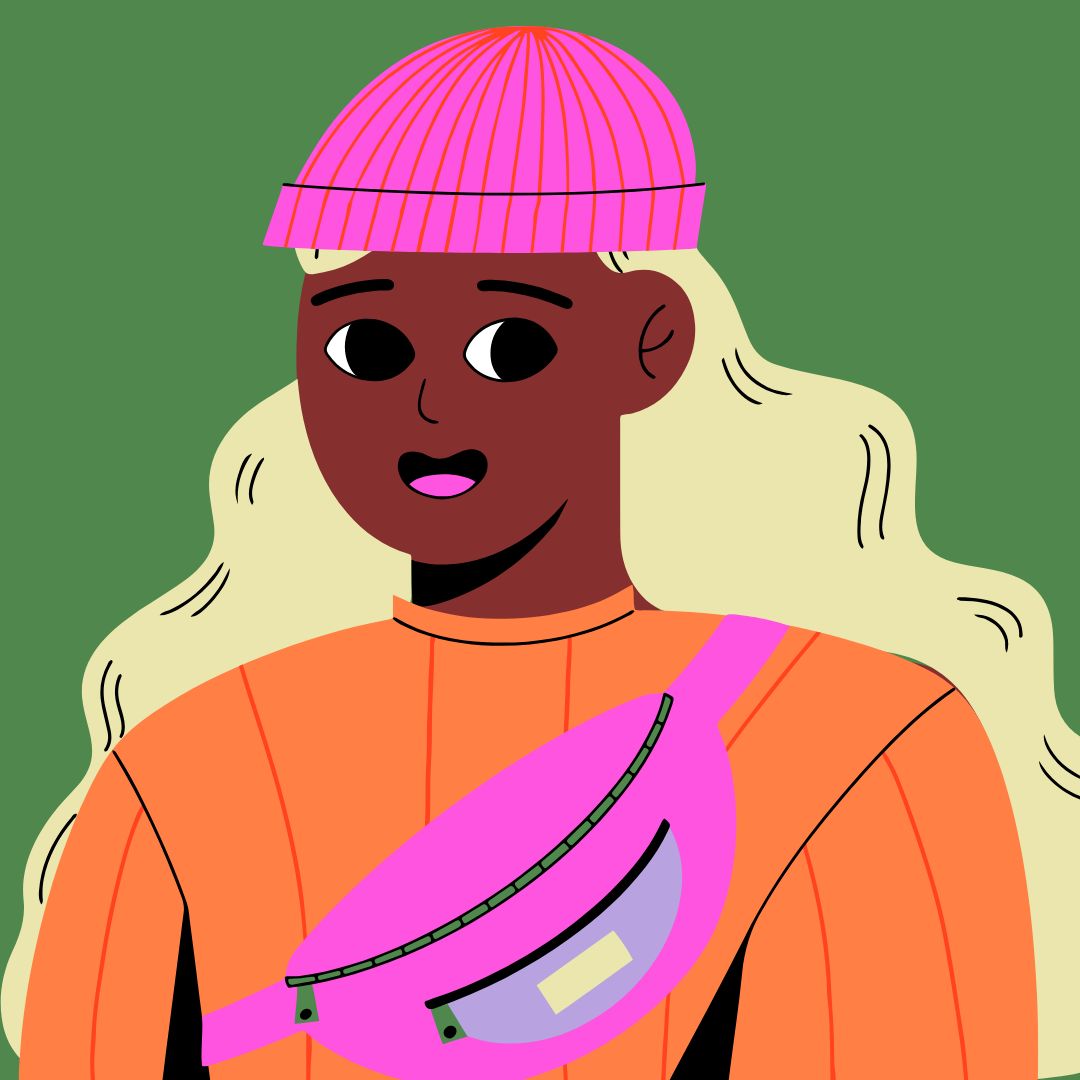
Sourav Pan
Transcript
Introduction to Endosymbiotic Theory, a revolutionary concept in cellular evolution.
The endosymbiotic theory proposes that certain eukaryotic organelles originated from free-living prokaryotic organisms that were engulfed by another cell.
Around one point five billion years ago, larger cells began to engulf free-living prokaryotes.
Instead of being digested, these prokaryotes survived inside the host cell.
Over time, a symbiotic relationship formed, with each organism providing benefits to the other.
These prokaryotes eventually evolved into the mitochondria and chloroplasts found in eukaryotic cells today.
This relationship was mutually beneficial. The host cell gained efficient energy production and new metabolic capabilities.
The prokaryotes received protection and a stable supply of nutrients.
The endosymbiotic theory revolutionized our understanding of cellular evolution. It explained the relatively sudden appearance of complex eukaryotic cells in the fossil record.
It provided a mechanism for evolutionary leaps and is strongly supported by molecular and genetic evidence.
This ancient endosymbiotic event led to the diverse and complex eukaryotic cells we see today in animals, plants, and fungi.
To summarize, the endosymbiotic theory states that mitochondria and chloroplasts were once free-living prokaryotes.
These prokaryotes were engulfed by host cells but survived to form a mutually beneficial relationship.
This process resulted in complex eukaryotic cells and transformed our understanding of evolution.
This groundbreaking theory continues to guide our understanding of cell evolution and the increasing complexity of life on Earth.
Lynn Margulis was an American evolutionary theorist, biologist, and science author who lived from 1938 to 2011.
She received her PhD from the University of California, Berkeley and later became a distinguished professor, first at Boston University and then at the University of Massachusetts Amherst.
Margulis began developing the Endosymbiotic Theory in the mid-1960s, proposing that certain organelles in eukaryotic cells originated as free-living bacteria.
Her theory was revolutionary and challenged the prevailing views of cellular evolution at the time.
In 1967, Margulis published her landmark paper ‘On the Origin of Mitosing Cells’ in the Journal of Theoretical Biology.
In this groundbreaking work, she argued that mitochondria evolved from aerobic bacteria, and chloroplasts from cyanobacteria, that were engulfed by host cells and formed permanent symbiotic relationships.
Her paper was initially rejected by fifteen different scientific journals, highlighting the resistance to her revolutionary ideas.
Despite the revolutionary nature of her ideas, Margulis faced significant skepticism from the scientific community throughout the 1970s.
But Margulis persisted, gathering evidence to support her theory, including similarities between organelle DNA and bacterial DNA, shared biochemical pathways, and structural resemblances.
By the 1980s, growing scientific evidence began to support Margulis’s theory, and with the advent of modern molecular techniques, her predictions were increasingly validated.
Today, the Endosymbiotic Theory is considered a cornerstone of our understanding of cell evolution. For her groundbreaking contributions, Margulis was awarded the National Medal of Science in 1999.
Lynn Margulis once said: ‘If you don’t know the right questions to ask, you’ll never come up with the right answers.’ Her willingness to question established paradigms revolutionized our understanding of cellular evolution.
After the first endosymbiotic event created mitochondria, a second major event occurred in the lineage leading to algae and plants.
The process began with an early eukaryotic cell that already contained mitochondria from the first endosymbiotic event.
A photosynthetic cyanobacterium lived independently in the same environment, capable of converting sunlight into energy.
Around 1.5 billion years ago, the eukaryotic cell engulfed the cyanobacterium, but instead of digesting it, established a symbiotic relationship.
Over hundreds of millions of years, this relationship evolved as the cyanobacterium gradually lost its independence.
The engulfed cyanobacterium gradually transformed into the chloroplast we see in modern plant cells. It retained its photosynthetic ability but lost many genes and functions.
Modern chloroplasts retain key features that reveal their bacterial origin. They have their own circular DNA, a double membrane structure, and still divide independently within the cell.
This second endosymbiotic event occurred only in the lineage leading to photosynthetic organisms. It gave rise to algae and plants, creating a major branch in the tree of life.
To summarize, the second endosymbiotic event occurred about one point five billion years ago. It followed the first endosymbiotic event that created mitochondria and happened only in the lineage that would become photosynthetic. This symbiotic relationship transformed a cyanobacterium into the chloroplast, enabling plants and algae to harness the power of photosynthesis.
The size and structure of organelles provide compelling evidence for the endosymbiotic theory.
Mitochondria and chloroplasts are remarkably similar in size to bacteria, typically ranging from one to two micrometers.
Unlike most cellular structures, mitochondria and chloroplasts possess a double membrane. The inner membrane closely resembles bacterial cell membranes in composition and structure.
Both mitochondria and chloroplasts divide through binary fission, a process identical to how bacteria reproduce. Unlike other cellular structures, these organelles replicate independently of cell division.
To summarize, the structural evidence supporting endosymbiotic theory includes the bacterial-like size of these organelles, their unique double membrane structure, their method of division by binary fission, and their internal organization that resembles their bacterial ancestors.
A compelling piece of evidence for the endosymbiotic theory comes from the DNA found in mitochondria and chloroplasts.
Both mitochondria and chloroplasts contain their own DNA, completely separate from the nuclear DNA of the cell.
The nuclear DNA exists as linear chromosomes.
In contrast, mitochondrial DNA is circular, resembling the chromosomes found in bacteria.
Similarly, chloroplast DNA is also circular in structure.
This circular structure is remarkably similar to the DNA found in free-living bacteria.
One of the most significant features of organelle DNA is that it replicates independently from the nuclear DNA of the cell.
Organelle DNA encodes some of the proteins needed for the function of mitochondria and chloroplasts.
However, over evolutionary time, many genes originally found in the organelles have been transferred to the nuclear genome.
The presence of DNA in these organelles, with its bacterial characteristics, provides strong evidence for the endosymbiotic origin of mitochondria and chloroplasts.
Ribosomal RNA sequences provide some of the strongest molecular evidence for the endosymbiotic theory.
Ribosomal RNA is an essential component of ribosomes in all living organisms. It’s highly conserved, meaning it changes very slowly over evolutionary time. This makes it an excellent molecular clock for tracing evolutionary relationships.
When scientists compared the ribosomal RNA sequences of mitochondria with various bacteria, they made a remarkable discovery.
Mitochondrial ribosomal RNA sequences share striking similarities with alpha-proteobacterial rRNA. The pattern of nucleotides shows clear homology, with matching regions that indicate a shared evolutionary history.
The sequence similarity between mitochondrial and alpha-proteobacterial rRNA is approximately 80 percent – far too high to be coincidental.
A similar pattern emerges when we examine chloroplasts and cyanobacteria.
Chloroplast ribosomal RNA sequences are remarkably similar to those found in cyanobacteria. The specific patterns of nucleotides show a level of homology that strongly suggests a common ancestry.
The similarity between chloroplast and cyanobacterial rRNA is even higher, at approximately 85 percent, providing compelling evidence for their shared evolutionary history.
When scientists construct phylogenetic trees based on ribosomal RNA sequences, the results are revealing. These evolutionary trees place mitochondria closest to alpha-proteobacteria and chloroplasts closest to cyanobacteria.
These close evolutionary relationships, revealed through ribosomal RNA analysis, provide molecular evidence that mitochondria and chloroplasts originated from free-living bacterial ancestors.
Could these molecular similarities be explained by convergent evolution rather than endosymbiosis?
While convergent evolution can explain functional similarities, the ribosomal RNA evidence goes far beyond this. The similarities include non-functional regions with no selective pressure, and the precise pattern of shared and unique mutations aligns perfectly with the proposed evolutionary timeline.
In summary, ribosomal RNA sequence analysis provides some of the strongest molecular evidence for the endosymbiotic origin of mitochondria and chloroplasts. The extensive similarities to their bacterial counterparts reflect their evolutionary origins as once free-living organisms that became integrated into eukaryotic cells.
Anaerobic eukaryotes live in environments with little to no oxygen, challenging our understanding of mitochondrial evolution.
Typical mitochondria perform aerobic respiration, using oxygen to efficiently produce ATP.
Many eukaryotes live in environments without oxygen, such as deep sea sediments, animal digestive tracts, and waterlogged soils.
In anaerobic environments, eukaryotes evolved modified mitochondria. Standard mitochondria transform into specialized organelles with different functions.
Hydrogenosomes are found in diverse organisms like trichomonads, fungi, and ciliates. They lack an electron transport chain but produce ATP through substrate-level phosphorylation, generating hydrogen as a byproduct.
Mitosomes represent the most reduced form of mitochondria. Found in parasites like Giardia and Entamoeba, they’ve lost the ability to produce ATP but retain crucial functions like iron-sulfur cluster assembly.
Despite their adaptations, both hydrogenosomes and mitosomes still show clear evidence of their endosymbiotic bacterial origin.
Many hydrogenosomes retain the characteristic double membrane structure of mitochondria, reflecting their endosymbiotic past.
Both organelles maintain protein import machinery similar to conventional mitochondria, using mechanisms derived from the original bacterial ancestor.
Some hydrogenosomes even retain small remnants of DNA, providing direct genetic evidence of their bacterial origin.
The existence of these modified mitochondria across diverse anaerobic lineages supports the theory that all eukaryotes share a common ancestor that already contained mitochondria.
These findings have profound implications. All eukaryotes, including anaerobes, evolved from aerobic ancestors with mitochondria. There’s no evidence for eukaryotes that never had mitochondria.
These anaerobic adaptations are secondary modifications of an originally aerobic endosymbiont, supporting the universality of mitochondrial endosymbiosis in the evolution of all eukaryotic cells.
During the evolution of eukaryotic cells, a remarkable process occurred where genes from endosymbiotic organelles were transferred to the host cell nucleus.
This process, known as Endosymbiotic Gene Transfer or EGT, was crucial for the integration of the endosymbiont and host genomes.
Over evolutionary time, DNA fragments from mitochondria and chloroplasts escaped their organelles and became incorporated into the nuclear genome.
Several mechanisms likely contributed to gene transfer. Direct DNA transfer occurs when organelle DNA fragments are released during breakdown and enter the nucleus.
RNA intermediates can also play a role, where organelle mRNA is reverse transcribed and then integrated into nuclear DNA.
In some cases, bulk DNA transfer occurs, where large segments of organelle genome move to the nucleus in single events.
As genes moved to the nucleus, a crucial innovation was needed: targeting sequences. These short peptide segments at the beginning of proteins act as address labels.
These targeting sequences direct proteins back to their appropriate organelles after being synthesized by ribosomes in the cytoplasm.
The extent of gene transfer is remarkable. In humans, approximately fifteen hundred genes of mitochondrial origin now reside in the nucleus.
In plants, around forty-five hundred genes of chloroplast origin have been transferred to the nuclear genome.
Meanwhile, human mitochondria retain only thirty-seven genes, a tiny fraction of their bacterial ancestor’s genome.
Gene transfer provided several evolutionary advantages. Nuclear DNA is better protected from the reactive oxygen species produced in mitochondria.
The nucleus offers more sophisticated gene regulation mechanisms, allowing for better control of gene expression.
Nuclear genes can undergo sexual recombination, increasing genetic diversity and adaptive potential.
Transfer decreases the risk of harmful mutations in organelles, where DNA repair mechanisms are more limited.
Finally, centralized genetic control in the nucleus allows for coordinated regulation of cellular functions.
The transfer of genes from organelles to the nucleus was a critical step in the integration of endosymbiont and host genomes.
This genomic integration transformed what began as separate organisms into a single, unified entity with shared genetic interests.
It represents one of the most profound examples of genetic integration in the history of life, fundamentally reshaping the eukaryotic cell.
Despite extensive gene transfer to the nucleus over evolutionary time, mitochondria and chloroplasts have retained some of their own genetic material.
This raises an important question: If most organelle genes have been transferred to the nucleus, why do any genes remain in these organelles?
The most widely accepted explanation is the redox regulation hypothesis.
This hypothesis suggests that some genes must remain within the organelle to allow rapid, local regulation of electron transport processes.
Both mitochondria and chloroplasts contain electron transport chains – complex protein systems that manage the flow of electrons and energy production.
Keeping these genes in the organelle allows for rapid local regulation in response to changing redox conditions.
This direct connection allows organelle DNA to respond instantly to the electron transport status, quickly adjusting protein production to maintain balance.
Maintaining redox balance is crucial for cellular health. If redox reactions become imbalanced, damaging reactive oxygen species can form.
These imbalances can lead to oxidative damage, causing protein dysfunction, DNA mutations, lipid peroxidation, and even cell death.
In summary, the redox regulation hypothesis explains that organelles retain specific genes to maintain rapid, local control over electron transport processes.
This local control is crucial for preventing potentially dangerous redox imbalances and maintaining efficient cellular energy production.
The Hydrogen Hypothesis provides an alternative explanation for the origin of mitochondria through endosymbiosis.
Proposed by William Martin and Miklós Müller in 1998, this theory suggests that the initial endosymbiotic relationship was based on hydrogen exchange between two distinct organisms.
The metabolic foundation of this hypothesis centers on the hydrogen dependency of the archaeal host.
Early archaea required hydrogen for their metabolism, while certain bacteria produced hydrogen as a waste product from fermentation.
This created a perfect syntrophic relationship. The archaeon, likely a methanogen, consumed hydrogen produced by the fermenting bacterium.
This metabolic relationship gradually evolved from external hydrogen exchange to full endosymbiosis through several steps.
First, the two organisms exchanged hydrogen externally. Then, they developed a closer physical association to improve the efficiency of this exchange.
Eventually, the archaeal host engulfed the bacterial partner, internalizing the hydrogen source. Finally, gene transfer occurred from the endosymbiont to the host.
The hydrogen hypothesis elegantly explains how the initial metabolic dependency led to a permanent cellular integration.
As this relationship became permanent, the engulfed bacterium evolved into what we now recognize as the mitochondrion.
The hydrogen hypothesis has several important implications for our understanding of early cellular evolution.
It suggests that eukaryotes originated in anaerobic environments and that mitochondria evolved from bacteria specifically involved in hydrogen metabolism.
Most importantly, it provides testable predictions that can be examined through comparative genomics and molecular biology.
The hydrogen hypothesis provides a compelling explanation for how metabolic dependencies could have driven the evolution of complex cellular structures through endosymbiosis.
Modern organisms exhibit various forms of endosymbiosis that provide insights into how organelles evolved.
The aphid-Buchnera relationship is one of the best-studied examples of obligate endosymbiosis. Aphids house Buchnera bacteria in specialized cells called bacteriocytes.
These bacteria synthesize essential amino acids that aphids cannot produce themselves. After millions of years of coevolution, Buchnera has lost 80% of its ancestral genome and cannot survive outside its host.
Coral polyps form symbiotic relationships with photosynthetic algae called zooxanthellae.
The algae provide photosynthetic products to their coral hosts, while the coral provides protection and nutrients. This mutualistic relationship can break down under stress, resulting in coral bleaching.
Paramecium can harbor bacterial endosymbionts such as Caedibacter, known as ‘killer bacteria.’
These bacteria produce toxic particles that kill sensitive Paramecium strains, giving the host a competitive advantage. The bacteria cannot complete their life cycle outside the host and are transmitted to offspring.
Paulinella chromatophora represents a recent endosymbiotic event that occurred about 60 million years ago.
This amoeba acquired photosynthetic organelles called chromatophores from cyanobacteria. Unlike other algae whose plastids evolved over a billion years ago, Paulinella’s endosymbiotic event is much more recent, providing a glimpse into early stages of organelle evolution.
These modern examples of endosymbiosis provide valuable insights into how ancient symbiotic relationships led to the evolution of organelles.
We can view endosymbiosis as a continuum from free-living organisms to fully integrated organelles. Modern examples represent different points along this evolutionary pathway.
By studying these modern relationships, scientists can better understand the processes that shaped the complex cells found in eukaryotes today.
These living examples serve as windows into the past, illuminating the evolutionary processes that led to the diverse cellular structures we see today.
Despite strong evidence supporting the endosymbiotic theory, it has faced several challenges throughout its history.
When Lynn Margulis first championed this theory in the 1960s, it was largely dismissed by the scientific community. Critics pointed to the lack of observable endosymbiotic events and insufficient fossil evidence.
Today, scientists still debate specific aspects of the theory. Some mitochondrial genes don’t match expected bacterial patterns. Chloroplast photosynthetic mechanisms show unexpected variations. And the timing of nuclear development remains contested.
Several alternative explanations have been proposed. The Direct Filiation Theory suggests organelles evolved from membrane invaginations rather than from free-living organisms. The Autogenous Model proposes organelles developed from the host cell’s own genome. Some researchers have even suggested viral integration as a source for organelle development.
The scientific community responded to these challenges with additional research. Expanded genomic sequencing confirmed endosymbiotic signatures in organelle DNA. Comparative studies using advanced techniques revealed phylogenetic relationships that further supported endosymbiosis. Laboratory models demonstrated the mechanisms of gene transfer and integration that explained anomalies in the data.
Ultimately, these challenges have strengthened rather than weakened the endosymbiotic theory. As scientists addressed each criticism with more precise research, the theory evolved to include more detailed mechanisms. It now integrates with other evolutionary theories and has gained broader scientific acceptance through the incorporation of genomic evidence.
By responding to challenges with rigorous research, scientists have transformed the endosymbiotic theory into a more robust explanation for the origin of eukaryotic cells.
Endosymbiosis has transformed our understanding of evolutionary relationships.
Traditionally, the tree of life was visualized as a simple branching structure with three main domains.
In this model, species evolved in a linear, hierarchical pattern from a universal common ancestor.
However, endosymbiotic events and horizontal gene transfer complicate this neat branching pattern.
Mitochondria originated from bacteria that were engulfed by an archaeal host. Similarly, chloroplasts came from cyanobacteria.
In addition, horizontal gene transfer allows genetic material to move between distantly related organisms, outside of parent-to-offspring inheritance.
These processes mean that instead of a simple tree, life’s evolutionary history is better represented as a complex web or network.
This is especially evident at the base of the eukaryotic lineage, where multiple endosymbiotic events and gene transfers shaped early cell evolution.
The modern eukaryotic cell represents a chimera of genes and organelles from multiple lineages – with an archaeal host, bacterial-derived mitochondria, and in plants, cyanobacterial-derived chloroplasts.
The web of life concept better captures these complex evolutionary relationships, challenging the traditional tree-like model especially at critical evolutionary transitions.
The endosymbiotic theory has become a cornerstone of modern biology.
Over decades, this theory has profoundly influenced evolutionary biology, genomics, and ecological studies.
It represents one of the most significant paradigm shifts in our understanding of how complex life evolved.
Despite its widespread acceptance, several important questions remain active areas of research.
The endosymbiotic theory demonstrates the extraordinary power of symbiotic relationships in driving major evolutionary innovations.
Future research will continue to explore new endosymbiotic events, examine understudied organisms, and investigate the molecular mechanisms that allow host and symbiont to integrate.
The endosymbiotic theory remains one of biology’s most profound insights into how complex life evolved through cooperation rather than just competition.
Study Materials
No study materials available for this video.
Helpful: 0%
Related Videos
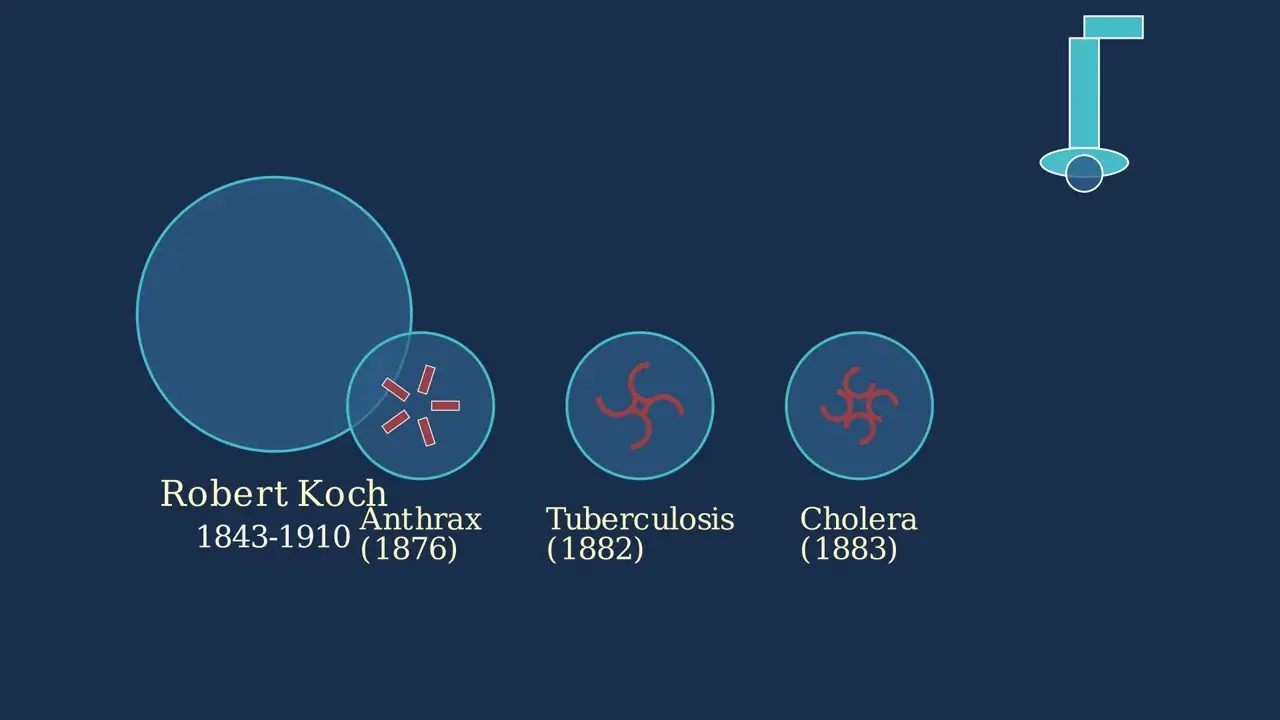
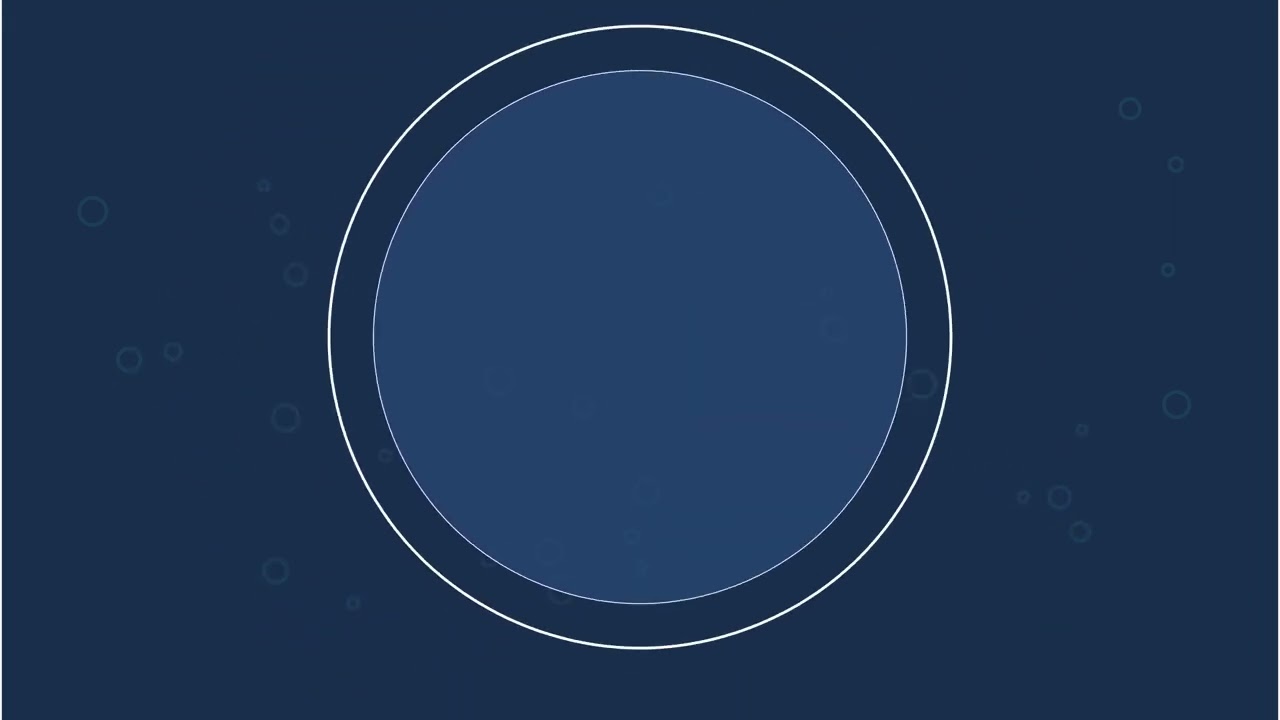
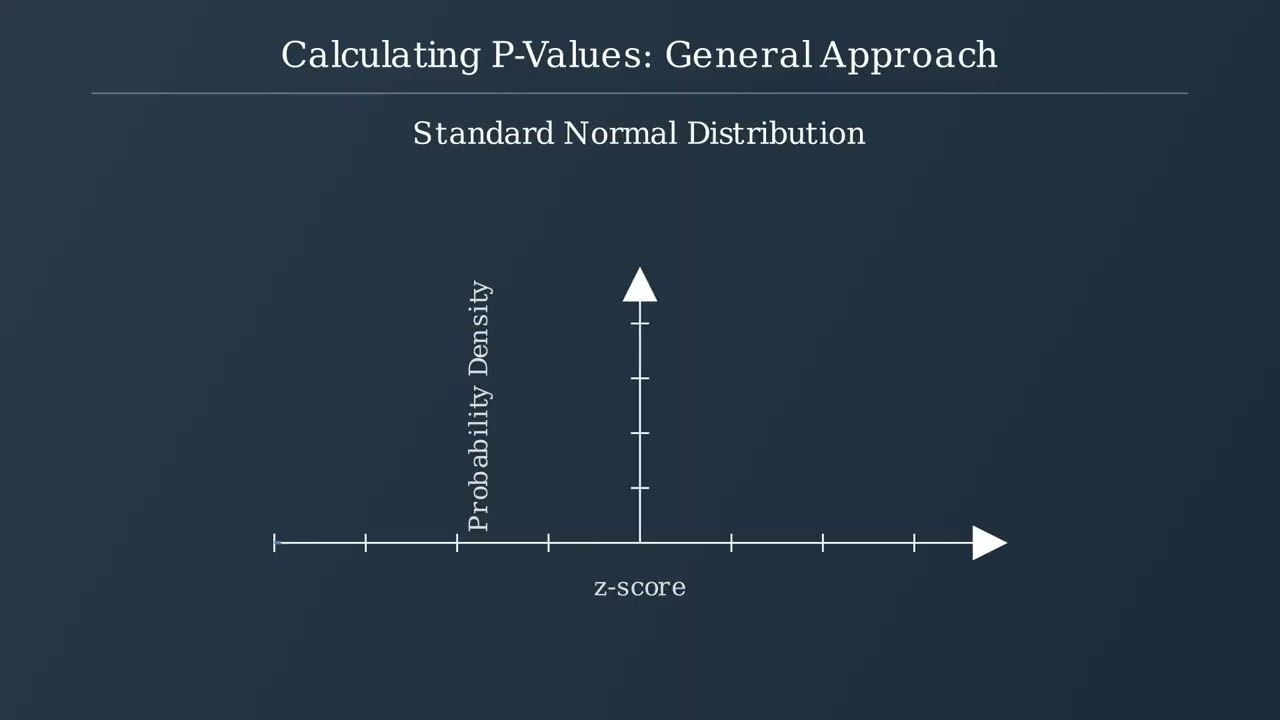
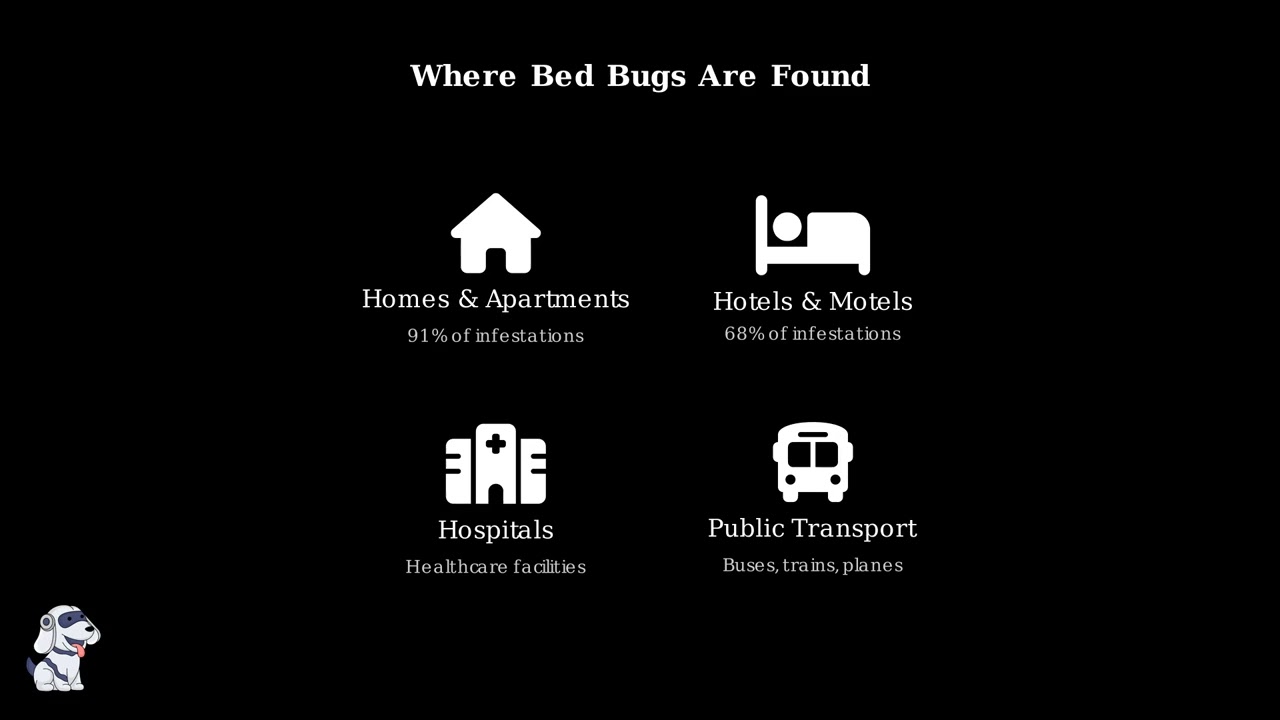
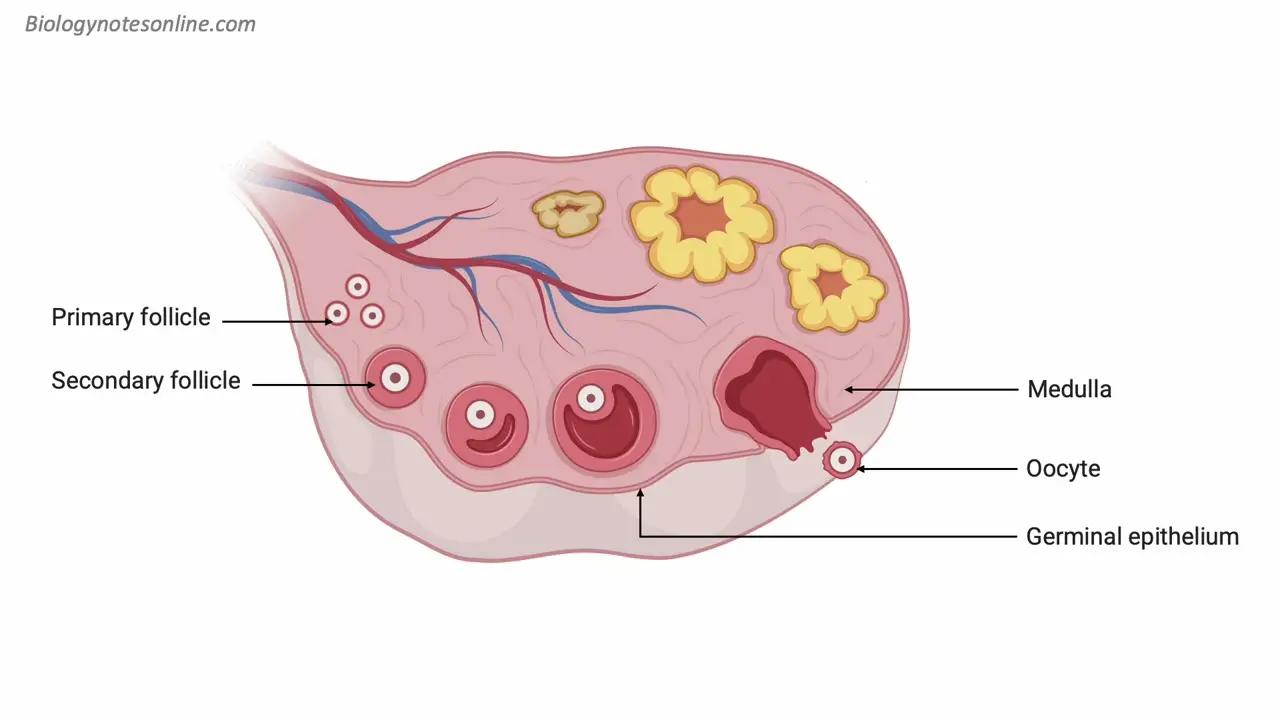
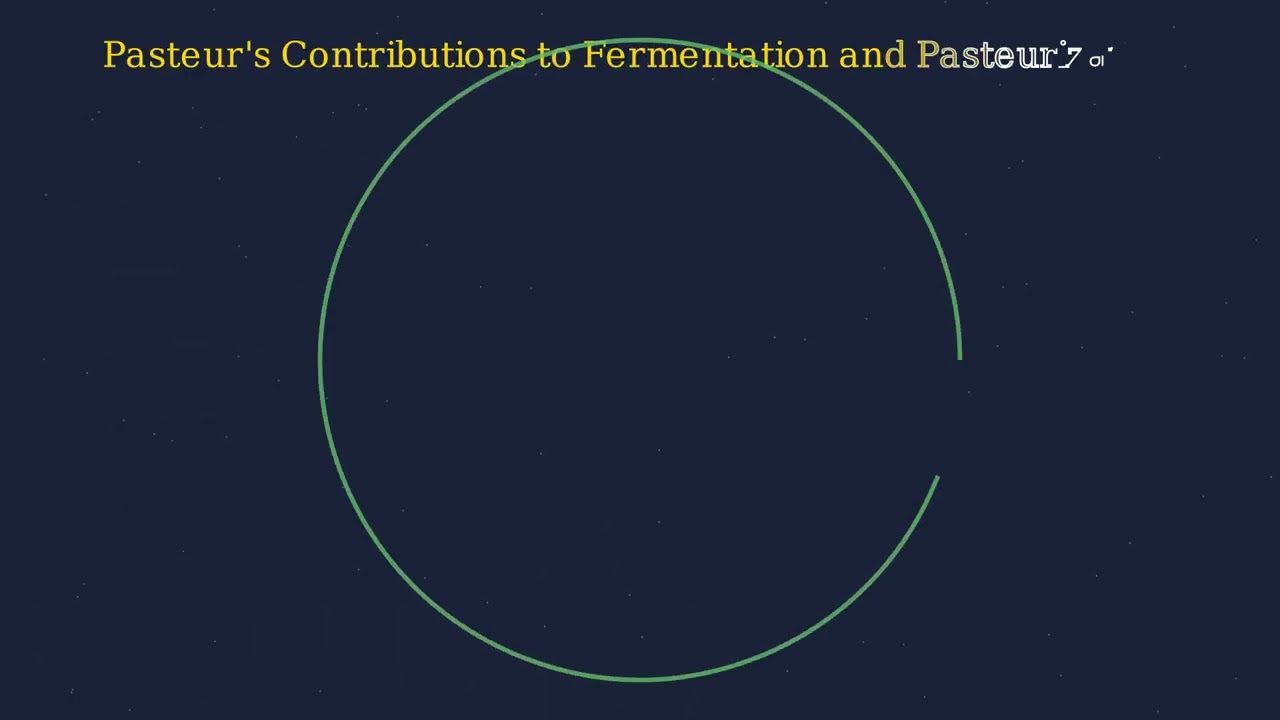
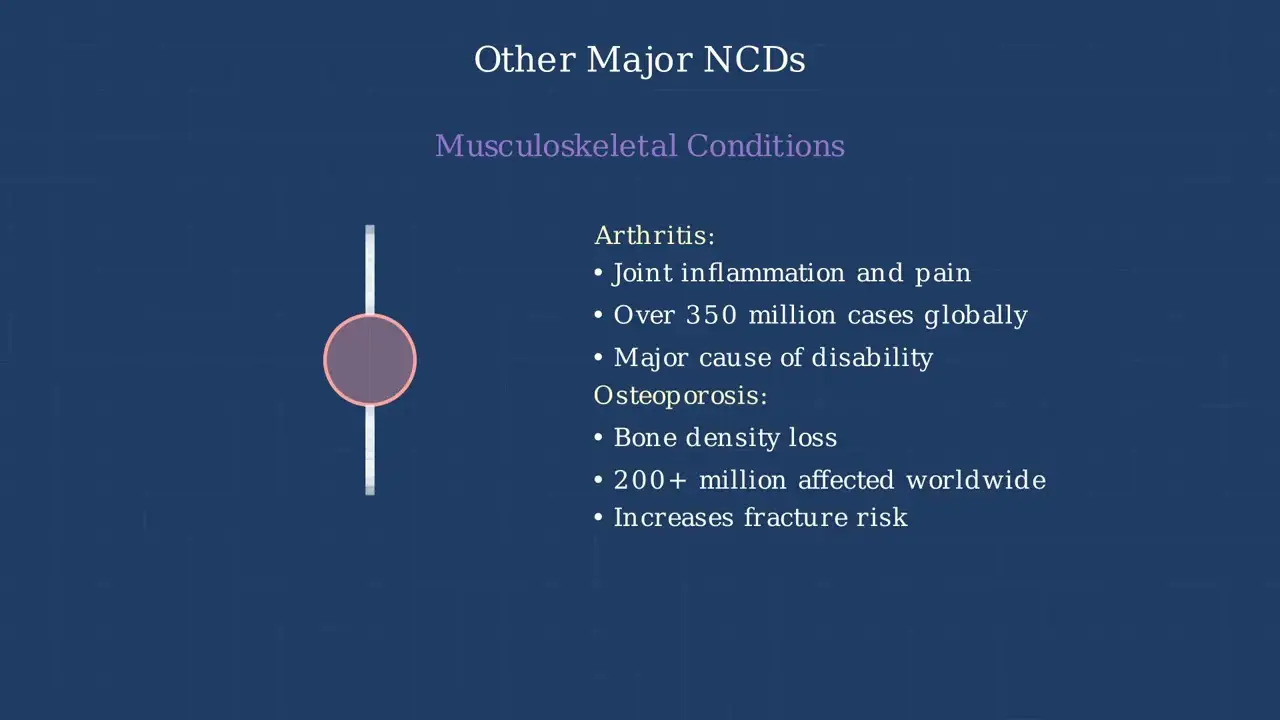
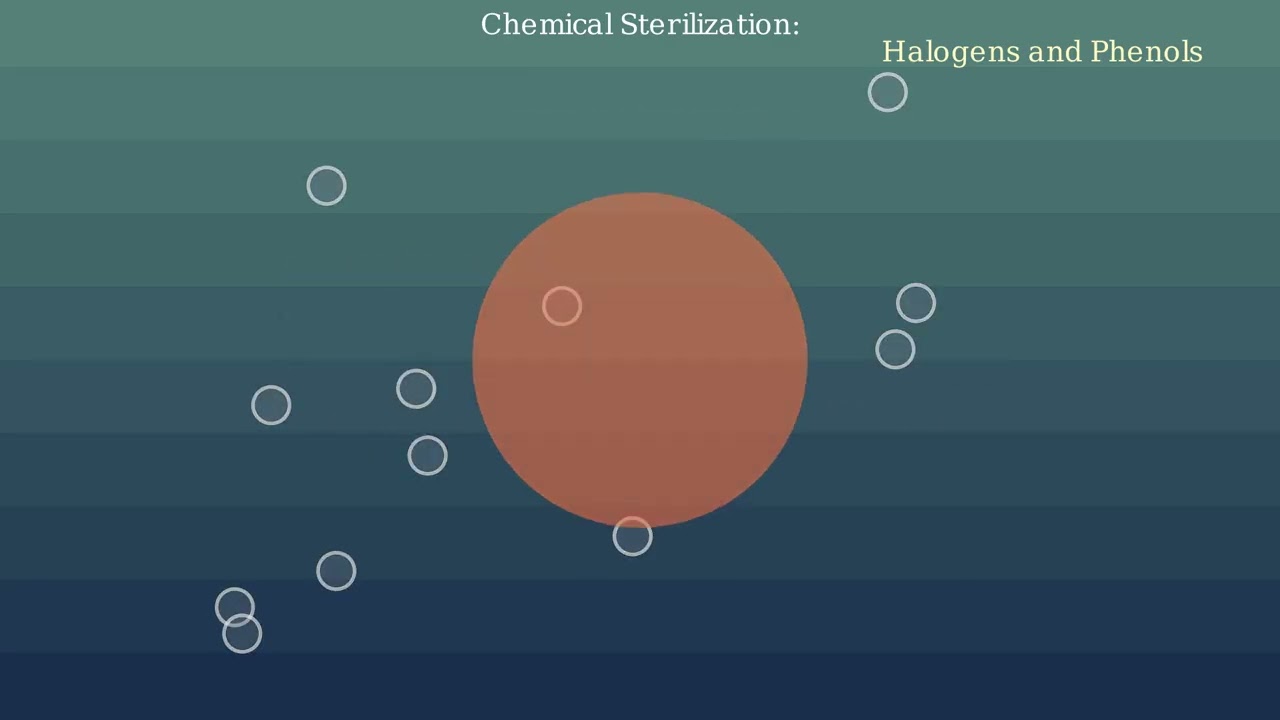
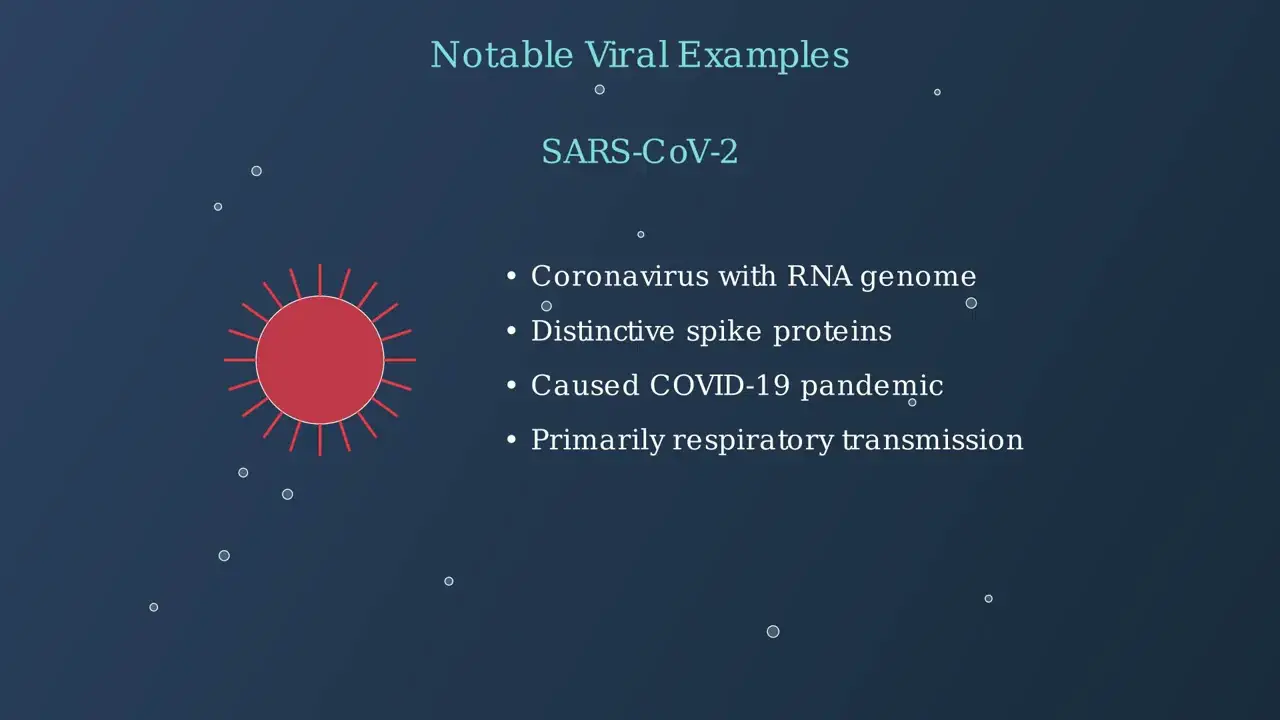
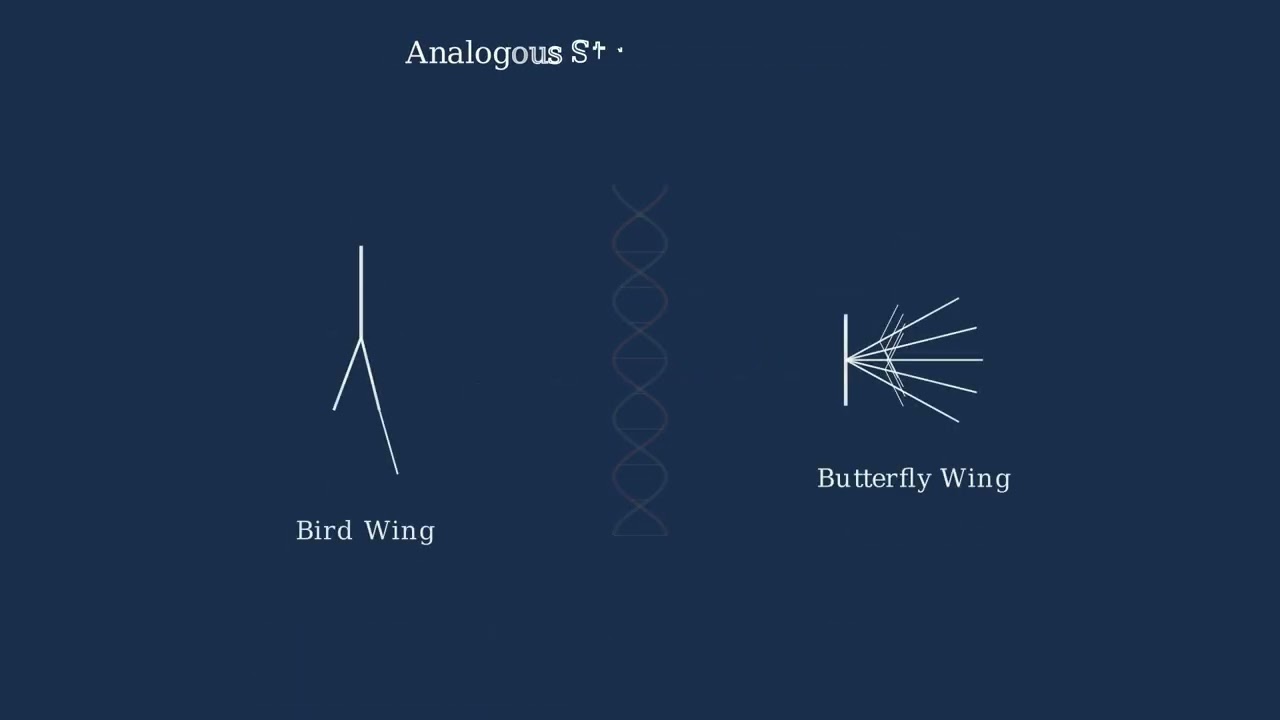